Introduction
Global health is rapidly being challenged by an aging population and epidemics of burden of lifestyle diseases that accumulate with age such as type-2 diabetes, obesity, non-alcoholic fatty liver, arteriosclerosis, depression, neurodegenerative diseases, hypertension, congestive heart failure (CHF), chronic kidney disease (CKD), cancer, chronic pulmonary disease, stroke and osteoporosis [Reference Stenvinkel, Meyer, Block, Chertow and Shiels1]. This rapidly growing group of chronic diseases is characterised by a low-grade chronic inflammation [Reference Fougère, Boulanger, Nourhashémi, Guyonnet and Cesari2], termed inflammageing [Reference Franceschi, Garagnani, Parini, Giuliani and Santoro3], mitochondrial dysfunction [Reference Stenvinkel4] and oxidative stress with increased generation of reactive oxygen species (ROS) [Reference Checa and Aran5] that accompanies the aging process (Fig. 1). These features are, in part, reflected by the repressed activity of a master regulator of hundreds of cytoprotective genes. This is the transcription factor “nuclear factor erythroid 2-related factor 2” (Nrf2), and its inhibitor kelch-like ECH-associated protein 1 (Keap1), which protects against inflammation and oxidative stress when upregulated [Reference Juul-Nielsen, Shen, Stenvinkel and Scholze6]. Age-related chronic disease is also commonly characterised by a loss of gut microbiota biodiversity [Reference Shiels, Buchanan, Selman and Stenvinkel7]. The emerging epidemic of burden of lifestyle diseases in modern society is partly due to genetic, epigenetic and functional adaptations that have taken place during evolution, as a result of changes in human development in response to changes in climate, access to food and pandemics. Thus, to handle the epidemics of burden of lifestyle diseases, we need to understand the history of our planet and the changes that occurred during evolution [Reference Vasseur and Quintana-Murci8]. Notably, we need a better understanding of how our health is influenced by our exposome across the human life course. The exposome comprises the totality of human environmental (both biotic and abiotic) exposures from conception to death [Reference Wild9]. The importance of the human exposome is emphasised by the finding that three simple exposome factors, namely air pollution, tobacco smoke and diet, account for ∼50% of mortality globally [Reference Lim, Vos and Flaxman10]. How such factors interplay, either cumulatively, independently or synergistically, with human genomes and epigenomes is poorly understood, especially in the context of antagonistic pleiotropy and psychosocial biology [Reference Simmons, Chaudhry and Graham11]. Thus, while the ongoing environmental crisis will undoubtedly have a significant impact on human health spans, identifying and mitigating the adverse effects of exposome dynamics at different life stages may take generations.
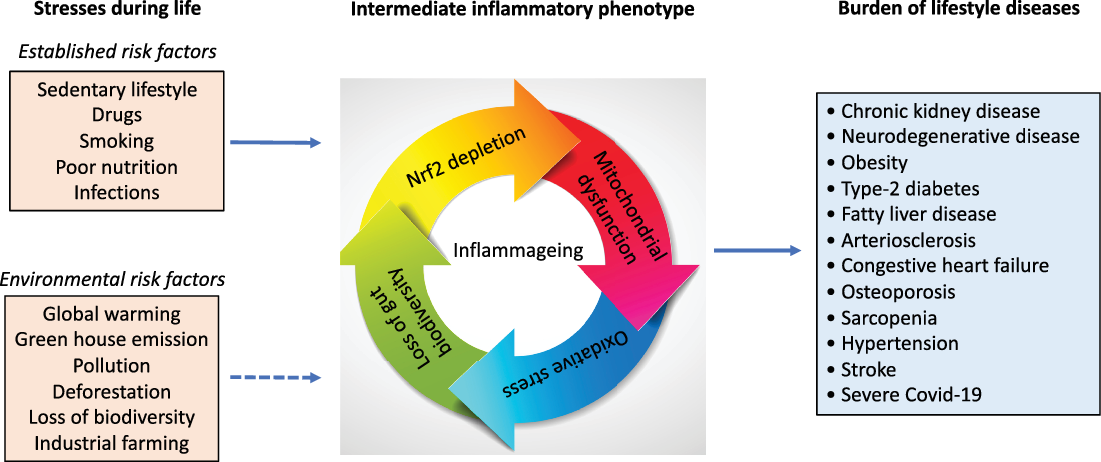
Fig. 1. Numerous life stressors activate the inflammatory intermediate phenotype associated with an imbalanced microbiota, oxidative stress and mitochondrial dysfunction, which drive the risk of burden of lifestyle diseases that accumulate with age. Besides established risk factors, emerging evidence suggests factors that are related to our external environment, such as global warming, deforestation, pollution also increase the risk of burden of lifestyle diseases and contribute to inflammageing.
About 3.5 billion years ago (BYA), life on the planet was created from the sea [Reference Ohno12]. A vital challenge, key to the success of the history of aerobic land life on our planet, is how cellular processes co-adapted to overcome the metabolic toxicity that results from the presence of ROS. The “Great Oxygenation Event” about 2.4 BYA, is recognised as the most geologically critical environmental change since it submersed the planet in an oxidising atmosphere, which set the stage for the evolutionary transition to the aerobe-dominated biota that still exists [Reference Gacesa, Dunlap, Barlow, Laskowski and Long13]. As a waste product of the planets most successful group of microorganisms (photosynthetic cyanobacteria) excess oxygen was released that induced the production of ROS, that benefit the immune defence and cellular structure synthesis. However, as a double-edged sword, elevated levels of ROS may also lead to cell damage and it has been proposed that ROS acted as a primary driver of evolution since it causes mutations in the genome and induces irreversible oxidative modification of lipids, glycans and proteins [Reference Checa and Aran5]. Orthologues of Nrf2 first appeared in fungi; thus, it is believed that Nrf2 evolved as a protection when organisms were exposed to oxygen and ROS [Reference Fuse and Kobayashi14]. Understanding the evolution of Nrf2 as an effective antioxidant response to various stressors in plants, animals and humans on the planet, has major implications for the ongoing epidemic of burden of lifestyle diseases associated with inflammageing and reduced mitochondrial biogenesis (Fig. 2). In this review, we discuss examples of animals that may provide novel solutions for prevention and/or treatment of burden of lifestyle diseases (Fig. 3).
