Introduction
Dietary fructose consumption in the USA has risen markedly in the past four decades and along with increased total sugar consumption has been correlated with the increased prevalence of the metabolic syndrome [Reference Rodriguez, Madsen, Cotterman and Lustig1]. During the same period, non-alcoholic fatty liver disease (NAFLD) has increased with over 25% prevalence in the adult US population in the context of the obesity epidemic [Reference Younossi, Koenig, Abdelatif, Yousef, Henry and Wymer2]. NAFLD may lead to non-alcoholic steatohepatitis (NASH), cirrhosis, and liver cancer [Reference Calzadilla Bertot and Adams3]. Fructose feeding induces fatty liver disease in rodents [Reference Stanhope, Schwarz and Havel4] and zebrafish [Reference Toop and Gentili5] and has been shown to trigger or worsen NAFLD in humans [Reference Chen, Zheng and Zhang6]. A fructose bolus in humans increases serum triglycerides and palmitate [Reference Hudgins, Parker, Levine and Hellerstein7] and nine days of a diet including 25% of total calories as fructose increases hepatic fatty acid synthesis [Reference Schwartz, Noworolski and Wen8], which is reversed by substitution of fructose by starch [Reference Schwartz, Noworoloski and Erkin-Cakmak9]. In monkeys, fructose supplementation leads to hepatic inflammation and hepatic fibrosis [Reference Kavanagh, Wylie and Tucker10]. Much of this work has recently been summarized [Reference Alwahsh and Gebhardt11]. The current mechanistic hypothesis for these effects focuses on fructose-induced changes in hepatic lipid metabolism [Reference Esler and Bence12]. Fructose undergoes first pass metabolism in the liver [Reference Havel13] and is a substrate for de novo lipogenesis driving triglyceride accumulation which causes cellular injury [Reference Stanhope, Schwarz and Keen14].
However, there is also preclinical data suggesting that at least part of the ill effects of excess fructose occur from changes in the gut. Small intestinal fructose absorption is limited compared to glucose absorption. Many individuals cannot absorb more than about 25–50 grams of fructose given as a bolus [Reference Rao, Attaluri, Anderson and Stumbo15]. Fructose absorption is limited because of selective absorption mechanisms for small intestinal transport of fructose [Reference Jones, Butler and Brooks16]. Unabsorbed fructose passes into the colon where it is rapidly fermented by gut bacteria into short chain fatty acids, hydrogen, carbon dioxide, and methane [Reference Gibson, Newnham, Barrett, Shepherd and Muir17]. Thus, large amounts of dietary fructose not absorbed in the small intestine passing into the colon might rapidly alter the distribution and function of colonic microbiota [Reference David, Maurice and Carmody18] leading to changes in microbial metabolite production. Microbial changes after feeding diets high in fructose content have been described in rats [Reference DiLucca, Crecenzo and Mazzoli19]. One consequence of fructose-induced changes in gut microbiota and metabolites in rodents is increased intestinal permeability. In mice, increased fructose feeding alters intestinal tight junctions [Reference Kawabata, Kanmura and Morinaga20]. Antibiotics or transfer of feces from chow-fed rats into the colon of fructose-fed rats reverses gut microbial changes with amelioration of the metabolic syndrome [Reference DiLucca, Crecenzo and Mazzoli19]. Altered fecal microbiota have been described in human fatty liver disease [Reference Boursier, Mueller and Barret21], and probiotics may reduce liver fat [Reference Ahn, Jun, Kang, Lim, Lim and Chung22].
Increased intestinal permeability has been described in NASH [Reference Luther, Garber and Khalili23] accompanied by endotoxemia [Reference Harte, DaSilva and Creely24]. Such endotoxemia is known to sensitize hepatic Kupfer cells inducing inflammation in the liver [Reference Chiu, Ching and Li25]. In mice, fructose-induced gut permeability is ameliorated by antibiotics [Reference Volynets, Louis and Pretz26]. However, we have found no studies that determine effects of fructose feeding on the gut microbiome and metabolites, nor on microbial translocation or intestinal permeability in humans.
The present pilot study was designed to test the hypothesis that fructose causes changes in gastrointestinal microbiota that might contribute to fructose-induced liver disease. Positive findings may allow for novel therapeutic approaches aimed at the gut to partially mitigate these deleterious changes in the liver.
