Introduction
Arctic sea-ice extent and thickness has declined at an accelerating rate over the past several decades, responding to and amplifying rapid Arctic warming (Reference Hartmann and StockerHartmann and others, 2013) through ice–albedo feedbacks (Reference Serreze, Barrett, Slater, Steele, Zhang and TrenberthSerreze and others, 2007; Reference Perovich and Richter-MengePerovich and Richter-Menge, 2009; Reference Cavalieri and ParkinsonCavalieri and Parkinson, 2012). Sea-ice loss has strongly modified the climate, ocean circulation, storm tracks and ecology of the Arctic (Reference Dieckmann and HellmerDieckmann and Hellmer, 2010), but our understanding of sea-ice extent and variability prior to the 20th century is limited (Reference Perovich and Richter-MengePerovich and Richter-Menge, 2009; Reference Kinnard, Zdanowicz, Fisher, Isaksson, de Vernal and ThompsonKinnard and others, 2011; Reference Abram, Wolff and CurranAbram and others, 2013). The Greenland ice sheet (GIS) has likewise responded to rising summertime temperatures with increases in the extent of surface melt (Reference Hall, Comiso, DiGirolamo, Shuman, Box and KoenigHall and others, 2013) and increasing rates of mass loss (Reference AlleyAlley and others, 2010; Reference ShepherdShepherd and others, 2012). In northwest Greenland, mass loss accelerated in 2005 due to higher surface melt and runoff and increasing ice discharge, despite higher annual snowfall rates (Reference SasgenSasgen and others, 2012; Reference HawleyHawley and others, 2014). Given the projections of 5–15 cm of sea-level rise by 2100 from GIS mass loss alone under SRES (Intergovernmental Panel on Climate Change (IPCC) Special Report on Emissions Scenarios) A1B (Reference Yoshimori and Abe-OuchiYoshimori and Abe-Ouchi, 2012), it is critical to understand both how the climate around Greenland changed in the past and how the ice sheet responded to those changes, especially during past warm periods like the Medieval Climate Anomaly and earlier Holocene Climatic Optimum (e.g. Reference VintherVinther and others, 2009).
Ice cores from Greenland provide high-resolution (seasonally to annually resolved) proxy records of past temperature, accumulation and atmospheric circulation. Most Greenland ice cores have been collected from the dry snow zone in the high (>2500 m elevation) inland part of the ice sheet, producing invaluable records of North Atlantic and Northern Hemisphere climate and environmental change spanning the past ∼120 000 years (Reference MayewskiMayewski and others, 1997; Reference AndersenAndersen and others, 2004; Reference Masson-DelmotteMasson-Delmotte and others, 2005). In contrast, coastal ice cores provide the opportunity to develop more regional-scale records of climate conditions along the climatologically sensitive and dynamic ice-sheet margins, as well as robust records of past sea-ice variability due to the proximity and greater influence of neighboring marine areas (Reference Isaksson, Kekonen, Moore and MulvaneyIsaksson and others, 2005; Reference Abram, Wolff and CurranAbram and others, 2013; Reference Sinclair, Bertler, Bowen and ArrigoSinclair and others, 2014).
The ice-sheet margins also preserve valuable landscape records of past ice-sheet extent (Reference Levy, Kelly, Howley and VirginiaLevy and others, 2012) and lake sediment records of past summertime temperature (Reference Axford, Losee, Briner, Francis, Langdon and WalkerAxford and others, 2013). Combining these records with a coastal ice core influenced by the same ice-marginal conditions presents the opportunity for multi-proxy reconstructions of past temperature and ice-sheet extent. Temperature and precipitation changes around the Greenland coast show pronounced regional variability and often opposing anomaly patterns due to the strong influence of atmospheric circulation patterns like the North Atlantic Oscillation (NAO), a ‘seesaw’ of atmospheric mass with centers of action in the Icelandic low and Azores high that is most active in the wintertime (Reference HurrellHurrell, 1995; Reference HannaHanna and others, 2013). NAO positive conditions are associated with a stronger Icelandic-low–Azores-high pressure gradient, with anomalously strong (weak) southerly flow to the east (west) of Greenland, resulting in warmer and wetter conditions in northeast Greenland and cooler and dryer conditions in northwest Greenland (Reference HurrellHurrell, 1995; Reference HannaHanna and others, 2013; Reference Mernild, Hanna, Yde, Cappelen and MalmrosMernild and others, 2014). Glacier surface mass balance responds to these regional temperature and precipitation anomalies (Reference SasgenSasgen and others, 2012), so it is critical to collect coastal ice cores close to the ice margins of interest.
Here we describe temperature and sea-ice proxy records from the coastal 2Barrel ice core, collected from a site ∼100 km from the northwest Greenland coast (Fig. 1). This is a 21 m long reconnaissance ice core spanning 1990–2010, collected to evaluate the suitability of this site for the recovery of a deeper, Holocene-length ice core. The core site is close enough to the coast to be strongly influenced by regional conditions in northern Baffin Bay (a significant local moisture source), but high enough on the ice sheet that surface melt does not significantly alter the ice chemistry record. We focus here on temperature and sea-ice concentrations because these climate parameters have changed dramatically in northwest Greenland since 1990 and would be valuable proxies in a Holocene-length record. The instrumental record from Thule shows a 1.5°C increase in annual temperatures since 1952, and a faster 3.3°C increase since 1991 with a summer and winter warming of 3.4°C and 5.4°C, respectively (Reference Wong, Osterberg, Hawley, Courville, Ferris and HowleyWong and others, 2015). This regional increase in temperature has driven the loss of sea ice in Baffin Bay during all seasons since at least the 1950s, with the largest sea-ice concentration declines in summer (−4.2% decade−1) and fall (−5.2% decade−1) from 1990 to 2012 (Reference RaynerRayner and others, 2003).

Fig. 1. Maps of Greenland (a) and the northern Baffin Bay region (b), showing locations discussed in the text including the 2Barrel core location (star), 100 km from the Thule coast. TAB: Thule Air Base; CC: Camp Century; NIC: North Ice Cap; NOW: North Water Polynya; SS: Smith Sound; EI: Ellesmere Island; DI: Devon Island. Also shown in (a) is the 1979–2012 average March sea-ice boundary (maximum annual extent) (Reference RaynerRayner and others, 2003). Baffin Bay south of Smith Sound is typically sea-ice free during the September minimum extent.