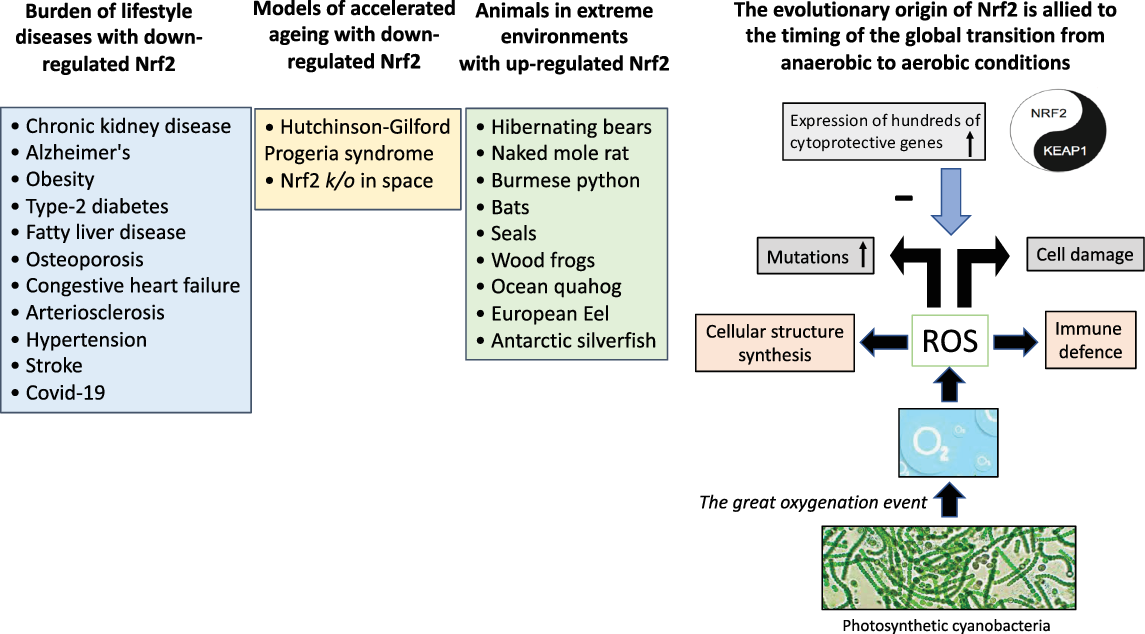
Fig. 2. The “Great Oxygenation Event” set the stage for the evolutionary transition to the aerobe-dominated biota that still continues and the evolutionary origin of Nrf2. When living organisms were exposed to oxygen (released from photosynthetic cyanobacteria), a need emerged for protection against cell-damaging reactive oxygen species. The master switch and transcription factor Nrf2 emerged for cytoprotective activation with an inhibitor Keap1 that fine-tuned the activity of Nrf2. Based on studies in animal species, it can be hypothesised that superior anti-oxidant defence mechanisms with enhanced Nrf2 expression are protective and facilitate survival in extreme environments. Experiences from rare progeric diseases, such as Hutchinson-Gilford Progeria syndrome and Nrf2 k/o mice stressed by space travel, imply a protective role of Nrf2 in aging. Given the association between depressed expression of Nrf2 and chronic burden of lifestyle diseases associated with inflammation and oxidative stress that accumulate with aging, nutrients targeting Nrf2, using “Food as Medicine“ [Reference Mafra, Borges, Lindholm, Shiels, Evenepoel and Stenvinkel127], may have a positive effect on a cluster of burden of lifestyle diseases and planetary health. ROS, reactive oxygen species.

Fig. 3. Selected examples of species that have provided clues for novel targets for “burden of life style” diseases, aging, alcoholism, COVID-19 and antibiotic resistance. CHF, congestive heart failure; DM, diabetes mellitus.
Transformation of Planets Natural Systems: We Face a Different Disease Panorama
The accelerating disturbances in the planet’s external environment will perturb interactions between a disturbed internal and external milieu, effectively increasing the chronic inflammatory burden of lifestyle diseases (i.e., a disturbed “internal environment”). It is critical that we understand these relations in order to reduce the burden of illness. Studying these complex interactions requires a syndemic approach that integrates studies of human health and animal health, with changes in our external environment; that is, “Planetary health” [Reference Acunzo, Escher and Ottersen15] defined as “the health of human civilisation and the state of the natural systems on which it depends.” It is likely that a disturbed planetary health will have a more severe impact on susceptible individuals such as children, the elderly and patients with chronic debilitating disorders. Thus, we need to understand the human health implications of the rapid disruption and transformation of our planet’s natural resources that we now witness. As an example, elevated atmospheric CO2 concentration may result in dietary deficiencies [Reference Myers, Zanobetti and Kloog16]. Moreover, WHO has reported that 23% of all global deaths relate to the environment and there is evidence of how disturbances in the global environment affect global health due to disruptions of the climate system, scarcity of clean water, loss of biodiversity, land degradation, deforestation, etc. Here are some examples. In hot parts of the world, such as Central America and Sri Lanka, an epidemic of CKD (Mesoamerican nephropathy) is an emerging health concern amongst poor agricultural workers [Reference Sörensen and Garcia-Trabanino17]. Moreover, global warming reduces gut microbiota diversity [Reference Bestion, Jacob and Zinger18] and a recent study of pregnant women suggests that rising temperature is associated with perturbations in maternal heat homeostasis that increase the risk of pre-eclampsia [Reference Shashar, Kloog and Erez19]. Concerns have also been raised that global warming increases the risk of tissue hypoxia [Reference Wei, Cao and Fu20]. Moreover, since air pollution alters the composition of the gut microbiota in mice [Reference Guo, Zhuang and Huang21], it may not only promote respiratory disease, but also other diseases that accumulate with age. Indeed, epidemiological studies have shown links between long-term exposure of fine particulate matter (PM2.5) and the risk of CKD [Reference Li, Huang and Wang22], obesity and type-2 diabetes [Reference Bailey, Naik, Wild, Patterson and Alderete23] and cardiovascular disease [Reference Al-Kindi, Brook, Biswal and Rajagopalan24]. The relationship between scarcity of clean water and global health, especially pertinent to gastrointestinal infections, has been evident for centuries. Lack of clean water and global warming increases the risk of chronic dehydration; a condition that may increase the risk of type-2 diabetes [Reference Roussel, Fezeu and Bouby25]. It has been estimated that the risk of obesity will increase by 12% from 1961 to 2081 due to global warming alone [Reference Kanazawa26]. Although activation of the fructose vasopressin pathway [Reference García-Arroyo, Muñoz-Jiménez and Gonzaga27] may play a major part in this, global warming will also lead to a more sedentary lifestyle and increased intake of high-calorie beverages, established risk factors for burden of lifestyle diseases. Finally, as positive associations between pollutants and obesity have been documented, it has been hypothesised that exposure to plastics, organic pollutants, heavy metals and pesticides causes obesity via damage to our natural weight control mechanisms [Reference Wang, Hollis-Hansen, Ren, Qiu and Qu28].
Inspiration from Nature Provide Innovative Ways to Improve Human Health
Due to the current environmental crisis, the human race is being challenged like never before. Thus, we must urgently find new and innovative solutions for this most challenging global problem, before it becomes humanity’s nemesis. Nature is the oldest model, measure and mentor we could ask for in our search for solutions to emerging human problems. The vast diversity of about 8.7 million species is one of the most striking aspects of life on our planet. Since the emergence of wildlife about 650 MA, animals have survived (or not) based on whether their adaptations to environmental change and the previous five mass extinctions (including global warming due to greenhouse gas emissions during the Permian–Triassic mass extinction) have been appropriate or not. Species that did not evolve and adapt to a changing environment became extinct [Reference Jurikova, Gutjahr and Wallmann29]. Because humans have consistently overexploited and destroyed ecosystems and animal habitats, it will take millions of years for the planet to recover from the loss of animal diversity that is expected to occur over the next 50 years [Reference Davis, Faurby and Svenning30]. This will have a catastrophic effect, as biodiversity not only includes the quantity of currently existing species, but also the sum of the unique evolutionary developments that have taken place over geological epochs, for each individual species [Reference Davis, Faurby and Svenning30]. If Homo sapiens lives up to the name we have christened ourselves (i.e., the “wise man”), we should instead of destroying and exploiting the natural environmental balance, learn from the ingenious evolutionary adaptations and imitate them (i.e., apply a biomimetic approach) to solve prevailing human issues [Reference Stenvinkel, Painer, Johnson and Natterson-Horowitz31]. Although innovation-based opportunities, based on solutions in nature, have proven to be successful in a wide range of areas, such as technology, chemistry and architecture, biomedical science has not yet developed its full potential in this context [Reference Snell-Rood32]. The opportunities in promoting human health are staggering and biomimetic studies have already identified potential applications, such as the antibiotic and sunscreen activities of red sweat from the hippopotamus [Reference Saikawa, Hashimoto and Nakata33], marine-inspired polymers for medical adhesives [Reference Balkenende, Winkler and Messersmith34], and inspired by micro-sized mosquitoes needles, a strategy to implant small flexible microprobes into the brain [Reference Shoffstall, Srinivasan and Willis35]. Current biological approaches focus largely on research using conventional laboratory model organisms, such as mice and rats, which take place in the unnatural laboratory environment. Recent reports have communicated doubts about the efficacy of laboratory studies in mice and rats seeking to find efficient treatment strategies for human disease. It has been argued that these models are metabolically morbid [Reference Martin, Ji, Maudsley and Mattson36] and that the majority of experimentation is not valid as a consequence [Reference Akhtar37]. A biomimetic approach, using evolutionary medicine as a powerful lens, could eliminate many of these problems since nature is never careless, nor cheats in its evolutionary experiments. The risk that biomimetic research leads to similar erroneous results is thus minimal [Reference Stenvinkel, Painer, Johnson and Natterson-Horowitz31]. Some limitations of biomimetics should be acknowledged. As most of the existing biomimetic examples is correlative, molecular genetics should be introduced to determine causation. Moreover, it is conceivable that not all physiological and anatomical elements in animals may be the result of natural selection.