Materials and Methods
Subjects
Subjects were recruited from the surrounding community and from the Rockefeller University volunteer database. Eligible were men and postmenopausal women with obesity (body mass index [BMI] 30–39 mg/kg/m2) between the ages of 45 and 70 years, without clinical or electrocardiographic evidence of cardiometabolic disease. Exclusion criteria were fasting blood glucose > 126 mg/dL, HgA1C > 6.5%, serum triglyceride levels > 200 mg/dL, liver function tests > 1.5 times the upper limit of normal, serum uric acid level > 9 mg/dL, current statin, insulin or oral hypoglycemic agents, daily laxatives, probiotics, or proton pump inhibitor usage. Individuals who have a history of broad-spectrum antibiotic therapy during the preceding 45 days, active hepatitis, HIV infection, chronic constipation or diarrhea, inflammatory bowel disease, gastrointestinal resection, or macronutrient malabsorption were also excluded. In addition, current tobacco smokers, and those with a history of more than 8 grams alcohol intake daily were excluded.
Twenty subjects were screened; 13 met the enrollment criteria and were randomized to receive dietary glucose or fructose replacement in the first study arm (Fig. 1) and were admitted to the Rockefeller University metabolic unit. Women were postmenopausal to avoid variation in fecal microbiota that could occur during the menstrual cycle [Reference Koren, Goodrich and Cullender27]. One subject withdrew for personal issues, and two for elevated fasting blood glucose after enrollment. Of 10 participants completing the study, there were six men and four women, two Caucasian, one Black Hispanic, and seven African American, self-reported. All participants read and signed an informed consent document approved by the Institutional Review Board of The Rockefeller University (Protocol PHO-0956).

Fig. 1. CONSOlidated Reporting of Trials (CONSORT) diagram of study participants.
Clinical Study Design and Setting
This was a pilot, double-blind, randomized, cross-over study. Subjects initially were screened in the Outpatient Research Center at the Rockefeller University Hospital. Screening included a complete history and physical examination, fasting blood tests, electrocardiogram, and hip and waist circumference measurements. Subjects met with the bionutritionist for a detailed three-day dietary recall history and completed a computerized diet questionnaire (VioScreen) to determine the participant’s “usual” dietary intake. As fructose is very sweet and can be unpalatable, participants were given taste tests of the fructose solution, mixed in water, lemonade, or tea, to determine which mixture they preferred.
Eligible subjects were randomized by the research pharmacist using SAS 9.4 and the PROC PLAN procedure resulting in four subjects receiving fructose replacement first and then glucose replacement, and six receiving glucose replacement first followed by the fructose phase. Stratification for gender was balanced within each sequence. To prevent rapid changes in the distribution of fecal microbiota with introduction of a new diet, subjects throughout the study were fed their “usual” diets mimicking diets which they consumed prior to entering the hospital. The daily 75 grams of study sugar administered to subjects was accompanied by removal of 75 grams of complex carbohydrate from the “usual” diets. The baseline usual macronutrient intake of our subjects was similar to a Western style diet consumed by many Americans.
Subjects were admitted to the inpatient metabolic unit of The Rockefeller University Hospital for 16–18 days in each study arm (Fig. 2). They were fed their “usual” diet for the first 2–3 days while undergoing baseline testing including body composition measurement. Fecal samples were collected for microbiome, metabolome, and calprotectin assays followed by a 4-sugar test of gut permeability. Subjects then started the 14-day fructose or glucose diet arm of the study. Subjects, care providers, and investigators except for the study clinician, were blinded to the assigned intervention, and the study drinks were dispensed by the pharmacist in identical receptacles.