The mean annual temperature of the 2Barrel site is estimated to be −22.5°C based on nearby 10 m firn temperatures (Reference BensonBenson, 1962; Reference PolashenskiPolashenski and others, 2014) and extrapolation from the −10.5°C mean annual temperature at Thule Air Base (1991–2012) using a 7.5°C km−1 lapse rate (Reference Hall, Comiso, DiGirolamo, Shuman, Box and KoenigHall and others, 2013). This is also consistent with limited data from the Greenland Climate Network (GC-Net) automatic weather station, GITS, at Camp Century (Fig. 1), located 50 km to the east at 1869 m elevation on the ice sheet, which recorded mean annual temperatures of −23.4°C from 2001 to 2002 and −22.7°C from 2006 to 2007 (Reference Steffen and BoxSteffen and Box, 2001). Hourly temperatures at GITS range from maximums of +1°C in summer to minimums of −53°C in winter (Reference Steffen and BoxSteffen and Box, 2001). In addition to the significant negative correlation between Thule temperatures and the NAO described above (NAO+ conditions associated with anomalously cool Thule temperatures), northwest Greenland temperature is also significantly influenced by the Atlantic multi-decadal oscillation (AMO). The AMO is the dominant multi-decadal mode of North Atlantic sea surface temperature variability, hypothesized to result from changes in Atlantic meridional overturning circulation strength (Reference Schlesinger and RamankuttySchlesinger and Ramankutty, 1994). AMO positive conditions are associated with anomalously high summertime temperatures in northwest Greenland and throughout most of the North Atlantic (Reference HannaHanna and others, 2013).
Annual snow accumulation at 2Barrel averaged 0.51 m w.e. (range 0.27–0.89 m w.e.) from 1990 to 2010, and the accumulation time series is significantly correlated to the instrumental Thule precipitation record (r = 0.63, p < 0.01), with both records indicating a precipitation increase since 1990 (Reference HawleyHawley and others, 2014; Reference Wong, Osterberg, Hawley, Courville, Ferris and HowleyWong and others, 2015). Measured daily precipitation at Thule from 1952 to 2012 has a strong summer–fall bias, with 64% of annual precipitation (rain and snow) falling from June to November (Reference Wong, Osterberg, Hawley, Courville, Ferris and HowleyWong and others, 2015). However, GITS data show a more even distribution of snow accumulation throughout the year, with 36% accumulating in summer, 31% in fall and 17% in each of winter and spring (Reference Steffen and BoxSteffen and Box, 2001). Our analysis of daily precipitation data indicates that precipitation at Thule and GITS (and therefore 2Barrel) typically falls during times of southerly and south-westerly flow originating over Baffin Bay, accentuated by ice-sheet orographic forcing.
The dynamics of northern Baffin Bay sea ice are influenced by the North Water (NOW) Polynya, a predominantly latent-heat polynya (Reference Melling, Gratton and IngramMelling and others, 2001) bounded by Ellesmere and Devon Islands in the west and the Thule region of Greenland in the east from 75° N to 78.5° N (Fig. 1b) (Reference VidussiVidussi and others, 2004). Pack ice flows southward through the narrow Nares Strait due to northerly winds and currents, forming an ice bridge in northern Smith Sound that blocks ice from moving into Baffin Bay (Reference Barber, Hanesiak, Chan and PiwowarBarber and others, 2001). The polynya is 95% covered by ice in January, but starts to open up in April and reaches its maximum extent in July (Reference VidussiVidussi and others, 2004). The NOW Polynya is the most biologically productive region of the Canadian Arctic (Reference StirlingStirling, 1997), with phytoplankton blooms typically peaking in May and June as ice cover retreats (Reference MeiMei and others, 2003). Phytoplankton and ice algae blooms emit dimethyl sulfide (DMS) to the atmosphere through the degradation of dimethylsulfoniopropionate, which regulates phytoplankton osmotic pressure (Reference Burgermeister and GeorgiiBurgermeister and Georgii, 1991). DMS is subsequently oxidized in the atmosphere to methanesulfonic acid (MSA), which is deposited in precipitation on the GIS with a spring–summer maximum reflecting peak marine production during sea-ice break-up (Reference Jaffrezo, Davidson, Legrand and DibbJaffrezo and others, 1994; Reference LegrandLegrand and others, 1997; Reference SharmaSharma and others, 2012). Marine primary production is the sole source of Arctic MSA (Reference Mulvaney, Pasteur, Peel, Saltzman and WhungMulvaney and others, 1992), and we use MSA here to develop proxy records for Baffin Bay sea-ice concentration and temperature.
We also focus on the 2Barrel record of deuterium excess (d-excess), defined as the isotopic deviation from the meteoric waterline: d-excess = δD − 8δ18O (Reference CraigCraig, 1961), where δD and δ18O are the parts per thousand deviation of deuterium/hydrogen and 18O/16O atomic ratios, respectively, from those of Standard Mean Ocean Water. Deuterium excess is primarily sensitive to the sea surface temperature and relative humidity of the moisture source. Vapor that evaporates from cold, polar surface waters with high relative humidity is associated with low d-excess, while warmer subtropical moisture sources with low relative humidity are associated with high d-excess vapor (Reference Merlivat and JouzelMerlivat and Jouzel, 1979; Reference Petit, White, Young, Jouzel and KorotkevichPetit and others, 1991). Thus, changes in the temperature or relative humidity of the moisture source, or changes in the relative proportion of subtropical and polar moisture sources, will lead to changes in d-excess at an ice-core site through time (Reference Vimeux, Masson, Jouzel, Stievenard and PetitVimeux and others, 1999; Reference Masson-DelmotteMasson-Delmotte and others, 2005). We describe robust relationships between d-excess in the 2Barrel ice core and Baffin Bay temperature and sea-ice concentrations.
Methods
2Barrel ice-core collection
The 2Barrel ice core (76.935° N, 63.153° W) was collected from a site ∼100 km inland from the northwest Greenland coast at an elevation of 1685 m on the ice sheet (Fig. 1). The core was drilled to 21.3 m depth in July 2011 using a Kovacs Enterprise Mark III (7.25 cm diameter) coring system (Reference HawleyHawley and others, 2014). It was shipped frozen to the Dartmouth Ice Core Laboratory, Hanover, NH, where it was processed, logged, and analyzed for glaciochemistry. The top 1 m of the snowpack was sampled in a snow pit directly above the drilling site at 10 cm resolution with pre-cleaned low-density polyethylene bottles by researchers wearing non-particulating Tyvek suits and vinyl gloves (cf. Reference Osterberg, Handley, Sneed, Mayewski and KreutzOsterberg and others, 2006). The core site is within the percolation zone with summer surface melting, but refrozen melt layers represent <3% of the core length.