Hibernation and Seasonal Variability Hold Clues for Human Health
There are many examples where humans can learn from wild animals, which through evolution have developed ingenious solutions for protecting themselves against chronic burden of lifestyle diseases. Hibernating bears, with a reduced metabolic state, do not develop insulin resistance or type-2 diabetes, despite pronounced obesity at the end of summer and in autumn [Reference Stenvinkel, Painer, Johnson and Natterson-Horowitz31]. A recent comparative study has shown that, in contrast to captive bears, free-ranging bears turn on a metabolic switch that shunts choline to generate the methyl donor betaine instead of the pro-atherogenic gut microbial metabolite trimethyl monoamine oxide (TMAO) [Reference Ebert, Painer and Bergman38]. Characterisation and understanding of how to turn on and off such a beneficial metabolic switch during stressful periods could hold clues for novel treatment options in many burden of lifestyle diseases. In addition, despite months of inactivity, starvation and impaired kidney function with anuria during hibernation, bears do not suffer from osteoporosis, muscle loss or atherosclerosis when they get back into an active lifestyle again in the spring [Reference Stenvinkel, Jani and Johnson39]. Elevated cholesterol efflux capacity and lower affinity of LDL cholesterol for arterial proteoglycans were recently found to constitute vasculoprotective properties in bear plasma lipoproteins [Reference Pedrelli, Parini and Kindberg40]. An upregulation of the transcription factor Nrf2 [Reference Chazarin, Ziemianin and Evans41] and a shift in metabolic profile with a slow-oxidative fibre and mitochondrial biogenesis [Reference Chang, Lazo, Appel, Gutiérrez and Grams42] has been shown to underlie the resistance to skeletal muscle atrophy in hibernating brown bears. High cortisol levels have been reported to be the key adaptation during hibernation, which is linked to reduced activation of AMPK/PGC-1α/PPAR-α in the regulation of metabolism in skeletal muscle and adipose tissue [Reference Vella, Nelson and Jansen43]. Finally, as the gut microbiota modulates energy metabolism in bears [Reference Sommer, Ståhlman and Ilkayeva44], accumulating evidence suggests that the gut microbiota plays a protective role during the vulnerable hibernation period. In contrast to the superior mechanisms that have developed during evolution to protect hibernating bears from the dramatic physiological transitions that occur between periods of high and low metabolic activity, other seasonal mammals, such as lemurs, are at higher risk, when the number of seasonal transitions increased in frequency [Reference Landes, Perret, Hardy, Camarda, Henry and Pavard45]. Such studies may be of relevance for climate-induced seasonal shift and increase reasons for seasonal variation in mortality observed in burden of lifestyle diseases, such as CKD [Reference Goto, Hamano, Ogata and Masakane46] and stroke [Reference Lanska and Hoffmann47].
Water Conservation Systems Have Evolved over the past 350 Million Years
The link between fat accumulation and water balance in those species that suffer from water shortages for long periods, such as camels and blue whales, gives us clues about metabolic survival mechanisms that have been put out of play in a modern society with a sedentary lifestyle and overconsumption of high-calorie foods [Reference Johnson, Stenvinkel and Andrews48]. Ingenious solutions that evolved to withstand extreme temperatures in the animal kingdom may provide hints for the treatment of human diseases in an era of global warming. One of the best examples of an ingenuous solution comes from a certain beetle species in the Namib Desert that have evolved a system to collect water from the fog on their backs by way of wettability patterns [Reference Chen and Zhang49]. The Arabian camel has developed an amazing capacity to cope with extreme heat stress and drought without any physiological impairment. Thus, camels could give us valuable indications on how to cope with global warming during the Anthropocene. Data suggest that heat shock proteins play a critical role in their tolerance to heat [Reference Hoter, Rizk and Naim50] while camel milk activates Nrf2 [Reference Du, Lv and Su51]. At the other extreme, Alaskan wood frogs can survive seasonal exposure to sub-zero temperatures and have developed amazing mechanisms to withstand interrupted blood flow and oxidative stress due to anoxia and dehydration. It was recently reported that wood frogs adapt to low-temperature stress via activation of Nrf2 and increased antioxidant defences [Reference Zang, Gupta and Stoery52]. In several recent large double-blind randomised trials, it has been demonstrated that substantial loss of glucose and sodium due to inhibition of sodium-glucose co-transporter 2 (SGLT2) has a major beneficial impact on the progression of kidney disease, major adverse cardiovascular events and hospitalisation due to heart failure and cardiovascular mortality [Reference Taylor, Yazdi and Beitelshees53]. Patients on SGLT2 inhibitor therapy adjust to the reduction in energy availability and conserve water. Estivation is an evolutionary process by which various animals, such as snails, crocodiles, hedgehogs, tortoises and lungfish, adapt to a state of dormancy during hot periods of the year to conserve energy and protect organs. As recently reviewed [Reference Marton, Kaneko and Kovalik54], SGLT2-inhibitors induce estivation-like metabolic patterns, which are likely to contribute to the observed improvements in cardiac and renal function. Thus, nature has already figured out a way for organ protection in estivating mammals, a metabolic switch that humans have learned to master in the 21st century.
Cancer Protective Solutions in Nature
Other wonders of nature that inspire solutions for human diseases include the amazing protection from cancer that has developed in elephants [Reference Abegglen, Caulin and Chan55]. Analysis of their genome has indicated that they have increased cellular apoptotic responses to DNA damage, potentially explained by the multiple copies of the p53 gene [Reference Abegglen, Caulin and Chan55], which triggers protein cell death when irreparable DNA damage is detected, which otherwise could make a cell cancerous. Humans with a mutation in TP53 develop Li–Fraumeni syndrome and have a nearly 100% lifetime risk for developing cancer [Reference Aedma and Kasi56]. Elephants also have 11 extra copies of the leukemia inhibitory factor (LIF) gene, which induces apoptosis in the absence of DNA damage, or activation by the p53 gene [Reference Vazquez, Sulak, Chigurupati and Lynch57]. Other mammals, such as naked mole rats, whales, grey squirrels, bats, cows and horses [Reference Seluanov, Gladyshev, Vijg and Gorbunova58] can provide additional clues for the human fight against cancer. As an example, the capybara, a gigantic rodent native to South America, has developed an expanded family of immune-related genes that involves T cell-mediated tumour suppression, and which enhances their immune surveillance against cancer [Reference Herrera-Álvarez, Karlsson, Ryder, Lindblad-Toh and Crawford59]. As the insulin signalling pathway allows capybara to grow large relative to other rodents, it is possible that their immune system has evolved differently compared to other rodents to compensate for the increased risk of cancer that comes with increased body size [Reference Herrera-Álvarez, Karlsson, Ryder, Lindblad-Toh and Crawford59]. In each of these species, evolution has taken a different path to decrease the risk of cancer, which could lead to novel complementary mechanisms for cancer resistance for the development of new human cancer therapies. This is particularly pertinent, as there is a clear link between an increase in environmental pollutants and cancer. Mammals with an exceptionally high risk of cancer, such as Tasmanian devils, ferrets and dogs, may also provide insights that benefit human health. Tasmanian devils provide a “natural” disease model for exploring immune evasion mechanisms in transmissible cancer [Reference Patchett, Tovar, Blackburn, Woods and Lyons60]. Since this cancer epidemic started in 1996, the Tasmanian devil has developed two independent lineages of allogeneic clonal transmissible facial tumour disease that has killed about 80% of the local population [Reference Murchison, Schulz-Trieglaff and Ning61]. As the disease recently shifted from an epidemic to an endemic phase, this implies that the Tasmanian devil has developed resistance against cancer. This was recently shown to be mediated via activation of RASL11A (code for small GTPases) [Reference Margres, Ruiz-Aravena and Hamede62] and the major histocompatibility complex (MHC-I) that was identified as a target for anti-tumour and allogeneic immunity [Reference Ong, Patchett and Darby63]. Moreover, as inhibition of cholesterol synthesis by atorvastatin shuts down Devil facial tumour disease energy metabolism and prevents tumour growth [Reference Ikonomopoulou, Lopez-Mancheño and Novelle64], this has provided understanding that could benefit the treatment of human cancer. Thus, elucidating the underlying mechanism of this transmissible tumour may give insights for cancer treatment. Additionally, as the phylodynamic analytical framework used to map the epidemiological dynamics of the Tasmanian devil can be applied to any pathogen, it can also guide intervention strategies in future pandemics [Reference Patton, L, awrance and Margres65].