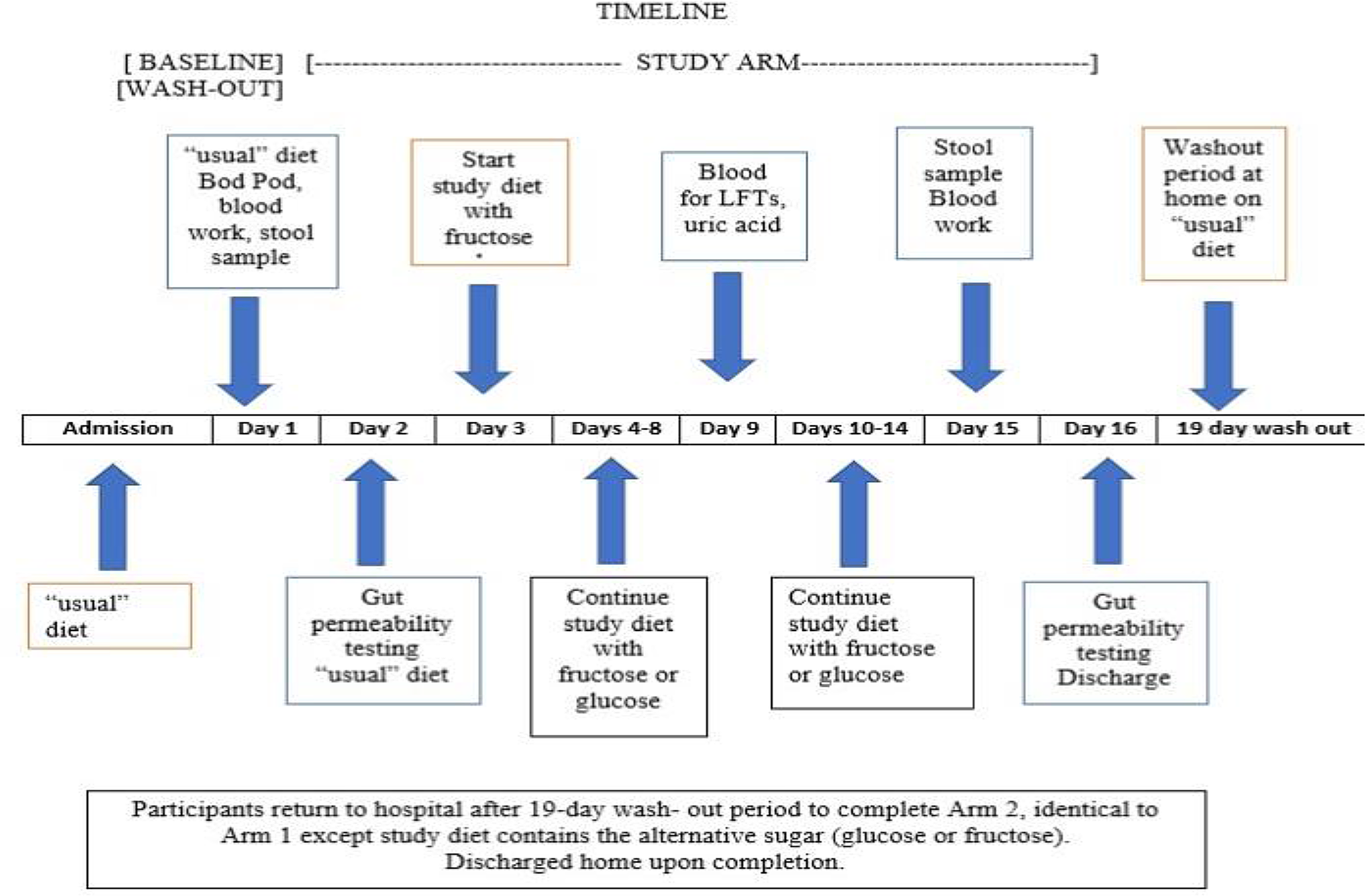
Fig. 2. Study schedule diagram.
Body weight was monitored daily and kept stable by adjusting caloric intake. Activity was monitored by a New Lifestyles NL-800 accelerometer (New Lifestyles, Lee’s Summit, MO). On non-testing days, participants were permitted to leave the hospital after breakfast with a packed lunch, but then returned for dinner. The glucose/fructose solution was prepared by the research pharmacy and administered during breakfast and dinner. The bionutrition staff ensured that food provided was acceptable and consumed, and any food that was not eaten was recorded.
Seven days after starting the glucose or fructose arm of the study, liver function, serum triglyceride, and uric acid levels were monitored as safety measures. At the end of each study arm, the same tests were administered as at the beginning of the study arm (except for the Bod Pod). The subjects returned home for the 17–19-day wash-out period to allow for the gut microbiome to revert toward a baseline state and for the metabolic staff to organize on weekdays the same baseline studies performed in the first arm of the study. While at home, subjects maintained their “usual” diet and received weekly phone contact by the bionutritionist to confirm dietary compliance. On return to the metabolic unit, in the second arm of the study, the subjects underwent the same baseline testing (without the Bod Pod) followed by the 14-day administration of the alternative sugar solution.