Glaciochemical analyses and timescale development
The ice core was sampled for major-ion, trace-element and stable isotope analyses at 4–10 cm resolution using a continuous ice-core melter system with discrete sampling (Reference Osterberg, Handley, Sneed, Mayewski and KreutzOsterberg and others, 2006). A total of 461 samples were analyzed for an average of 20 samples a−1. Based on the results of Reference Koffman, Handley, Osterberg, Wells and KreutzKoffman and others (2014), ice-core and snow-pit samples for trace-element analyses were acidified with Optima™ nitric acid to 3% concentration under a HEPA clean bench, and allowed to react with the acid at room temperature for 60 days prior to analyses. We measured stable water isotope ratios (δD and δ18O), from which we calculate d-excess, using a Picarro L2130-i cavity ringdown analyzer with a typical uncertainty of ±0.6‰ for δD, ±0.2‰ for δ18O and ±0.45‰ for d-excess. Major cation and trace-element sample concentrations (Na, Mg, Ca, Al, Fe, S, K, Li, Ti, Sr, Cd, Cs, Ba, Pb, Bi, U, As, V, Cr, Mn, Co, Cu, Zn, REEs (rare-earth elements)) were measured by inductively coupled plasma mass spectrometry using a Thermo Element2 at the Climate Change Institute, University of Maine, Orono, USA, as detailed in Reference Osterberg, Handley, Sneed, Mayewski and KreutzOsterberg and others (2006). Anion concentrations (SO4 2−, NO3 −, Cl− and MSA) were measured using a Dionex ICS 5000 capillary ion chromatography system at Dartmouth with a typical uncertainty of 1.0 ppb.
The 2Barrel ice core was annually dated from 1990 to 2010 using robust summertime peaks in MSA, δD and δ18O (Fig. 2). The summertime MSA peaks are consistent with its source from marine phytoplankton blooms in Baffin Bay. We estimate a timescale uncertainty of ±0.5 years based on repeat independent annual-layer counts. Thus, we do not attempt here to evaluate seasonal ice-core chemistry averages, but rather compare annually averaged ice-core data to seasonal and monthly climate parameters. We annually average the ice-core data based on approximating a 1 January date corresponding with MSA, δD and δ18O minima (Fig. 2), so our annual averaging approximates a calendar year (January–December).

Fig. 2. Stable water isotope (δ18O; black line) ratios and MSA (gray line) concentrations in the 2Barrel ice core, used to develop the annual timescale. Note the consistent summer seasonal peaks in both records, representing summer temperatures (δ18O) and spring–summer emissions from phytoplankton in Baffin Bay. Data average 20 samples per year, and are displayed with a five-point smoothing.
Meteorological and climate reanalysis data
Herein we develop 2Barrel ice-core proxy records for Baffin Bay sea-ice concentrations using sea-ice data from the ERA-Interim reanalysis model developed by the European Centre for Medium-Range Weather Forecasts (Reference DeeDee and others, 2011), averaged over the region 70–78° N, 55–80° W. We also develop regional temperature proxies from 2Barrel data using a composite record of temperature for the nearby Thule region developed by Reference Wong, Osterberg, Hawley, Courville, Ferris and HowleyWong and others (2015). This record is based on Danish Meteorological Institute (DMI) stations at Dundas (04200) and Pituffik (04202) (Reference CappelenCappelen, 2013) with missing DMI data replaced with observations from the weather center at Thule Air Base. The resulting daily composite temperature record has no gaps from 1952 to 2012.
Results
2Barrel sea-ice proxies
The 2Barrel ice-core annually averaged MSA concentrations are significantly correlated with Baffin Bay sea-ice concentrations in May (r = −0.43, p < 0.05) and June (r = −0.53, p < 0.01). The negative correlations indicate that years with lower May–June sea-ice concentrations are associated with higher ice-core MSA concentrations. No other month in the sea-ice concentration dataset has a statistically significant correlation with 2Barrel MSA. The strengths of the May and June correlations increase to −0.46 (p < 0.05) and −0.59 (p < 0.01), respectively, if the sea ice is averaged over a smaller region in northern Baffin Bay (72–76° N; Fig. 3). This is reflected in the spatial correlation plot shown in Figure 4, where the strongest correlations with the 2Barrel MSA record are located along the retreating sea-ice edge of northern Baffin Bay and in the NOW Polynya region.
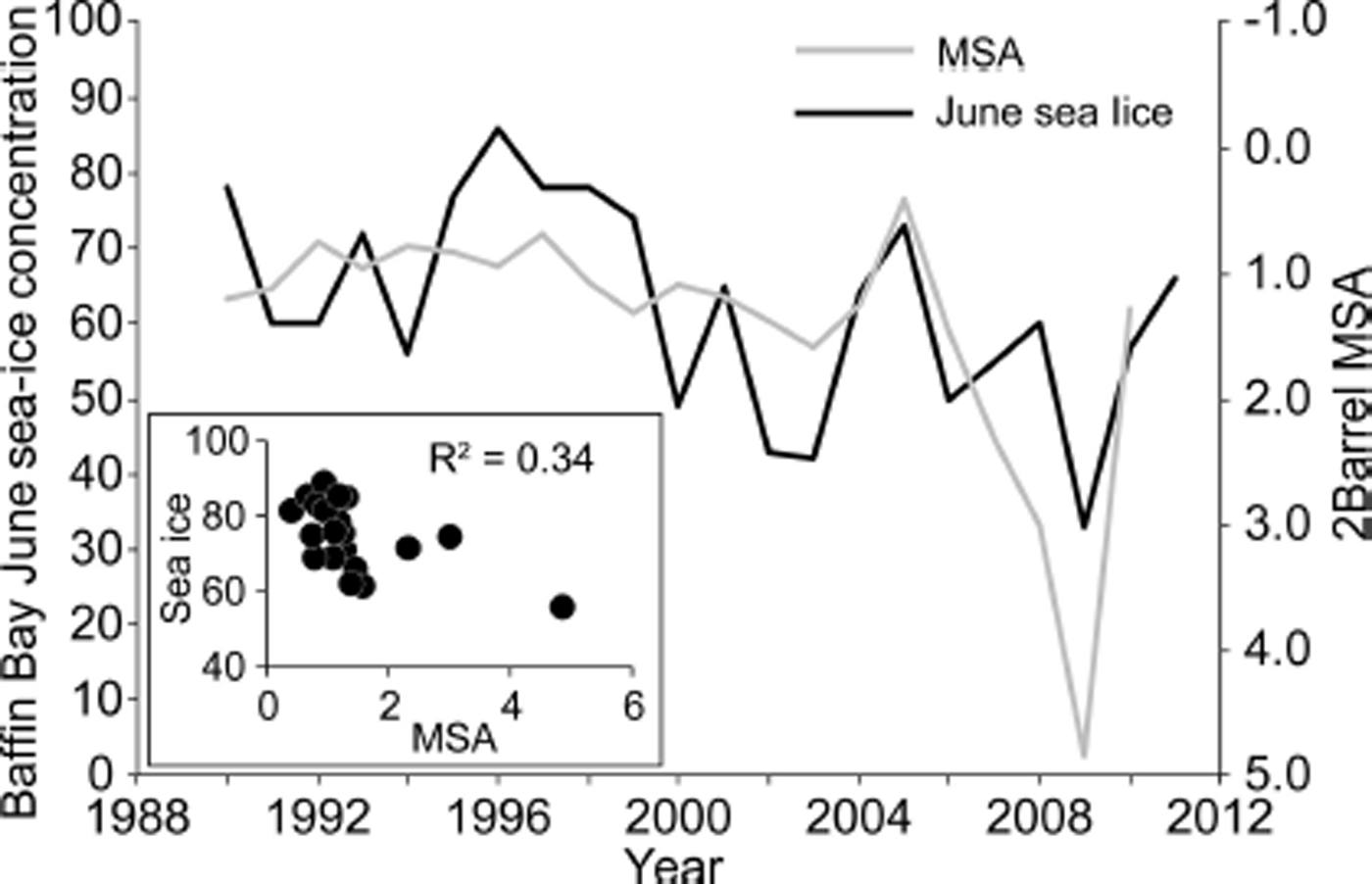
Fig. 3. Annually averaged MSA (gray line) compared to June Baffin Bay sea-ice concentration (black line) from 1990 to 2010. The negative correlation is interpreted to represent increased phytoplankton production and DMS emissions during years with lower Baffin Bay sea-ice concentration.