Organ Growth and Protection against Heart Failure in the Animal Kingdom
Nature is replete with other examples of elegant solutions for protection from burden of lifestyle diseases that could serve as biomimetic inspiration for improving human health [Reference Stenvinkel, Painer, Johnson and Natterson-Horowitz31]. Vertebrates have a vast array of epithelial appendages including teeth, feathers, scales, spines and hair. Over 450 million years of evolution sharks, salamanders and lizards have developed unique mechanisms for appendage regeneration of amputated or injured tissues. As explorations of this process point to customary mechanisms, such as Wnt/β-catenin and fibroblast growth factor (FGF) signalling for the restoration of a functional appendage, these animals provide a useful guide for effective regenerative strategies in man [Reference Daponte, Tylzanowski and Forlino66]. The reason(s) why larger mammals, during their evolution, lost the capacity for appendage regeneration remains elusive and requires further study. Another elegant solution needing mention is the extreme organ growth, such as the 40% cardiac hypertrophy (with increased cardiac output for about 48–72 hrs) after Burmese pythons ingest large meals, such as a goat [Reference Andersen, Rourke, Caiozzo, Bennett and Hicks67]. As injection of a combination of fatty acids found in python plasma after large meals promotes physiological mammalian cardiomyocyte hypertrophy [Reference Riquelme, Magida and Harrison68], targeted fatty acid supplementation may be a novel strategy to modulate cardiac gene expression and function in CHF. As a consistent enrichment of the Nrf2-mediated oxidative stress responses was recently demonstrated in the Burmese python [Reference Andrew, Perry and Card69], this ubiquitous cytoprotective pathway may also be important in mediating cellular stress during extreme regenerative growth.
The superior protective mechanisms against the effects of high blood pressure (twice as high as in other mammals) that have developed in the giraffe may serve as an inspiration for the development of strategies to protect humans from hypertensive-related kidney disease and CHF with preserved ejection fraction (HFpEF) [Reference Damkjaer, Wang and Brøndum70]. One major difference in human hypertensive-induced left ventricular hypertrophy (LVH) and developmentally induced thick left ventricular wall in the giraffe is reduced cardiac fibrosis in the giraffe myocardia [Reference Østergaard, Baandrup and Wang71]. A recent study, which generated a high-quality giraffe genome, identified the giraffe FGFRL1 gene as an outlier compared to other ruminants [Reference Liu, Gao and Cui72]. When the giraffe mutation was inserted into the FGFRL1 gene in mice, significantly less renal and heart fibrosis was observed during hypertension [Reference Liu, Gao and Cui72]. Thus, as the giraffe FGFRL1 gene counteracts the detrimental effects of hypertension, it may hold a clue for treatments to protect humans from the adverse effects of hypertension.
Early vascular aging, which is highly prevalent in patients with burden of lifestyle diseases, contributes to premature cardiovascular disease. A recent study using the transparent extracorporeal vascular network of the colonial ascidian star tunicate (Botryllus schlosseri) show that age-related changes, such as vessel narrowing, reduced blood flow and less responsiveness to stimuli, resemble changes that occur in aging mammalian vessels [Reference Rodriguez, Taketa and Madhu73]. As newly regenerated vascular cells of this invertebrate maintain an aged phenotype, this suggests that heritable epigenetic vascular changes promote aging [Reference Rodriguez, Taketa and Madhu73]. Thus, the global nature and progression of aging in star tunicate make it a new and robust model of vascular progeria studies.
The World’s Most Unappreciated Animals may Help us Combat Antibiotic Resistance
Insects – which comprise about 85% of animal biodiversity and about 55% of total planetary biodiversity – can rapidly clear microbial infections by producing a variety of immune-induced cytokine-like molecules including antibacterial, antifungal peptides/polypeptides, which even may have anticancer activities. Indeed, antimicrobial peptides from insects have been reported to counteract antibiotic resistance [Reference Manniello, Moretta and Salvia74] and prevent skin cancer [Reference Tonk, Vilcinskas and Rahnamaeian75] (as it can be formulated as ointments and creams). As antimicrobial peptides are potent at low concentrations and have high specificity with low toxicity towards normal cells, their biological properties could be utilised as novel biopharmaceuticals for both prophylactic and therapeutic applications [Reference Chernysh, Kim and Bekker76]. Antibiotic resistance is a major threat to global health, which has been accelerated by the misuse of antibiotics, both in animals and humans. As gut bacteria of animals living in polluted environments, such as cockroaches, could be a potential source of anti-bacterials [Reference Akbar, Siddiqui, Sagathevan and Khan77], therapeutic anti-bacterials for potential human use may already exist in some of the world’s most unappreciated animal species.
Metabolic Magicians in Nature
The kidneys of Weddel seals are protected from hypoxia despite severe renal vasoconstriction upon extended periods of deep-see diving [Reference Davis, Castellini, Kooyman and Maue78]. Although the exact mechanism(s) enabling this are unknown, it could occur through the upregulation of antioxidants. Indeed, fasting seals display increased expression of Nrf2 [Reference Vázquez-Medina, Soñanez-Organis and Rodriguez79], antioxidant enzymes [Reference Vázquez-Medina, Zenteno-Savín, Elsner and Ortiz80] and glutathione levels [Reference Vázquez-Medina, Zenteno-Savín, Forman, Crocker and Ortiz81]. In keeping with a thesis of such a protective role for antioxidants, increased expression of Nrf2 has been reported to prevent progression of tubular damage after renal ischemic injury in mice [Reference Nezu, Souma and Yu82]. Other amazing features that can inspire researchers to find new solutions to lifestyle diseases, such as diabetes and obesity, include the resistance to diabetic complications in hummingbirds. These small flying powerhouses possess the highest mass-specific metabolic rates known amongst vertebrates and increase their body fat >40% before migration [Reference Hargrove83]. As they almost exclusively feast on nectar sugar, they face extreme challenges to meet the high metabolic fuel requirements. Although this leads to blood glucose levels up to 42 mmol/L [Reference Beuchat and Chong84], the poor metabolic control does not seem to cause the neurological, renal and microvascular pathologies that would be found in diabetic humans [Reference Ali, Dick and Muhammad85]. The anti-obesity effects of a glucagon-like receptor agonist from the saliva of the Gila monster should also be mentioned [Reference Alves, Abdalla, Alponti and Silveira86].