Anthropometric Measurements
Body weight was measured daily with a Scale-Tronix 5002 scale (Welch Allyn, Skaneateles Falls, NY) with precision of ±0.1 kg. Subjects were weighed in a hospital gown, after an overnight fast and post-voiding. Height was measured to the nearest 1 cm at baseline with a Seca-216 stadiometer (Hamburg, Germany) in 1 mm increments. BMI was calculated as weight/height squared (kg/m2), using the National Institutes of Health (NIH) Standard Metric BMI calculator.
Daily Blood Pressure Monitoring
After sitting for five minutes, manual blood pressure readings (Welch Allyn aneroid monitor, Skaneateles Falls, NY) were taken each morning.
Diet Formulation Process and Analysis of Dietary Fructose Content Are Provided in Supplemental Material and Methods
Body composition
Bod Pod (Cosmed, Rome, Italy) for body composition assessment is an air displacement plethysmograph which uses whole body densitometry to determine body composition (fat and fat-free mass).
Blood collection and measurements
Fasting blood samples were analyzed in the Clinical Pathology Laboratory of the Memorial Sloan-Kettering Cancer Center for complete blood count, electrolytes, glucose, creatinine, blood urea nitrogen (BUN), aspartate aminotransferase (AST), alanine aminotransferase (ALT), alkaline phosphatase, C-reactive protein, sedimentation rate, and uric acid. Research serum and plasma samples were drawn pre- and post-intervention, aliquoted and stored at −80°C for subsequent analysis.
Bacterial profiling of feces
Immediately after defecating, bean-sized fecal specimens were collected in triplicate from the middle of the stool, placed in 10 mL Falcon tubes and rapidly frozen at −80°C until analysis. Fecal microbiome profiling was performed at MR DNA (Shallowater, TX) as described in the Supplemental Materials and Methods.
Fecal and plasma metabolomics
Fecal and plasma metabolomics were performed at the New York University Metabolomics Core Resource Laboratory as previously described [Reference Pacold, Brimacombe and Chan28]. Samples were subjected to liquid chromatography-mass spectrometry analysis to detect and quantify known peaks (Supplemental Materials and Methods).
Fecal Fructose and Glucose Measurements Are Described in Supplemental Material and Methods
Surrogate markers of microbial translocation and gut inflammation
Circulating proteins associated with an increased plasma lipopolysaccharide content were assessed by measuring lipopolysaccharide-binding protein (Cell Sciences, Inc., Canton, MA) and soluble CD14 concentrations (R&D Systems, Inc., Minneapolis, MN) via an Enzyme Linked ImmunoSorbent Assay (ELISA) following the manufacturer’s instructions. Serum concentration of intestinal fatty acid binding protein (Hycult Biotech, Inc., Wayne, PA) and fecal calprotectin (Genova Diagnostics, Ashville, NC) also were detected by an ELISA following the manufacturer’s instructions and were used as markers of gut damage and inflammation, respectively. All measurements were performed in duplicate.
Gastrointestinal permeability
Gastrointestinal permeability was determined as previously described [Reference Del Valle-Pinero, Van Deventer and Fourie29]. The lactulose/mannitol ratio is used to assess small intestinal permeability and sucralose and sucrose are used to assess colonic and gastric permeability, respectively. In brief, after a baseline urine collection, fasting subjects drank a permeability test solution (100 mL solution containing sucrose [10 g/dL], lactulose [5 g/dL], mannitol [1 g/dL], and sucralose [0.1 g/dL]) and urine was collected for 5 h. Urine was stored at −80°C until analysis. Analysis of sucralose, sucrose, lactulose, and mannitol in urine was performed in duplicate as described in the Supplemental Materials and Methods.
Statistical Analysis
Sample Size and Power Analysis
The primary statistical analysis compares the changes in fecal microbiota distribution between two diets supplemented with fructose or glucose. A cross-over experiment submitted each subject to both diets in a randomized order. A sample of n = 6 would allow 80% power to detect minimum differences as twofold changes in the fecal microbiota distribution at 5% significance with the use of Wilcoxon non-parametric tests. This power analysis is based on a variability that is 20% inflated when compared to the average coefficient of variation (˜40%) reported by DiLucca et al. [Reference DiLucca, Crecenzo and Mazzoli19] when comparing microbiota distribution between two groups of six different rats each. All calculations were performed with G* power software version 3.1.