Fig. 4. Spatial correlation map of 2Barrel MSA compared to ERA-Interim sea-ice concentration in May (left) and June (right) from 1990 to 2010. The locations of strongest negative correlations in northern Baffin Bay and the North Water Polynya region are indicative of the location of the retreating ice front during these months. An earlier sea-ice retreat is associated with higher MSA concentrations in the 2Barrel ice core, interpreted to result from higher phytoplankton productivity. Only gridcells with significant correlations (|r| > 0.36, p < 0.05) are shaded. The polygon demarks the region over which Baffin Bay sea-ice extent is averaged for the majority of correlation analyses in the text.
The 2Barrel d-excess record is strongly correlated (r = 0.62, p < 0.005) with the annually averaged (January–December) Baffin Bay sea-ice concentration. Figure 5 displays the strong positive relationship between the two time series from 1990 to 2010, with the trend of declining Baffin Bay sea-ice concentration associated with progressively lower d-excess values. Figure 6 shows the spatial pattern of the d-excess–sea-ice correlation, with statistically significant positive correlations across the northern half of Baffin Bay. On a monthly basis, 2Barrel d-excess is most strongly correlated with June (r = 0.61, p < 0.005), October (r = 0.56, p < 0.01) and November (r = 0.55, p < 0.01) sea-ice concentrations. In general, the larger the decline in sea-ice concentration over the 1990–2010 period, the stronger the correlation with 2Barrel d-excess (Table 1). June and November are associated with the fastest Baffin Bay sea-ice retreat rates over the 1990–2010 interval, with rates of −8.0% decade−1 and −9.4% decade−1, respectively. October sea-ice concentration has declined 4.1% decade−1 over Baffin Bay, but has a faster decline of 8.7% decade−1 in northern Baffin Bay (75–78° N) closer to Thule. Conversely, August and September sea-ice variability has low (r = 0.01–0.08) and insignificant correlations with d-excess because most of Baffin Bay is consistently ice-free during these months (average concentrations of 5.9–7%).
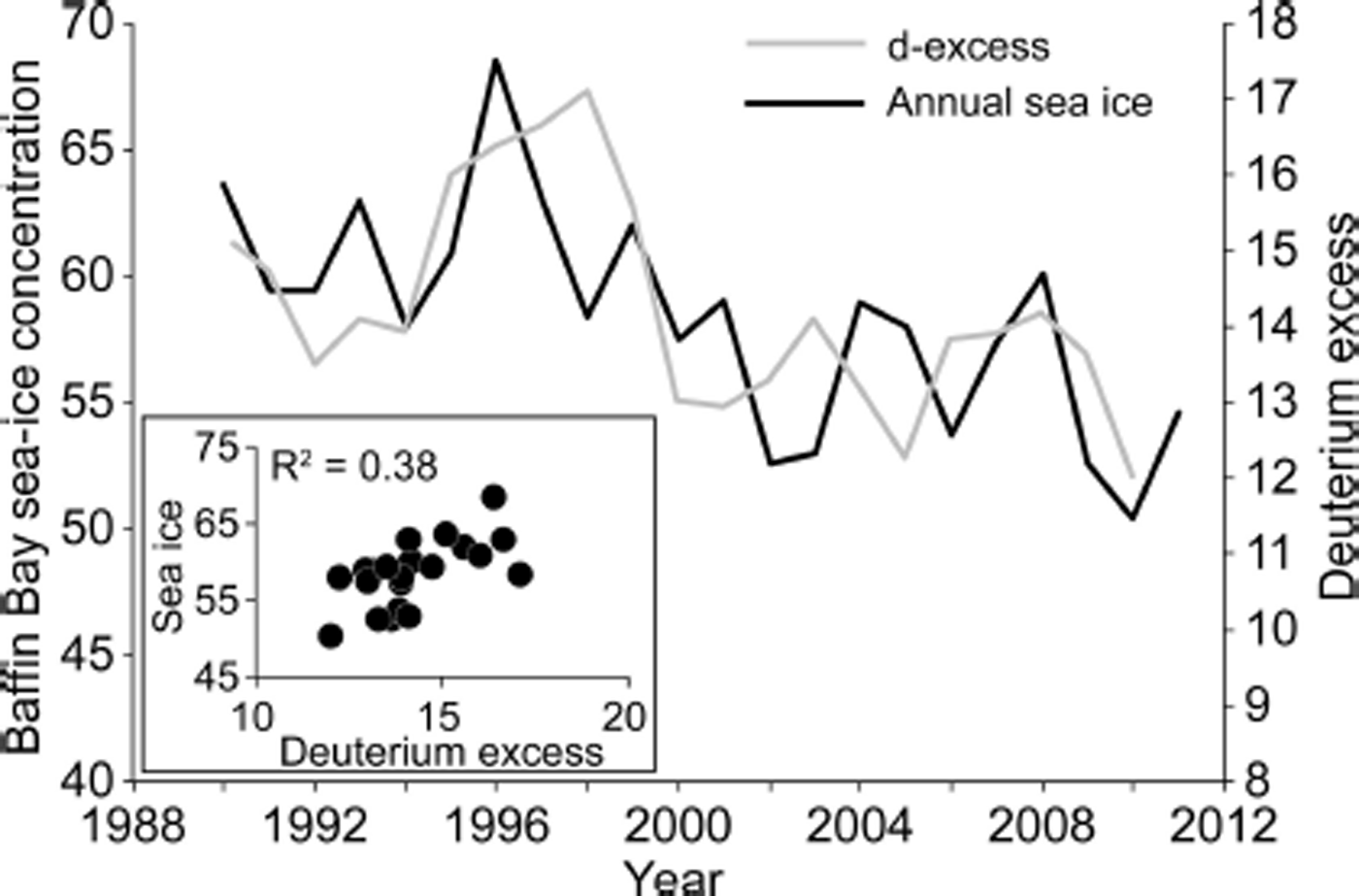
Fig. 5. Annually averaged 2Barrel d-excess (gray) compared to annually averaged Baffin Bay sea-ice concentration (black) from 1990 to 2010. The positive correlation is interpreted to result from an increased proportion of low d-excess Baffin Bay moisture in years with lower sea-ice concentrations.