Alcoholism is a major global health problem that can induce hepatic steatosis with liver dysfunction and oxidative stress. Nrf2 may play an important protective role against acute alcohol-induced hepatic and pancreatic damage and Nrf2-KO mice have a defective hepatic acetaldehyde (the major toxic metabolite of alcohol) metabolism [Reference Sun, Fu and Zhong87]. Tree shrews – a small mammal native to the tropical forests of Southeast Asia – is a close relative of primates in terms of evolution. They have some dietary traits in common with humans; one of them ingestion of alcohol on a regular basis. Due to daily intake of fermented floral nectar from the Bertram Palm (the highest alcohol concentration reported in a natural food), the pen-tailed tree shrew has high concentrations of the alcohol metabolite ethyl glucuronide, but with no signs of intoxication [Reference Wiens, Zitzmann and Lachance88]. It has been estimated that they consume intoxicating levels of alcohol about twice per week assuming alcohol metabolic rates similar to humans. The mechanisms that have evolved to protect tree shrews from repeated alcohol intoxication may provide clues for the treatment of chronic alcoholism and a model for studying alcohol-induced fatty liver disease [Reference Xing, Jia and He89]. The p53 family proteins are an evolutionarily conserved group of transcription factors that emerged at the start of multicellular life over 1 BYA [Reference Dötsch, Bernassola, Coutandin, Candi and Melino90]. p53 plays a role in protecting organisms from genotoxic stresses, in part via enhancing the protein level of Nrf2 [Reference Chen, Jiang and Wang91]. As p53 protective mechanisms include DNA damage induced by acetaldehyde, it is of interest that the p53 protein in tree shrews is considerably more thermostable than human p53, which may lead to superior maintenance of genomic integrity and protection of acetaldehyde-induced DNA damage [Reference Nakagawa, Sakaguchi, Nomura, Kamada, Omichinski and Sakaguchi92].
Lessons from Animals with an Increased Risk of a Disease
Obligate carnivores, such as the domestic cat, tigers and lions, have a markedly increased risk of developing CKD [Reference Brown, Elliott, Schmiedt and Brown93,Reference Junginger, Hansmann and Herder94]. Amongst geriatric domestic cats, 35%–80% develop CKD; the most common cause of death in domestic cats >5 years of age [Reference Brown, Elliott, Schmiedt and Brown93]. In accordance, renal injuries were reported in 87% of tigers, leopards and lions in German zoos and safari parks [Reference Junginger, Hansmann and Herder94]. Given the evolutionary law of “survival of the fittest,” it is unlikely that wild felids have evolved a predisposition to CKD. Thus, the dramatic increase in CKD might reflect climate change and/or environmental change, in which felids are particularly vulnerable [Reference Zanin, P, alomares and Mangabeira Albernaz95]. In contrast to domestic and zoo felids, which are often fed high-protein diets on a daily basis, dietary acquisition of protein in the wild is intermittent and separated by days of fasting without prey. As fasting drives muscular Nrf2-related antioxidant responses [Reference Lettieri-Barbato, Giuseppina Minopoli and Caggiano96], further studies should relate to the common occurrence of fasting in the animal kingdom to survival in extreme environments. Although several different causes may explain the increased risk of CKD in felines, veterinary observations support epidemiological studies that have shown that increased intake of red meat increases the risk of CKD [Reference McClelland, Christensen and Mohammed97,Reference Lew, Jafar and Koh98]. The high protein and phosphate intake that accompanies the intake of red meat and animal-based foods are likely contributory. A recent study based on the Korean Genome and Epidemiology Study concluded that in the general population, a high-protein diet increases the risk of hyperfiltration and a rapid decline in renal function [Reference Jhee, Kee and Park99]. Thus, high-protein regimens including meat and other animal-based foods, such as the Atkins diet, to reduce the risk of obesity and diabetes may be bad for kidney health [Reference Kalantar-Zadeh, Kramer and Fouque100]. Another culprit in felids may be hyperphosphatemia due to increased intake of phosphate that accompanies a high intake of protein. In mammals, serum phosphate level is inversely related to lifespan, thus it has been speculated that this element promotes CKD, cardiovascular disease and shortened lifespan [Reference Stenvinkel, Painer and Kuro-O101]. In the general population, high dietary intake of phosphate is associated with increased all-cause mortality [Reference Chang, Lazo, Appel, Gutiérrez and Grams42].
Negligible Senescence and Environmental Stress – What is the Role of Nrf2?
Studies across a range of different animal species have shown that superior mitochondrial biogenesis and resistance to inflammation and oxidative stress, again via upregulation of Nrf2, protects animals in extreme habitats [Reference Stenvinkel, Painer, Johnson and Natterson-Horowitz31] against multi-stress genotoxicity. Indeed, in a wide variety of species, such as bats [Reference Yin, Zhu, Liu, Irwin, Zhang and Pan102,Reference Yin, Ge, Liao, Liu, Zhang and Pan103], bears [Reference Chazarin, Ziemianin and Evans41], seals [Reference Vázquez-Medina, Soñanez-Organis and Rodriguez79], the Burmese python [Reference Andrew, Perry and Card69] and long-lived species, such as naked mole rats [Reference Lewis, Wason, Edrey, Kristan, Nevo and Buffenstein104], and ocean quahog [Reference Stenvinkel and Shiels105], an upregulated Nrf2 system seems to be protective in an extreme natural habitat. Current evidence suggests that a common mechanism mediating outstanding stress resistance in long-lived mammals are the maintenance of protein homeostasis, superior protection against oxidative stress and robust mitochondrial function [Reference Stenvinkel and Shiels105]. In accordance, negligible senescence in sea urchins is accompanied by a lack of accumulation of cellular oxidative damage [Reference Du, Anderson, Lortie, Parsons and Bodnar106]. Studies on naked mole rats [Reference Debebe, Biagi and Soverini107] and hibernating bears [Reference Sommer, Ståhlman and Ilkayeva44] also support a role of a favourable gut microbiome in healthy aging. A protective role for Nrf2 in the aging process is further supported by low Nrf2 expression in children with extreme premature vascular aging due to Hutchinson-Gilford Progeria syndrome [Reference Kubben, Zhang and Wang108]. Additionally, a recent study conducted in Nrf2 k/o mice sent into space has revealed that Nrf2 deficiency induces aging-like changes in plasma metabolites and weight gain [Reference Suzuki, Uruno and Yumoto109]. While important lessons on protective mechanisms in aging can be learned from long-lived fish with negligible senescence, such as the Greenland shark (>400 years) and Bowhead whale (>200 years), short-lived fish, such as killifish (13 weeks) have emerged as an important natural animal model for aging research [Reference Stenvinkel and Shiels105].
While nature has allowed the evolution of homeostatic systems to counteract metabolic extremes within the body, the Nrf2 antioxidant pathway may also play a role in resistance to allostatic environmental stress. Heat extremes are increasing in frequency around our planet and affect both human and animal well-being, especially during the hot summer period. Heat stress affects normal development and differentiation at both the cellular and the organismal levels. It induces inflammation, mitochondrial damage with oxidative stress [Reference Slimen, Najar, Ghram, Daddebi, Mrad and Abdrabbah110] and premature senescence with cell cycle arrest [Reference Shimoni, Goldstein and Ribarski-Chorev111], all features of the dysregulated aging process. Many of the observed adverse health effects caused by inhaled particular matter are also associated with mitochondrial damage, oxidative stress and inflammation [Reference Pardo, Qiu, Zimmermann and Rudich112]. As ROS reacts with cellular macromolecules, including DNA and proteins, environmental stress is likely to increase the risk of mutation and protein misfolding [Reference Cooke, Evans, Dizdaroglu and Lunec113]. Inflammation, mitochondrial stress and oxidative stress are closely related to the “diseasome of aging” [Reference Stenvinkel4]; that is, it is likely that global warming, air pollution and other environmental stresses will increase the incidence of burden of lifestyle diseases. In this respect, it is interesting and promising that nutritional stimulation of Nrf2 with resveratrol [Reference Sahlin, Orhan, Akdemir, Tuzcu, Iben and Sahlin114], hydrolysed camel whey protein [Reference Du, Lv and Su51] and curcumin [Reference Zhang, Bai and Su115] protects against thermal stress in animals. Moreover, sulforaphane – a potent nutritional Nrf2 agonist – activates heat shock transcription factor 1-mediated heat shock response [Reference Gan, Wu and Brunet116]. Equally interesting are studies showing that Nrf2 stimulation by Juglanin isolated from knotgrass protects against prolonged PM2.5 exposure-triggered liver inflammation [Reference Ge, Tan and Zhong117] and RT-408 protects against ozone-induced asthma [Reference Zhang, Yang, Chen, Zhang and Li118]. Finally, tyrosol from olive oil prevented the toxic effects of aluminium in rats via activation of the Nrf2-Keap1 pathway [Reference Güvenç, Cellat and Gökçek119], which has been reported as a secret weapon against pesticide persecution in Drosophila [Reference Chem, Zhaing and Ge120].