Results
Participants who successfully completed the study were 57.6 ± 6.2 years of age with class II obesity weighing an average of 101.8 kg (BMI 35.9 ± 3.3), a mean body fat composition of 41.1% and Hemoglobin A1c of 5.5% (Table 1). Three subjects in the fructose arm and one subject in the glucose arm reported abdominal discomfort following the sugar drink. Participants’ activity was similar as judged by pedometer step counts (mean for the fructose and glucose arms: 9523 steps and 9124 steps per day, respectively). These data are similar to step counts calculated for New York City residents.
Table 1. Participant characteristics

BMI, Body mass index = weight in kilograms/height in meters squared.
Fat mass measured by Bod Pod.
The calculated “usual” dietary intake contained a mean of 3258 kcal with 54% of calories as carbohydrates, 15% as protein, and 33% as fat (Table 2). The caloric density and distribution of macronutrients in the designed study diets and the actual consumed diets were very similar to the subjects’ “usual” diets.
Table 2. Predicted and consumed calorie and macronutrient intake

Mean calorie and micronutrient intake predicted from the calculated “usual” intake, the study diet designed by the nutritionists, and the study diet consumed by participants.
Macronutrient composition also shown as percent of total calories.
Cals, calories; % kcal, percent of mean dietary kilocalories; CHO, carbohydrate; SEM, standard error of the mean.
Analysis of Routine Clinical Blood Indices, Microbial Translocation, and Gut Inflammation
No significant differences between the two arms of the study were noted in the routine hematology or biochemistry values including serum lipids, AST, ALT, and uric acid levels (Table 3). Also, no significant differences in any of the surrogate markers of microbial translocation or gut inflammation were found between the two arms. These included serum levels of soluble CD14 and lipopolysaccharide binding proteins as surrogate markers for changes in circulating lipopolysaccharide concentrations (Table 4). Fecal calprotectin and serum levels of intestinal fatty acid binding protein as measures of intestinal inflammation and damage respectively showed no changes between the fructose or glucose arms of the study. All results were within the normal range.
Table 3. Serum AST, ALT, triglycerides, uric acid pre- and post-intervention

AST, aspartate transaminase; ALT, alanine transaminase; U/L, units per liter of serum; mg/dL, milligrams per deciliter of serum.
No significant differences were noted in values between pre- and post-intervention or between the two arms of the study.
Analyzed by paired t-test using SPSS software version 27.
Table 4. Markers of gut translocation and inflammation (N = 10)

I-FABP, intestinal fatty acid binding protein; sCD-14, a protein often consistent with circulating lipopolysaccharides; LBP, lipopolysaccharide binding protein.
* Mean differences in data between study start and study end for participants in the glucose and fructose arms of the study.
** P-values of data comparing differences from study start and end in the glucose versus fructose arms of the study.
These data all were within the normal range.
Fecal microbiota and metabolome
Fecal samples were analyzed for microbial composition before and after each study arm using 16S rRNA marker gene sequencing to quantify the relative abundance of bacterial taxa, which yielded a median of 63,207 and a minimum of 39,480 reads per sample. Analysis of 16S marker gene data showed no differences between groups before each treatment in alpha diversity, UniFrac distance, or taxonomic abundance in the cross-over study (Supplemental Fig. 1). The gut microbiota exhibited no differential response to glucose or fructose treatment in alpha diversity (Fig. 3(A)), as measured by richness (P = 0.32), Shannon-diversity (P = 0.43), and Faith’s phylogenetic diversity (P = 0.47). Beta diversity also was not different between the treatments (Fig. 3(B)), based on unweighted UniFrac (P = 0.99) and weighted UniFrac distances (P = 1.0). Differences in taxon relative abundance were sought from all genus-level bacterial taxa with a mean abundance of more than 1% (Fig. 3(C)). Based on a mixed-effects model of logit-transformed relative abundance, Bifidobacterium decreased after treatment in both groups (P = 0.004) but did not differ between treatments (P = 0.89). Bifidobacterium was the only taxon that showed a statistically significant change in abundance after correction for multiple comparisons. In summary, we observed no microbiota associations that differed between treatments.

Fig. 3. Isocaloric fructose administration preserves fecal microbiome diversity. (A) alpha diversity (richness calculated at a rarefaction level of 1000 Operational Taxonomic Units (OTUs), (B) beta diversity (unweighted and weighted Unifrac distance), individual subjects shown by different colors on the right, and (C) changes in abundance of specific taxa (outcome variable of logit-transformed abundance based on a mixed-effects model of the predictive variables: type of sugar and pre/post treatment).