Fig. 6. Spatial correlation map of 2Barrel d-excess compared to ERA-Interim sea-ice concentration from 1990 to 2010. Sea-ice concentration in each gridcell is correlated against the 2Barrel d-excess record, and the resulting correlation coefficient is shown in color if it is statistically significant (|r| > 0.36, p < 0.05).
Table 1. Pearson correlation between monthly Baffin Bay sea-ice concentration and 2Barrel annual average d-excess, and monthly Baffin Bay sea-ice concentration mean, trend and variance from 1990 to 2010. Months with the strongest correlations are associated with the largest trends and variance

June and November also show the largest year-to-year variance in sea-ice concentrations (Table 1), indicating that these months are the most sensitive to external forces like temperature and storms that can significantly influence sea-ice coverage. This would be expected given that June and November are the months during which sea ice most rapidly melts and expands, on average, during the annual sea-ice cycle in Baffin Bay. Consequently, the sea-ice concentrations in June and November closely track annual sea-ice concentrations, with each representing 76% of the annual sea-ice variance.
Combining the 2Barrel MSA and d-excess annually averaged data produces a multilinear regression model of spring–summer (May–July (MJJ)) Baffin Bay sea-ice concentration that explains 51% of the sea-ice variance (SIMJJ = −4.42 × d-excess − 2.79 × MSA + 22.4; r = 0.72, p < 0.001). Thus, a Holocene-length record from the 2Barrel site would provide valuable information about past summer (51% variance) and annually averaged (38% variance) sea-ice variability in Baffin Bay.
2Barrel temperature proxies
Stable isotope ratios (δ18O and δD) have traditionally been used as temperature proxies in ice cores, but 2Barrel annually averaged δ18O is only weakly correlated with summer (June–August (JJA); r = 0.33, p < 0.1) and fall (September–November (SON); r = 0.31, p < 0.1) temperatures at Thule. However, we find significant 2Barrel temperature proxies through the strong relationship between temperature and sea-ice extent in the instrumental period. Baffin Bay sea-ice concentrations are significantly correlated (p < 0.01) with Thule temperatures from 1979 to 2012 in the winter (r = −0.67), spring (r = −0.51), summer (r = −0.59), fall (r = −0.80) and annually (r = −0.64). It therefore follows that 2Barrel annually averaged MSA (r = 0.57, p < 0.005) and d-excess (r = −0.38, p < 0.05) are likewise significantly correlated with Thule summer temperatures from 1990 to 2010. Higher summertime temperatures are associated with less summer sea ice, higher MSA from marine phytoplankton, and lower d-excess. A multilinear regression model incorporating the 2Barrel MSA and d-excess record explains 42% of the Thule summertime temperature variance (T JJA = −0.26 × d-excess + 0.67 × MSA + 7.1; r = 0.65, p < 0.01). The 2Barrel summer temperature model is significantly correlated with summer temperatures in northern Baffin Bay and adjacent northwest Greenland and eastern Baffin Island coastlines.
Reference Wong, Osterberg, Hawley, Courville, Ferris and HowleyWong and others (2015) show that the 2Barrel snow accumulation record is significantly correlated with the Thule annual precipitation record from 1990 to 2010. 2Barrel accumulation is also significantly correlated with spring (MAM; r = 0.49, p < 0.05), autumn (SON; r = 0.45, p < 0.05), winter (DJF; r = 0.46, p < 0.05) and annual Thule temperatures (r = 0.39, p < 0.05). Thus, by combining the MSA, d-excess and annual 2Barrel snow accumulation in a mulitilinear regression model, we develop a robust proxy for annual Thule temperature (T ann) that explains 49% of the instrumental Thule temperature variance (T ann = −0.22 × d-excess + 0.74 × MSA + 5.17 × Accum − 11.1; r = 0.70, p < 0.01; Fig. 7). This annual temperature regression model is strongly correlated (r > 0.7, p < 0.01) with ERA-Interim annual surface temperatures throughout most of Greenland, Baffin Bay and eastern Arctic Canada (Fig. 8). Note, however, that temperatures on the northeast coast of Greenland and into the Greenland Sea are weakly or not significantly correlated, which is consistent with temperature patterns associated with both the NAO and AMO.

Fig. 7. Summer (top) and annual (bottom) Thule temperatures (black) compared to 2Barrel regression models (gray) using MSA and d-excess (summer), and MSA, d-excess and accumulation (annual).

Fig. 8. Spatial correlation map of the 2Barrel MSA–d-excess–accumulation regression model of annual Thule temperature compared to ERA-Interim annual temperatures. The large area of significant correlation from the eastern Canadian Arctic to East Greenland is indicative of the combined importance of the NAO and AMO for temperature in these areas. Only gridcells with significant correlations (|r| > 0.36, p < 0.05) are shaded.
Discussion
MSA–climate relationships
We find statistically significant negative correlations between May–June sea-ice concentrations and 2Barrel MSA, and suggest that larger open-water areas and a longer ice-free season (associated with lower sea-ice concentration) lead to enhanced phytoplankton production and higher DMS emissions in northern Baffin Bay and the NOW Polynya. Reference SharmaSharma and others (2012) similarly found rising MSA concentrations at Arctic aerosol-monitoring stations (Alert; Barrow; Ny-Ålesund) over the past two decades, attributed to declining sea-ice concentrations. In particular, satellite studies show that the strongest blooms occur along the retreating sea-ice edge (Reference Perrette, Yool, Quartly and PopovaPerrette and others, 2011), consistent with the locations of our May–June MSA–sea-ice correlations (Fig. 6). We hypothesize that the MSA–temperature relationship is similarly mediated by sea-ice concentration, rather than a direct mechanism by which temperature affects productivity or DMS oxidation.