It would be naïve to think that the examples given in this text regarding the putative merits of stimulation of the cytoprotective transcription factor Nrf2 could be the one and only solution. There will never be a “silver bullet” and it should be emphasised that overstimulation of Nrf2 may have adverse effects [Reference Wakabayashi, Itoh and Wakabayashi121]. However, considering emerging data in the literature showing the importance of this evolutionary conserved cytoprotector for animal species living in extreme environments and studies showing that Nrf2 activation protects animals from heat stress, pollution, pesticides, etc., it is important to further study the role of this system for the benefit of planetary health. It may be one of the low-hanging fruits, where the concept of “Food as medicine” may come of age and have an impact on future pandemics and non-communicable disorders alike. After all, humans have been safely ingesting nutritional Nrf2 stimulators in plant and fermented food since ancient times.
Loss of Biodiversity Means Loss of Possible Solutions to Improve Human Health
In the “Living Planet Report 2020,” the World Wildlife Fund reported an alarming 68% decline in the animal population between 1970 and 2016 (https://livingplanet.panda.org/en-us/). This loss of biodiversity undermines the very foundations of the planet’s livelihood, health and quality of life. In a modelling analysis, Smith et al [Reference Smith, Singh, Mozaffarian and Myers122] reported that complete removal of animal pollinators will have a dramatic effect on global health due to micronutrient deficiencies and non-communicable diseases. The loss of biological diversity, from genetic to ecosystem level, means that we are missing out on a unique opportunity to identify biomimetic solutions to human diseases (Fig. 4). There are already multiple cases demonstrating the virtue of such solutions, a few are presented below.
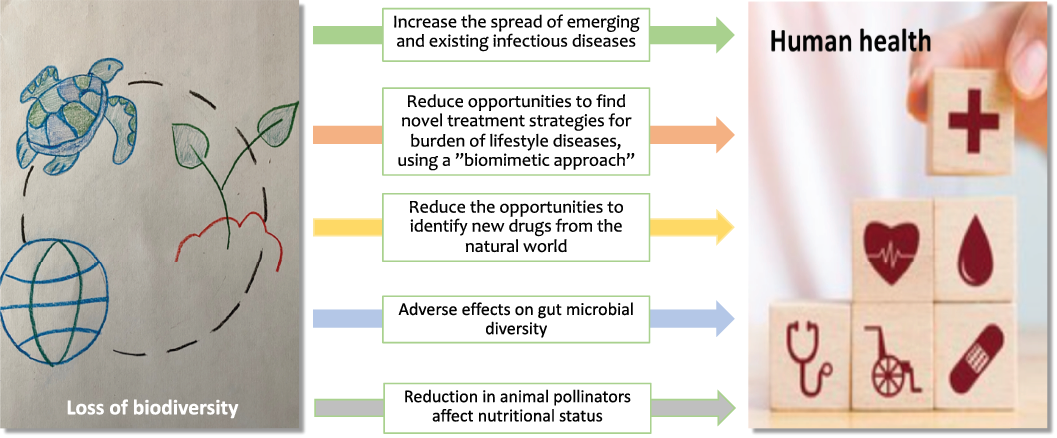
Fig. 4. Direct or indirect mechanisms by which loss of biodiversity may affect human health.
One of the best examples of a successful biological biomimetic application is the development of the antihypertensive drug captopril from the poisonous Brazilian viper, whose effect on the renin–angiotensin system mimics the snake’s venom. It is likely that in the toxins from the >3000 different snakes that exist on the planet, interesting substances for drug development will be identified. As <0.01% of the planet’s snake venom has so far been identified and characterised [Reference Mohamed Abd El-Aziz, Soares and Stockland123], the forests of our planet are full of potentially novel drugs. The possibility of using venom from honey bees, Israeli scorpions and a marine mantle as a future treatment for cancer should also be considered [Reference Noble, Rohaj, Abegglen and Schiffman124]. About one-third of the drugs we use today have originated from nature; the development of future drugs is dependent on humankind preserving the diversity of nature. With >50,000 species of different plants in the Amazonas, it is of the utmost importance that ongoing deforestation is prevented, not only for the preservation of the environment – but also for health reasons. Clearance and disruption of Amazonian forests is one of the greatest threats to our planet’s biodiversity conservation and may increase the risk of future pandemics.
Emerging evidence suggests that in parallel with the loss of planetary biodiversity, there is a loss of human gut bacterial biodiversity. Burden of lifestyle diseases associated with the “diseasome of aging” are in general characterised by poor eating habits, dysbiosis and loss of microbial diversity [Reference Shiels, Buchanan, Selman and Stenvinkel7]. A consequence of this is that bacterially derived short-chain fatty acid production will be altered, leading to epigenetic dysregulation and eventually morbid conditions, such as obesity [Reference Austvoll, Gallo and Montag125]. In response to the deforestation of Amazonian forests, the majority of observed impacts on soil biodiversity, microbial biomass, richness and diversity indexes were negative [Reference Franco, Sobral, Silva and Wall126]. Thus, loss of planetary biodiversity may drive loss of the gut bacterial biodiversity. As the plants that feed our planet need a rich and bio-diverse soil, deforestation may affect both planetary and gut biodiversity. Moreover, as plant foods are the richest source of pro-Nrf2 compounds (such as sulforaphane, physethin, curcumin and quercetin), loss of soil biodiversity and quality due to intensive monoculture and deforestation may reduce the health-promoting effects of plant food [Reference Mafra, Borges, Lindholm, Shiels, Evenepoel and Stenvinkel127]. It may seem ironic now that we are beginning to receive evidence that tailor-made bioactive nutritional treatment can affect lifestyle diseases [Reference Mafra, Borges, Lindholm, Shiels, Evenepoel and Stenvinkel127], this development is threatened by a disturbed external environment with global warming, greenhouse gas emission, air pollution and deforestation. The impact on burden of lifestyle diseases and gut microbial biodiversity of the global trend of migration from rural to urban areas also need attention. One recent study has shown that when sparrows migrate from rural to urban areas, this is not only associated with a loss of gut microbiota diversity, but also fewer metabolic functions in cities [Reference Teyssier, Rouffaer and Hudin128]. Another study, using functional magnetic resonance imaging, links the urban environment to vulnerability in certain brain regions and social stress [Reference Lederbogen, Kirsch and Haddad129].