Specific analysis of Akkermansia muciniphila and Lactobacillus johnsonii, which previously had changed with fructose treatment in mice [Reference Volynets, Louis and Pretz26], was performed by aligning the unique 16S rRNA amplicon sequence variants in our dataset to the 16S rRNA gene sequence of the type strains for each species to identify their closest representative. No differences in relative abundance for Akkermansia muciniphilia and Lactobacillus johnsonii, using a mixed-effects model were found.
Fecal metabolome
Overall, few significant metabolite differences were observed in the fructose versus glucose arm following correction for multiple comparisons. To account for inter-personal variation pairwise analysis of data from each subject was performed before and after each treatment arm showing significantly lower levels of fecal glucose and fructose 6-phosphate after fructose supplementation and higher levels of niacinamide and guanine (Supplemental Fig. 2).
Fecal fructose
Fecal fructose content showed wide variation between subjects. The study-end fecal specimens contained 36% higher relative peak intensities than the baseline specimens in the fructose arm of the study, whereas fecal fructose fell by 16% in the glucose arm of the study (Supplemental Fig. 3).
Plasma metabolome
To gain further insight into the relationship of fecal microbiota, metabolites, and changes in intestinal permeability, plasma metabolites were measured at baseline, and study end for each intervention arm. Overall, 115 metabolites were detected in at least one sample, and 66 of these metabolites were detected in all 40 samples. These data were evaluated by principal components analysis, unsupervised hierarchical clustering, and volcano plots and few significant (P < 0.05) differences were observed using the groupwise statistical comparisons. Similarly, the overall metabolite profile was unable to distinguish the four groups, with all samples appearing equally dispersed. Pairwise analysis using the DESeq2 genomics library [Reference Love, Huber and Anders30] to model subject covariate and calculate a corrected P-value showed no individual metabolite significantly differed in the glucose arms but methionine increased by ˜7% (P-adj = 0.035) and ornithine decreased by 1.8-fold (P-adj = 0.035) in the fructose arm.
Intestinal permeability studies
To study the effects of fructose compared to glucose feeding on gut permeability, a four sugar solution was administered before and after the 14-day sugar supplemental diets. No significant differences in urinary excretion of the test sugars used to detect increased permeability in the stomach, small, or large intestine were observed (Fig. 4). We then conducted a cross-over design analysis to assess both the diet differences and the timing of the fructose or glucose arm. Again, no significant differences were detected in these test sugars (P > 0.05). Since residual analysis showed potential outliers, we repeated analysis after removing outliers, but differences in diets or timing remained insignificant. Based on the data from our 10 subjects, we would need to recruit 36–182 participants to find a significant difference in urinary sugar excretion between the fructose and glucose arms (Supplemental Materials and Methods).

Fig. 4. Intestinal permeability is unchanged by fructose administration. Quantification of intestinal permeability by ultra-performance liquid chromatography mass spectrometry. Analysis of changes in concentration of sucrose, sucralose, lactulose, and mannitol in urine. Cross-over design analysis of both diet and timing of the fructose or glucose arm of the study.
Discussion
The present pilot study aimed to determine the effects of excess fructose compared to isocaloric glucose ingestion on the fecal microbiome, metabolome, intestinal permeability, and markers of endotoxemia in individuals with obesity in a rigorous cross-over study conducted in an inpatient metabolic unit. Since bacterial fructose metabolism likely impacts colonic homeostasis, we needed to provide enough dietary fructose to ensure that significant amounts of the sugar entered the colon from the small intestine. This goal was achieved as the fecal content of fructose increased by 36% during fructose supplemental feeding but fell by 16% during the glucose supplemental diet. Since fructose is rapidly metabolized by colonic bacteria, the finding of excess fructose in the feces indicated that considerable fructose passed into the colon. The dietary regimen used achieved a fructose intake of as much as a mean of 20.1 % of total calorie intake. The average fructose consumption in the US population has been calculated as about 10% of calories [Reference Vos, Kimmons, Gillespie, Welsh and Blanck31], noting that frequent consumption of sugary drinks can add 9% or more of fructose as percent of calories [Reference Nielsen and Popkin32], a total figure that approaches amounts used in our study. We also examined changes in fecal microbiome distribution and function following the fructose–glucose regimens. Switching subjects from their normal diet to a standard metabolic study diet would be expected to rapidly change gut microbiota [Reference David, Maurice and Carmody18]. To eliminate such effects, we determined and mimicked details of each subject’s “usual” diet as we have previously reported [Reference Bokulich, Battaglia, Aleman, Walker, Blaser and Holt33], modifying these diets only by reducing complex carbohydrates for the fructose or glucose supplemental drinks to maintain macronutrient stability. These goals were achieved since the planned study diets and the macronutrient content consumed by subjects during the study was very close to their “usual” diets.
