The prevalence of diabetes mellitus (DM) in pregnancy is rising in part due to the sharp increase in obesity and type 2 DM(Reference Bell, Bailey and Cresswell1). Fetal exposure to hyperglycaemia during pregnancy can have profound and long-lasting consequences to the offspring(Reference Freinkel2). In the Northwestern University Diabetes in Pregnancy follow-up study, 40 % of offspring of diabetic mothers at the age of 16 years had impaired glucose tolerance, defined as blood glucose 7·8–11·0 mmol/l 2 h after receiving a 75 g glucose load(Reference Metzger3). Furthermore, the occurrence of impaired glucose tolerance was associated with higher insulin in amniotic fluid(Reference Silverman, Metzger and Cho4). Based on the SEARCH Case–Control study, 30·4 % of youth with type 2 DM were exposed to maternal diabetes in utero compared with 6·3 % of non-diabetic control youth(Reference Dabelea, Mayer-Davis and Lamichhane5).
Whether altered maternal–fetal nutrient transfer or fetal metabolism explains the propensity for offspring of mothers with DM to develop obesity, impaired glucose tolerance and DM is not clear. In cell culture, arachidonic acid (AA) is fundamental to functional integrity of the pancreatic β-cell(Reference Dixon, Nolan and McClenaghan6), while glucose-stimulated insulin release is dependent on the plasma membrane release of AA(Reference Konrad, Major and Wolf7). Furthermore, insulin sensitivity is positively associated with higher AA status in human subjects(Reference Borkman, Storlien and Pan8). In rats, dietary AA supplementation lowers fasting blood glucose and insulin concentrations(Reference Sasagawa, Ishii and Hasuda9), prevents high-fat diet-induced insulin resistance(Reference Wu, Wang and Duan10) and enhances glucose disposal(Reference Song, Hwang and Rosenthal11). However, women with DM have lower AA in erythrocytes, particularly if obese(Reference Min, Ghebremeskel and Lowy12), and newborn infants of women with DM also have reduced AA in cord blood(Reference Ortega-Senovilla, Alvino and Taricco13–Reference Thomas, Ghebremeskel and Lowy18). Lower neonatal status is likely a reflection of lower maternal AA status, placental sequestration of AA and increased fetal utilisation(Reference Ortega-Senovilla, Alvino and Taricco13). Several studies have explored the possibility of maternal dietary intervention in modifying the susceptibility of offspring to adult diseases(Reference Ibrahim, Basak and Ehtesham19–Reference Korotkova, Gabrielsson and Holmang21). The differences in body weight and fasting insulin levels in adult rat offspring relate to the n-6:n-3 fatty acid ratio in the maternal diet during the perinatal period(Reference Korotkova, Gabrielsson and Holmang21). Similar effects have also been observed in diabetic pregnant rats and their adult offspring(Reference Soulimane-Mokhtari, Guermouche and Yessoufou22). We thus hypothesised that maternal supplementation with AA during pregnancy and lactation would prevent subsequent development of impaired glucose tolerance in the offspring at young adult age.
New evidence suggests that there are linkages between glucose metabolism and bone metabolism(Reference Confavreux, Levine and Karsenty23). Since newborn infants of diabetic mothers have compromised bone growth and mineralisation(Reference Tsang, Kleinman and Sutherland24–Reference Verhaeghe, Van Herck and Bouillon26), as well as reduced AA status(Reference Ortega-Senovilla, Alvino and Taricco13–Reference Thomas, Ghebremeskel and Lowy18), we hypothesised that maternal AA supplementation may also be beneficial to offspring body composition and bone health. AA is a precursor to PGE2 that has a biphasic effect on bone by enhancing bone formation at lower concentrations in animals while stimulating resorption at higher concentrations(Reference Watkins, Li and Seifert27). Alteration in the precursor pool affects PGE2 metabolism, while modification of dietary fatty acids leads to parallel alterations in bone fatty acids(Reference Weiler28) and PGE2 metabolism(Reference Watkins, Shen and McMurtry29, Reference Alam, Kokkinos and Alam30). In a recent study, serum AA was positively associated with whole body bone mass in 8-year-old children(Reference Eriksson, Mellstrom and Strandvik31). Dietary γ-linoleic acid (a precursor to AA) supplementation enhanced bone mass in young male rats(Reference Claassen, Potgieter and Seppa32), and was effective against diabetes-induced fetal bone development defects(Reference Braddock, Siman and Hamilton33). Similarly(Reference Korotkova, Gabrielsson and Holmang21), variations in fatty acid composition in the maternal diet during the perinatal period caused changes in bone growth in adult rat offspring(Reference Korotkova, Ohlsson and Hanson34, Reference Korotkova, Ohlsson and Gabrielsson35). Thus, in the present follow-up study, we sought to define the effect of maternal diabetes and AA supplementation on offspring body composition including bone mass and glucose tolerance up to 12 weeks of age.