What both evolution and the natural world have illustrated to mankind is that it is not advisable to stand still. Consequently, physical activity (exercise) is often a form of medicine, which can enhance physiological capabilities, both via stimulation of the immune system [Reference Simpson, Cosgrove and Chee130] and via upregulation of Nrf2 [Reference Vargas-Mendoza, Morales-González and Madrigal-Santillán131]. The sedentary lifestyle in modern man does not lend itself to the maintenance of peak physical activity. Additionally, socioeconomic factors interplay with modern lifestyles to exacerbate this situation. Indeed, neighbourhood stressors associated with socioeconomic deprivation have been linked with obesity and its sequelae, including diabetes and poor cardiovascular health, particularly amongst women. This has been attributed to a reluctance to take suitable levels of exercise when living in adverse social environments and to adopt more sedentary behaviours [Reference Ellaway, Dundas, Olsen and Shiels132]. Biomimentics may help here by identifying more salutogenic environmental conditions that may be incorporated into urban environments.
Has the Therapeutic Dilemma in COVID-19 Already Been Solved in Nature?
Emergence of zoonotic diseases – one of the greatest threats to biodiversity and human health – is a complex process involving not only wildlife and natural ecosystems, but also societal aspects. It has been speculated that permafrost melting due to global warming will result in the release of bacteria and virus (currently blocked in ice) may increase the risk of future epidemics [Reference Charlier, Héry-Arnaud and Coppens133]. The effects of the ongoing COVID-19 pandemic on human welfare, socioeconomic and political structures are, and will be, enormous. As humans destroy ecosystems through intensive land exploitation and trade in wildlife, we run the risk of being exposed to bacteria, viruses or parasites that spread between animals and humans. Climate change may have played a key role in the evolution or transmission of viruses, such as SARS-Cov-2. Indeed, changes in temperature (and other events associated with climate change) influence the reservoirs of viral infections, their transmission by insects and other vectors. It was recently reported that the Southeast Asian region is a global hotspot of climate change-driven increase in bat-borne coronaviruses [Reference Beyer, Manica and Mora134]. It has also been suggested that there is a need to monitor Southeast Africa and South America for future pandemics, as these regions amalgamate many of the circumstances that could create a perfect storm for new hotspots [Reference Frutos, Serra-Cobo, Pinault, Lopez Roig and Devaux135]. For the prevention and treatment of viral pandemics, much can be learned from nature. Even before the current COVID-19 pandemic, various zoonotic diseases such as Ebola, HIV, rabies caused millions of deaths each year [Reference Kreuder Johnson, Hitchens and Smiley Evans136].
The therapeutic dilemma in severe COVID-19 is the combination of hyper-inflammation (cytokine storm) with a reduced interferon response [Reference Blanco-Melo, Nilsson-Payant and Liu137]. In the worst-case scenario, this combination results in a pronounced vascular inflammation, endothelial dysfunction, hypercoagulability, acute respiratory distress syndrome, multi-organ failure and death [Reference Poor, Ventetuolo and Tolbert138]. Research conducted before the COVID-19 pandemic established a protective role for Nrf2 in multiple components of severe COVID-19 disease, such as respiratory distress [Reference Liu, Gao and Ci139], endothelial injury [Reference Priestley, Kautenburg, Casati, Endres, Geurts, Julian and Lombard140], hypercoagulability [Reference Akin-Bali, Eroglu, Ilk, Egin and Kankilic141] and the cytokine storm [Reference Kobayashi, Suzuki and Funayama142]. COVID-19 patients with severe symptoms usually have lower interferon responses than patients with mild symptoms and SARS-CoV-2 ORF3b encodes a potent interferon antagonist [Reference Konno, Kimura and Uriu143].
Bats have over 64 MA of adaptive evolution developed features that are unique amongst mammals, such as a long lifespan relative to body size, a low rate of tumourigenesis and an exceptional ability to host viruses without presenting clinical disease [Reference Irving, Ahn, Goh, Anderson and Wang144]. As many of the planet’s ≈1200 bat species harbour viruses, such as Ebola and SARS-Cov-2, with at most mild symptoms, biomimetic inspiration for how virus infections should be handled can be obtained through studies of bats. During evolution, bats have developed an elegant solution to harbouring multiple infectious viral strains, as they control hyper-inflammation via upregulated Nrf2 expression [Reference Yin, Zhu, Liu, Irwin, Zhang and Pan102], target the inflammasome pathway at multiple levels, mitigating potential immune-mediated tissue damage and disease [Reference Goh, Ahn and Zhu145], while at the same time exhibiting robust interferon-based defences. A recent study including 26 species of bats reported that DNA methylation changes are associated with innate immunity or tumourigenesis genes, reinforcing the view that bat longevity results from cancer suppression and augmented immune responses [Reference Wilkinson, Adams and Haghani146]. A recent clinical study has supported the biomimetic findings in bats. It has shown that robust interferon and Nrf2 systems play a significant role in protection against SARS-CoV-2; that is, the Nrf2 system was downregulated in COVID-19 patients and Nrf2 agonists (such as dimethyl fumarate) have potent interferon-independent inhibitory effects on both virus replication and hyperinflammation [Reference Olagnier, Farahani and Thyrsted147]. It is also evident that antibodies and/or mutations that inhibit the interferon response promote life-threatening cases of COVID-19 [Reference Wadman148]. The recently reported role of the gut microbiota in interferon secretion (by Bacteroide) and the natural resistance against COVID-19 [Reference Stefan, Kim, Iwasaki and Kasper149] are of major interest. Furthermore, vaccination with live vaccines (such as BCG and measles) trains our immune system and increases interferon levels, which can contribute to lower mortality [Reference O’Neill and Netea150]. Interferon production decreases with age, comorbidity [Reference Uno, Yagi, Yoshimori, Tanigawa, Yoshikawa and Fujita151] and stress [Reference Glaser, Rice, Speicher, Stout and Kiecolt-Glaser152]. Thus, this could partly explain why mortality in COVID-19 is related to age, comorbidity and more often affects people in socially disadvantaged areas. The mortality rate in COVID-19 is higher in areas with chronic exposure to lower air quality [Reference Pan, sini and Fornaca153] and chronic sterile inflammation in the elderly may in itself be an important risk factor for a serious course of COVID-19 [Reference Akbar and Gilroy154].
In order to survive stressful environmental conditions bats usually hibernate. Normally, viruses are harboured in bats without a high risk of spill-over to other species, but if the bat becomes stressed (e.g., becomes trapped, threatened, or undergoes an infection), the titre and spread of virus increase significantly. As an example, the intestines of virus-infected bats that were also infected with a fungus (white-nose syndrome) contained 60-fold more coronavirus RNA than bats with virus alone [Reference Davy, Donaldson and Subudhi155]. As intestinal organoids from both bats and humans can be infected by SARS-CoV-2 [Reference Zhou, Li and Liu156], the gastrointestinal transmission route of COVID-19 needs attention when discussing cause(s) of transmission. It is not hard to imagine that the mixing of different animal species (that normally not interact in nature) at Southeast Asian food markets could transmit viruses from bat intestines to other species [Reference Wacharapluesadee, Tan and Maneeorn157].
The bat is the only known mammal that has developed the ability to fly. This extraordinary skill comes at an enormous expense, as 15–16 times higher metabolism is required (compared to twofold increased metabolism in birds) for them to fly. The extremely high metabolism in bats not only increases the risk of ROS generation and DNA damage, but also causes a fever of 38–41°C that may in itself reduce the viral load [Reference Rodriguez, Taketa and Madhu73]. The high risk of DNA damage may be one reason why bats, over evolution developed increased expression of Nrf2 [Reference Yin, Zhu, Liu, Irwin, Zhang and Pan102] for protection during hibernation [Reference Yin, Ge, Liao, Liu, Zhang and Pan103] in a state of increased endoplasmic reticulum stresses [Reference Huang, Liao and Han158]. The extraordinary immunology system of camelids also needs mentioning. It was recently reported that cross-reactive single-domain camelid antibodies from llama could be a potential therapeutic candidate for COVID-19 [Reference Wrapp, De Vlieger and Corbett159].