Neither fructose nor glucose supplementation significantly changed fasting routine laboratory blood tests, liver function tests, or lipid and uric acid levels taken 10–12 h after the last meal. Changes in postprandial lipids are well known to occur after test meals with high fructose content [Reference Stanhope and Havel34], but fasting levels usually are unchanged [Reference Dolan, Potter and Burdock35]. Furthermore, no differences in abdominal symptoms between the two study periods occurred. Pronounced abdominal symptoms occur following ingestion of a bolus of fructose [Reference Ravich, Bayless and Thomas36], but the co-presence of dietary glucose increases small intestinal fructose absorption [Reference Gray and Ingelfinger37] reducing rapid passage of unabsorbed fructose into the colon. Despite this, a considerable amount of fructose must have entered the colon to be metabolized by gut bacteria as fecal fructose increased during fructose supplementation. Overall, feeding neither the fructose nor glucose supplemented diets for 14 days significantly changed fecal microbial alpha or beta diversity and using untargeted microbial analysis, no significant differences were detected in individual taxa. These data contrast with those described in several studies in the mouse [Reference Volynets, Louis and Pretz26,Reference Ferraris, Choe and Patel38].
Furthermore, Akkermansia muciniphilia and Lactobacillus johnsonii are significantly altered by fructose administration in mice but were not significantly changed by either fructose or glucose feeding in our human subjects. Our data supports others that demonstrates fecal microbiome studies in mice often are not reproduced in human studies [Reference Wan, Wang and Yuan39]. We provided our human subjects a mean of 20.1% of total calories as fructose, not significantly less than the 30% fed to rodents in several studies [Reference DiLucca, Crecenzo and Mazzoli19, Reference Volynets, Louis and Pretz26], or to monkeys [Reference Kavanagh, Wylie and Tucker10]. Other investigators gave rodents as much as 50% or more fructose in the diet [Reference Kawabata, Kanmura and Morinaga20].
Although we detected no significant effects on fecal microbiota distribution and individual taxa, these bacteria might have changed their metabolic activity. However, few changes in fecal metabolites were found in our study. In the fructose arm, there were significant reductions in fecal glucose and fructose 6-phosphate levels, presumably representing carbohydrate metabolic re-programming of the existing microbiota. Higher levels of nicotinamide and guanine after fructose were found in the feces. Dietary and bacterial tryptophan are metabolized to niacin by many organisms [Reference Yoshii, Hosomi, Sawane and Kunisawa40] which also can synthesize purine nucleotides. In contrast to our human studies, fructose supplemented diets in rodents show changes in gut microbiota [Reference Volynets, Louis and Pretz26,Reference Do, Lee, Oh, Kim and Park41], and evidence of colonic inflammation, increased intestinal permeability [Reference Rahman, Desai and Iyer42], and endotoxemia, together with changes in gut tight junction enzymes [Reference Sellmann, Priebs and Landmann43]. Our studies showed no evidence of gut damage as judged by fecal calprotectin and circulating intestinal fatty acid binding protein concentrations. Direct measures of intestinal permeability and markers of endotoxemia also were unaltered by either fructose or glucose supplemental feeding.
Preclinical studies that show the pronounced changes that excess fructose feeding induces in the gut would imply that they have a role in the initiation or progression of NAFLD. It has been shown that some NAFLD patients may harbor alcohol-producing microbiota in their gut [Reference Zhu, Baker and Gill44] and that probiotics may improve liver function, however, usually associated with some weight loss [Reference Sharpton, Maraj, Harding-Theobald, Vittinghoff and Terrault45]. Rifaxamin treatment not only improved liver enzyme and endotoxin concentrations in patients with NAFLD but also lowered body weight [Reference Gangarapura, Ince and Baysal46]. Some children with NAFLD or NASH have a distinctive gut microbiome [Reference Schwimmer, Johnson and Angeles47], but the human response to excess fructose is very heterogeneous due to differences in liver metabolism [Reference Esler and Bence12]. This might also occur because of varying rates of fructose absorption [Reference Jones, Butler and Brooks16] or differences in fructose microbial metabolism.