Although MSA has been used extensively as a sea-ice proxy in ice cores from Antarctica, comparatively few records exist for the Arctic (Reference Abram, Wolff and CurranAbram and others, 2013). In records from both the Arctic and Antarctic, the sign of the MSA–sea-ice relationship (positive or negative) varies from site to site and sometimes through time (Reference Abram, Wolff and CurranAbram and others (2013) provide a comprehensive review). Similar to the 2Barrel record, the MSA record from Penny Ice Cap, Baffin Island, in southern Baffin Bay is negatively correlated with Baffin Bay sea-ice extent from 1870 to 1991 (Reference FisherFisher and others, 1998; Reference Kinnard, Zdanowicz, Fisher, Isaksson, de Vernal and ThompsonKinnard and others, 2011). Negative MSA–sea-ice relationships are also documented in near-coastal ice cores collected from the Ross Sea (Reference Rhodes, Bertler, Baker, Sneed, Oerter and ArrigoRhodes and others, 2009, Reference Rhodes2012; Reference Sinclair, Bertler, Bowen and ArrigoSinclair and others, 2014) and Amundsen Sea (Reference CriscitielloCriscitiello and others, 2013) sectors of Antarctica, and at some inland Antarctic locations (Reference Sneed, Mayewski and DixonSneed and others, 2011). These studies all propose a similar dependence of ice-core MSA on the extent and duration of open water and its positive influence on primary production. Interestingly, the Lomonosovfonna (Svalbard) ice-core MSA record also shows a negative MSA–sea-ice correlation from 1920 to 1997, but the correlation is positive prior to 1920 (Reference Isaksson, Kekonen, Moore and MulvaneyIsaksson and others, 2005). The authors attribute the higher MSA before 1920, when sea ice was more extensive (Little Ice Age), to higher productivity from increased vertical stability of Barents Sea surface water (Reference Isaksson, Kekonen, Moore and MulvaneyIsaksson and others, 2005).
Elsewhere, however, the sea-ice–MSA correlation is consistently positive; higher MSA ice-core concentrations are associated with increases in sea-ice extent. For example, ice-core records from the Greenland interior at Summit (Reference LegrandLegrand, 1997), NorthGRIP (Reference AndersenAndersen and others, 2004; Reference Kinnard, Zdanowicz, Fisher, Isaksson, de Vernal and ThompsonKinnard and others, 2011), and at 20D in south-central Greenland (Reference Whung, Saltzman, Spencer, Mayewski and GundestrupWhung and others, 1994) all show a significant declining MSA trend through the 20th century and a positive correlation with sea-ice extent. In Antarctica, ice cores from Law Done (Reference Curran, Van Ommen, Morgan, Phillips and PalmerCurran and others, 2003), South Pole (Reference Meyerson, Mayewski, Kreutz, Meeker, Whitlow and TwicklerMeyerson and others, 2002; Reference Sneed, Mayewski and DixonSneed and others, 2011) and the Ross Sea region (Reference Welch, Mayewski and WhitlowWelch and others, 1993) also show consistent positive MSA–sea-ice correlations. Reference Curran, Van Ommen, Morgan, Phillips and PalmerCurran and others (2003) interpreted the Law Dome record as responding to the size of the seasonal sea-ice zone; a larger winter sea-ice extent is associated with a larger region of sea-ice break-up in the spring–summer. This is consistent with the observations of large blooms along retreating sea-ice edges (Reference Perrette, Yool, Quartly and PopovaPerrette and others, 2011), in that more expansive winter sea ice creates a larger area and duration of retreating sea-ice edges during the subsequent summer (Reference Curran, Van Ommen, Morgan, Phillips and PalmerCurran and others, 2003; Reference Abram, Wolff and CurranAbram and others, 2013).
The records noted above highlight the critical importance of understanding the regional climate and sea-ice dynamics that influence an ice-core site in order to correctly interpret an MSA proxy record. Ice cores from high on the ice sheet receive their moisture from further afield than lower-elevation coastal sites. Interior cores may integrate air masses from a larger region that varies in size and location through time, with changes in atmospheric circulation, complicating interpretations (Reference MayewskiMayewski and others, 1997; Reference Vimeux, Masson, Jouzel, Stievenard and PetitVimeux and others, 1999; Reference Masson-DelmotteMasson-Delmotte and others, 2005). Baffin Bay is restricted in size and experiences near-total ice cover in the winter even under today’s relatively warm climate. Thus, warmer and colder periods during the Holocene will influence the time-integrated area of ice-free water in the spring–fall, but will not significantly change the overall area of Baffin Bay experiencing seasonal sea-ice cover and spring break-up that could lead to positive MSA–sea-ice relationships. We therefore hypothesize that the 2Barrel site is well positioned to maintain a negative MSA–sea-ice relationship back through the Holocene, providing a valuable proxy for Baffin Bay sea-ice concentration. This hypothesis is supported by the consistent negative MSA–sea-ice relationship observed in the Penny Ice Cap core during the large temperature and sea-ice changes over the past 120 years (Reference FisherFisher and others, 1998; Reference Kinnard, Zdanowicz, Fisher, Isaksson, de Vernal and ThompsonKinnard and others, 2011). However, the brevity of the 2Barrel record precludes our ability to directly assess the stationarity of the MSA–sea-ice relationship through time, so non-stationarity must be considered if a longer record is recovered from this site.
Many ice cores also show a significant positive or negative relationship between sea-ice and sea-salt sodium or chloride concentrations (Reference GrumetGrumet and others, 2001; Reference Rankin, Wolff and MartinRankin and others, 2002; Reference Wolff, Rankin and RothlisbergerWolff and others, 2003; Reference Kinnard, Zdanowicz, Fisher and WakeKinnard and others, 2006; Reference Sneed, Mayewski and DixonSneed and others, 2011; Reference CriscitielloCriscitiello and others, 2013; Reference Sinclair, Bertler, Bowen and ArrigoSinclair and others, 2014). We note that the 2Barrel sea-salt sodium and chloride records have weak positive correlations with sea-ice concentrations from August through October (r < 0.35, p < 0.1). The positive correlation and fall bias suggest that larger fall sea-ice extents lead to enhanced frost flower formation on new frazil ice (Reference Rankin, Wolff and MartinRankin and others, 2002), and consequently more sea salt advected to the 2Barrel site. High-salinity snow blowing from the sea-ice surface has also been implicated as a significant source of polar sea-salt aerosol (Reference Yang, Pyle and CoxYang and others, 2008; Reference Levine, Yang, Jones and WolffLevine and others, 2014), which would likewise create a positive correlation between 2Barrel sea-salt and sea-ice concentration. The bursting of white cap bubbles on the open ocean, in proportion to average wind speeds, has also been interpreted as a key sea-salt source at other ice-core sites (Reference Fischer, Siggaard-Andersen, Ruth, Röthlisberger and WolffFischer and others, 2007; Reference Koffman, Handley, Osterberg, Wells and KreutzOsterberg and others, 2014), but would likely result in a negative correlation with sea-ice concentration. It is likely that all these sources contribute in some proportion to 2Barrel sea-salt flux, and consequently sea salt is not as sensitive a sea-ice proxy as MSA or d-excess at the 2Barrel site.