Amongst a number of potential treatments for severe COVID-19, it has been suggested that nutritional Nrf2 activation, especially when combined with transient receptor potential ankyrin 1 (TRPA1), represents a potential path out of the COVID-19 pandemic [Reference Pasini, Straieri, Cominacini and Mozzini160]. Recently, three experimental clinical cases using broccoli and glucoraphanin have provided a proof of concept, confirming the hypothesis that Nrf2-interacting nutrients are effective in COVID-19 [Reference Bousquet, Le Moing and Blain161]. The pro-Nrf2 protective effect of cabbage and fermented food on COVID-19 [Reference Bousquet, Anto and Czarlewski162], is also of interest when linking nutritional habits to a “Covid-19 protective phenotype.” The emerging links between severity and immune dysfunction in COVID-19 and gut microbiota composition may provide clues for nutritional interventions that may limit the severity of the infection [Reference Yeoh, Zuo and Lui163]. The benefits of nutritional Nrf2 agonists for COVID-19 (and burden of lifestyle diseases) as part of a “Food as medicine” concept [Reference Mafra, Borges, Lindholm, Shiels, Evenepoel and Stenvinkel127] needs to be assessed through large studies by employing a double-blind, placebo-controlled design to stand on a robust scientific ground. It is likely that the planet will have a poor protection from future pandemics unless we protect the planet’s food systems.
Urgent Need of a Radical Transformation in Global Food Systems
The health effects of poor dietary habits on the global burden of disease risks was recently shown in a systematic analysis including 195 countries, where it was revealed that 11 millions of deaths and 255 millions of disability-adjusted life years (DAILYs) were attributable to dietary risk factors [164]. Amongst these factors, high sodium intake, low intake of whole grains and low intake of fruits were the leading dietary factors contributing to the increased mortality rates and to the loss of DAILYs [164]. Summing up these figures, unhealthy diets (increased amount of salt, low in whole grain and fruits) is the largest global burden of disease and lead to a higher increase in morbidity and mortality than does unsafe sex, alcohol, drug and tobacco use together [Reference Willett, Rockström and Loken165]. Thus, it is becoming painfully clear that the food systems developed in the modern world represent a “triple whammy” that not only promotes burden of lifestyle diseases, but also contributes to climate change and loss of biodiversity [Reference Clark, Springmann, Hill and Tilman166]. Processed food – the key hallmark of Western diet – promotes weight gain and drives microvascular disease and inflammation via increased intestinal barrier permeability [Reference Snelson, Tan and Clarke167]. Biomimetic studies of mammals living in extreme environments underpin the role of dietary habits, periods of fasting and the gut microbiota in survival. A carnivorous diet and processed food are detrimental to human health [Reference Zinöcker and Lindseth168] and is associated with the “diseasome of aging” [Reference McClelland, Christensen and Mohammed97]. Thus, the transformation of global food systems may be the single most important factor to improve global health. Innovations in food system will also be instrumental if we should achieve the UN’s multiple Sustainable Development Goals (SDGs). Global food production is a key source of greenhouse gas emission – emitting 24% of the global total greenhouse gases (https://www.ipcc.ch/srccl/). Meeting the 1.5°C target for global warming, which is identified as critical by the UN Environment Programme, requires rapid and ambitious changes in food systems [Reference Clark, Domingo and Colgan169]. Food production is the largest water-consuming sector with the share of water withdrawn varying from 21% in Europe to 82% in Africa [Reference Willett, Rockström and Loken165], and with a global average of 70% of water withdrawal used for irrigation. As the transformation of croplands and pastures is the largest factor causing species extinction [Reference Tilman, Clark, Williams, Kimmel, Polasky and Packer170], food has been considered as the single strongest lever to optimise human health and environmental sustainability [Reference Willett, Rockström and Loken165].
A plant-based diet aligned with the concept of “Food as Medicine” [Reference Mafra, Borges, Lindholm, Shiels, Evenepoel and Stenvinkel127] targeting a foodome of >25,000 substances that make up the human diet, offers an alternative path to evidence-based non-pharmacological treatments to improve both health and the environment. The effectiveness of “Food as Medicine” for the treatment of human diseases has been evident since the 17th century, from when it was reported that citrus fruits prevented scurvy in sailors. Subsequent reports in the mid 20th century support the use of “Food as medicine” for the treatment of a number of diseases such as pellagra, beriberi, anaemia, rickets. That the concept “Food as Medicine” has the potential to be a clinically viable route is exemplified by a study showing that the treatment with broccoli sprouts (rich in the Nrf2 agonist sulforaphane) can be as effective as metformin in difficult-to-control type-2 diabetes [Reference Axelsson, Tubbs and Mecham171]. Moreover, urolithin A, a major microbial metabolite derived from polyphenolics of berries and pomegranate fruits, upregulates epithelial tight junction proteins through activation of Nrf2-dependent pathways to enhance gut barrier integrity [Reference Singh, Chandrashekharappa and Bodduluri172]. An additional and important point to consider, pertinent to the modern Western diet, is the radical changes in food preservation that have occurred over history. The fermentation process (introduced during the Neolithic age to enable humans to eat “not-so-fresh” food and survive) converts components of fruits, vegetables, etc., to Nrf2 activators. As Lactobacillus bacteria, the dominant species in the fermentation process, activate Nrf2 [Reference Li, Evivie and Lu173], a link between healthy gut microbiota as a protection against oxidative stress and inflammation and reduced risk of disease is established. The absence of alkyl catechols from the modern diet may have serious negative consequences for Nrf2 cell defence, resulting in reduced protection against burden of lifestyle diseases [Reference Donald, Senger, Li, Jaminet and Cao174]. However, robust clinical evidence for precise medical effects of “Food as Medicine” is still anticipated. As it was recently reported that with a combination of more plant-based human diets and reduced food waste more than two-thirds of future biodiversity losses could be avoided [Reference Leclère, Obersteiner and Barrett175], we still have an opportunity to make a difference for the next generations.
Next Steps – Could Biomimetics help us Improve Planetary Health?
There are several reasons why protecting the environment and the biodiversity of our planet will have profound impacts on human health. If the “wise man” wants to survive on our planet, we must gather knowledge from multiple perspectives and actively engage in studies of planetary health. The real opportunity now lies in adopting a strategy and securing funding for the whole health of the planet, where studies of human health are integrated with studies of the environment and animal health. Such studies are currently lacking, and we call for the creation of novel interdisciplinary research teams. An important obstacle is that traditional studies are funded in narrowly defined silos of current granting bodies. We call for the creation of novel granting mechanisms that transcend national and disciplinary boundaries, effectively targeting planetary health. In order to better prevent and treat the lifestyle diseases that increase with age, we can learn a lot from solutions already developed in nature. With a biomimetic approach, we should be able to learn from the ingenious solutions that have been developed in nature for the better. Unfortunately, many of our best scientific efforts today remain siloed, fragmented or rivalrous. As knowledge must be gathered from multiple perspectives, a multidisciplinary collaboration where medical doctors, natural scientists, veterinarians, climate scientists, ecologists, wildlife biologists and anthropologists meet at the intersection is required [Reference Stenvinkel, Painer, Johnson and Natterson-Horowitz31]. A reformed medical curriculum that includes planetary health and biomimetics would help to achieve this. If we are to adopt a more biomimetic approach in research, immediate action is required, as a rapid loss of species diversity and habitats may prevent this opportunity to learn from solutions developed in nature.
Acknowledgments
PS was supported by the Swedish Heart and Lung Foundation, CIMED, Westman and the Strategic Research Programme in Diabetes at Karolinska Institutet (Swedish Research Council grant No. 2009–1068. PSh is funded by IPP awards with 4D Pharma and Constant Pharma.
Disclosures
PS is on scientific advisory boards of REATA, Baxter, Vifor and Astra Zeneca. PGS is funded by awards from 4D Pharma, Constant Pharma and acts as a consultant for Mars UK. The remaining authors have no conflicts of interest to declare.