We found no detailed studies in the literature on effects of fructose administration on the human plasma metabolome. One study reported that fructose feeding increased plasma uridine [Reference Yamamoto, Moriwaki, Takahashi, Tsutsumi, Yamakita and Higashino48] and another investigator [Reference Gonzales-Granera, Damns-Machado, Basrai and Bischoff49] showed fructose fed as high fructose syrup increased plasma lysopholipids and decreased mean acylcarnitine levels suggestive of increased lipid oxidation. Our study showed that fructose compared to glucose administration led to a modest 7% increase in methionine and a 1.8-fold reduction in plasma ornithine concentration which are unlikely to contribute to the ill effects of excess fructose consumption.
A study strength was the cross-over design in a controlled metabolic unit directly comparing equal amounts of fructose or glucose provided in the context of participants’ “usual” diet. The mean of 20.1% of total energy intake as fructose exceeds the maximum consumed by humans and was sufficient to increase fructose in the feces implying that considerable fructose reached the colon. A further strength was the simultaneous measures of the fecal microbiome, fecal, and plasma metabolites, gut permeability, and markers of endotoxemia, gut damage, and inflammation.
A potential weakness was limiting fructose or glucose supplementation to only 14 days, determined in part by practical considerations of this hospital inpatient study. Available data show that the fecal microbiome (and probably fecal metabolites) can change well within 14 days of initiating a change of diet [Reference David, Maurice and Carmody18,Reference Wu, Chen and Panyod50]. Direct-acting damaging agents cause gut damage within a few days, but the speed of changing gut permeability is unknown. Although the number of participants was small, the cross-over design enhanced statistical power. As many as 182 subjects would need to be recruited to be able to detect a significant difference between the fructose and glucose study arms of a cross-over study which was not feasible in terms of logistics and cost given the multiple omic endpoints studied in each subject. Fructose and isocaloric glucose supplementation could be extended longer than 14 days, but this would likely require conducting such studies under free-living conditions with additional confounding factors.
In summary, in a controlled metabolic unit cross-over pilot study of equal amounts of fructose or glucose for 14 days, keeping caloric intake stable, excess fructose did not induce significant changes in the fecal microbiome, fecal or plasma metabolites, gut permeability, markers of endotoxemia, gut damage, or inflammation. If excess fructose consumption leads to no changes in gut microbiota or permeability, then therapeutic measures that are aimed to change the gut microbiome to improve the treatment of NAFLD may not be beneficial.
Supplementary Material
To view supplementary material for this article, please visit https://doi.org/10.1017/cts.2021.801.
Acknowledgements
We thank volunteers for willingly participating in the study as part of the research team, the research nurses for their professional conduct of study and support of our volunteers, and nutrition staff for support and assurance of dietary compliance. We thank Andrew Dannenberg and David Montrose for providing background data of their work for our application for grant support, and Ariel Myatt for help with preparation of manuscript and figures.
This research was supported in part by Rockefeller University Center for Clinical and Translational Science (RUCCTS) grant # UL1TR001866 from the National Center for Advancing Translational Sciences (NCATS), National Institutes of Health (NIH), Clinical and Translational Science Award (CTSA) Program, The Sackler Center for Biomedicine and Nutrition at the Rockefeller University 1ZIANR000018, Division of Intramural Research, NIH, Department of Health and Human Services, and American Heart Association 17-SFRN33520045 K08-DK117064, NIH, at New York University Langone Health. The funders had no role in study design, data collection and analysis, decision to publish, or preparation of the manuscript.
Disclosures
The authors have no conflicts of interest to declare.
Data Availability Statement
All individual participant data collected during the trial, after de-identification, including text, tables, figures, and supplemental material, will be available after publication to anyone who wishes to access the data. Data are available upon reasonable request to:
Dr Peter R. Holt, holtp@rockefeller.edu. ORCID identifier 0000–0002–8469–2766 or
Dr. Jose O. Aleman, Jose.Aleman@nyulangone.org. ORCID identifier 0000–0003–3753–6717.
ClinicalTrials.gov Identifier, NCT03339245.