Deuterium-excess–climate relationships
We hypothesize that the positive correlation between Baffin Bay sea-ice concentration and 2Barrel d-excess results from changes in the relative balance of polar versus subtropical moisture sources influencing 2Barrel on a year-by-year basis. In years with a lower (higher) Baffin Bay sea-ice concentration, proportionally more (less) of the moisture reaching 2Barrel is low d-excess vapor sourced from ice-free regions of Baffin Bay. B.G. Kopec and others (unpublished information) find the same relationship between d-excess and Baffin Bay sea-ice extent in precipitation samples collected monthly at Alert, Eureka and Cambridge Bay, Canada, from 1990 to 2012. Using d-excess, they suggest that the proportion of Arctic moisture at these sites increases 13.9 ± 3.9% per 105 km2 of Baffin Bay sea-ice loss (B.G. Kopec and others, unpublished information).
In contrast, Reference Sinclair, Bertler, Bowen and ArrigoSinclair and others (2014) find a negative correlation between sea-ice area and d-excess in the coastal Whitehall Glacier ice core collected in the Ross Sea region of Antarctica. However, this is because higher sea-ice extent is related to stronger southerly winds advecting ice northwards into the Ross Sea. These southerly winds concurrently increase the size of the Ross Sea Polynya, thereby increasing the proportion of low d-excess moisture reaching the nearby Whitehall Glacier ice-core site. Thus, the relationship between d-excess, local moisture proportion and polynya size is the same at 2Barrel and Whitehall Glacier. The contrasting correlations relate, instead, to the opposing relationships between polynya size and regional sea-ice extent, which is a positive relationship in the Ross Sea and a negative relationship in Baffin Bay.
Our hypothesis is that the significant negative correlation between 2Barrel d-excess and regional air temperature is due to the strong influence of temperature on sea-ice concentration, and the sea-ice–d-excess relationship described above. Ice-core d-excess records have often been interpreted as representing changing sea surface temperatures (SST) at the moisture source region (Reference Vimeux, Masson, Jouzel, Stievenard and PetitVimeux and others, 1999; Reference Masson-DelmotteMasson-Delmotte and others, 2005), but an increase in Baffin Bay SST would lead to an increase in d-excess, contrary to the observed relationship. It is further possible that temperature-dependent post-depositional processes at the 2Barrel ice-core site significantly influence the d-excess of the snowpack (Reference Johnson, Clausen, Cuffey, Hoffman, Schwander, Creyts and HondohJohnson and others, 2000), but our current hypothesis is that any such effect is minor compared to the sea-ice influence.
We also evaluated a potential temperature–d-excess relationship via the NAO, which has a strong negative relationship with northern Baffin Bay temperature as recorded at Thule (annual NAO–T Thule r = −0.42 from 1952 to 2012, p < 0.001; winter NAO–T Thule r = −0.54, p < 0.001), and a consequential positive relationship with Baffin Bay sea-ice concentration (SICBB; annual NAO–SICBB r = 0.34, p < 0.05 from 1979 to 2012; winter NAO–SICBB r = 0.48, p < 0.005). B.G. Kopec and others (unpublished information) find a negative relationship between Canadian Arctic precipitation d-excess and the NAO due to the NAO’s strong influence on meridional transport. A positive NAO state is associated with stronger northerly winds in Baffin Bay and eastern Arctic Canada, increasing the proportion of low-d-excess Arctic Ocean moisture to their precipitation sites (B.G. Kopec and others, unpublished information). However, we do not find a statistically significant relationship between 2Barrel d-excess and the NAO from 1990 to 2011. We hypothesize that this is because an increase in low-d-excess Arctic Ocean moisture advection to 2Barrel during NAO positive conditions (due to stronger northerly winds) is offset by a decrease in the advection of low-d-excess Baffin Bay moisture due to enhanced sea-ice concentration.
Influence of the NAO and AMO on Thule temperature
Although we argue that the 2Barrel ice core is sensitive to local–regional conditions in Baffin Bay, temperatures in this region are sensitive to the state of the NAO and AMO, so the 2Barrel temperature proxy record is indicative of these important modes of North Atlantic ocean–atmosphere variability. This is reflected in the large region of strong correlation between ERA-Interim annual temperatures and the 2Barrel MSA–d-excess–accumulation regression model for Thule annual temperature (Fig. 8). Correlations between Thule temperature and the NAO and AMO show distinct seasonal biases. The annual NAO–Thule-temperature correlation is r = −0.46 (p < 0.001) from 1952 to 2012, with the strongest correlations in autumn (r = −0.62, p < 0.001) and winter (r = −0.54, p < 0.001). The annual AMO-Thule-temperature correlation is r = 0.51 (p < 0.001) from 1952 to 2012, with the strongest correlation in summer (r = 0.58, p < 0.001). Thus, enhanced wintertime southerly flow in Baffin Bay associated with NAO negative conditions (weakened Icelandic low) increases Thule wintertime temperatures, as do higher summertime SSTs in the North Atlantic. A regression model based on annually averaged NAO and AMO indices explains 33% of the annual Thule temperature variance (r = 0.57, p < 0.001; Fig. 9). Interestingly, the residual signal rises consistently from 1952 to 2012 at a rate of 0.24°C decade−1. We hypothesize that this residual represents the influence of anthropogenic greenhouse gases superimposed on the annual–decadal temperature variability related to the NAO and AMO.
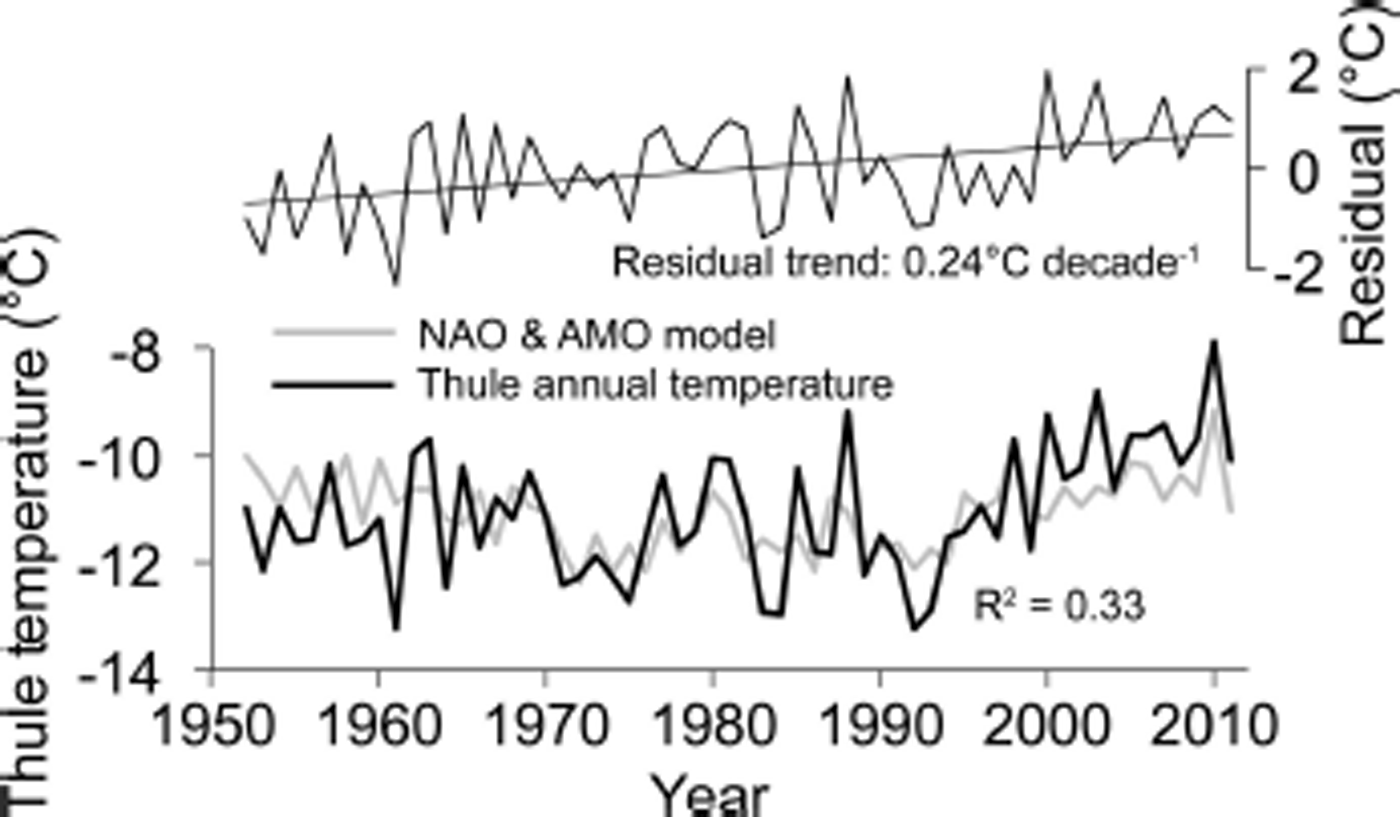
Fig. 9. Thule annually averaged temperature compared to a regression model using annually averaged AMO and NAO index values, showing that a third of the temperature variance can be ascribed to these modes of ocean–atmospheric circulation. The residual trend rising at 0.24°C decade−1 is interpreted to represent the influence of anthropogenic greenhouse gases.
Conclusions
The MSA and d-excess records from the coastal 2Barrel ice core have statistically significant correlations with spring–summer (May–July) and annual (January–December) sea-ice concentrations in Baffin Bay, and summer (June–August) and annual (January–December) temperatures in Baffin Bay and surrounding areas. We interpret high 2Barrel MSA during low sea-ice years (negative relationship) as evidence for enhanced primary production in Baffin Bay, consistent with negative MSA–sea-ice relationships at coastal Arctic aerosol stations (Reference SharmaSharma and others, 2012) and with the Penny ice core MSA–sea-ice relationship (Reference FisherFisher and others, 1998; Reference Kinnard, Zdanowicz, Fisher, Isaksson, de Vernal and ThompsonKinnard and others, 2011). We hypothesize that the 2Barrel site is particularly sensitive to changes in the length of spring–summer ice-free conditions because it receives a significant portion of its moisture from a local region (Baffin Bay) that has consistent winter sea-ice cover. This is in contrast to central Greenland ice-core sites and many sites in Antarctica, where the moisture sources impacting the sites are characterized by significant changes in winter sea-ice extent, which can lead to a positive MSA–sea-ice relationship (Reference Curran, Van Ommen, Morgan, Phillips and PalmerCurran and others, 2003; Reference Abram, Wolff and CurranAbram and others, 2013).
The strong, positive 2Barrel d-excess–sea-ice relationships described here provide further support that the core is sensitive to sea-ice conditions in Baffin Bay. Years with lower (higher) Baffin Bay sea-ice concentrations are associated with more (less) low-d-excess Baffin Bay moisture at the ice-core site, consistent with recent studies of Arctic precipitation d-excess (B.G. Kopec and others, unpublished information). Such a sea-ice–d-excess relationship has only been previously documented once from a coastal Antarctic ice-core site in the Ross Sea region (Reference Sinclair, Bertler, Bowen and ArrigoSinclair and others, 2014).
Due to the strong relationship between temperature and sea-ice concentration in Baffin Bay, the 2Barrel MSA and d-excess time series are significantly correlated with summer and annual Thule temperatures. The multilinear regression models become more robust when the 2Barrel snow accumulation record is also incorporated. Temperatures in northern Baffin Bay are significantly correlated with the NAO and AMO, particularly during the fall–winter and summer, respectively (Reference HannaHanna and others, 2013). Thus, although recording local climate conditions in Baffin Bay, the 2Barrel ice core is sensitive to these larger-scale modes of atmospheric and oceanic circulation variability.
Our results indicate that a deeper, Holocene-length record from the 2Barrel site would be a valuable complement to deep ice-core sites in central, interior Greenland, providing a focused view of Holocene temperature and sea-ice conditions in Baffin Bay. Such a record would be particularly valuable in combination with temperature records from lake cores and landscape records of northwest Greenland ice-margin advance and retreat, using the early-Holocene climatic optimum as an analog for understanding the sensitivity of the northwest Greenland ice sheet and Baffin Bay sea ice to warmer conditions.
Acknowledgements
The US National Science Foundation Arctic Natural Sciences Program supported this research through grants ARC-0909265 to Hawley and ARC-1107411 to Osterberg. Eric Lutz, Ashley Corbett and Alexandra Giese assisted in collecting the 2Barrel ice core, and Sarah Caughey assisted in assembling the Thule weather station data. We thank CH2M HILL Polar Services, and particularly Kim Derry, Kyli Cosper and Joe Hurley, for assistance with field logistics. We also gratefully acknowledge Sean Birkel and his climate reanalyzer tool (www.cci-reanalyzer.org) for facilitating the production of many of the graphics herein.