Materials and methods
Dam streptozotocin-induced diabetes treatment and dietary arachidonic acid supplementation
The study design, time course and diet composition (Table 1) have been published in detail(Reference Zhao, Del Bigio and Weiler36). Briefly, 9-week-old female Sprague–Dawley rats (University of Manitoba breeding colony) were randomised into six groups using a 3 × 2 design (five animals/group) and permitted a 1-week adaptation period while being fed the control diet. Diabetes was induced on week 1 using streptozotocin (STZ) (60 mg/kg intraperitoneally; Sigma-Aldrich, St Louis, MO, USA); control received an equivalent amount of saline denoted as saline-placebo. Diabetes was confirmed by blood glucose >13 mmol/l using a glucometer (One Touch® Ultra®) and blood sampling from the saphenous vein 5 d later at 16.00 hours. Five dams with glucose < 13 mmol/l were given a second dosage of STZ, and the increase in glucose was confirmed again 5 d later. At 1 week after STZ injection (week 2), all the diabetic rats received dosages of insulin (glargine Lantus®, 0·5–10 unit/d subcutaneously) until postpartum week 4. Half of the diabetic rats had glucose controlled at < 13 mmol/l as good control (STZ/GC), and the other half as poor control (STZ/PC) with glucose 13–20 mmol/l. Glucose before insulin management in the STZ/GC group ranged from 14·6 to 30·3 mmol/l, while in the STZ/PC group, glucose ranged from 21·8 to 33·3 mmol/l.
Table 1 Major composition of the diets (g/kg)

AA, arachidonic acid; FA, fatty acid; LA, linoleic acid; ALA, α-linolenic acid.
All ingredients were purchased from Harlan Teklad (Madison, WI, USA) except for the AA, which was provided in the form of RBD-ARASCO® (Martek Biosciences Corporation, Columbia, MD, USA).
The dietary AA intervention started on the same day (week 2) as the insulin treatment. Half of the dams continued on control diet, which was modified from AIN-93G(Reference Reeves37) with maize starch as the sole source of carbohydrate. The other half was fed the AA diet, which was the same diet but with 0·5 g/100 g of fat as AA (RBD-ARASCO: 40·6 % AA; Martek Biosciences Corporation, Columbia, MD, USA).
After the 1-week adaptation period to insulin treatment and diet (week 3), dams were mated and continued on their respective diets and treatments, and the pups were reared by their natural dams until postnatal (PN) week 4. On PN day 3, the pups were weighed and randomised to end points (cull or PN week 4 or 12); then, litters were culled to ≤ 8 pups, keeping equal numbers of each sex when possible. At PN week 4, half of the pups were euthanised with dams for measurement of fatty acid status at weaning; the other half of the pups were transferred to a regular chow (LabDiet® 5012: 23 % crude protein, 6 % crude fibre and 4·5 % fat) and housed in same-sex groups of up to four per cage until 12 weeks of age (PN week 12) for a long-term assessment. Thus, based on the dams’ treatment and diet, the six groups of offspring were as follows: S+C (saline-placebo+control diet); S+A (saline-placebo+AA diet); STZ/GC+C (STZ/GC+control diet); STZ/GC+A (STZ/GC+AA diet); STZ/PC+C (STZ/PC+control diet); STZ/PC+A (STZ/PC+AA diet).
Throughout the entire study period, dams were housed individually or with pups, and all rats were housed in standard hanging cages with controlled temperature (21–23°C), humidity (55 %) and 12 h light cycle, and fed ad libitum. Dam food intake was monitored daily. The institutional and national guidelines for the care and use of animals were followed, and all the experimental procedures involving animals were approved by the University of Manitoba Protocol Management and Review Committee.
Offspring body composition and bone mass
Weight was measured weekly for the assessment of growth using a digital scale (Mettler Toledo SB32000, Columbus, OH, USA). At 4, 8 and 12 weeks of age, rats were anaesthetised using isoflurane gas (PrAErrane; Baxter Corporation, Mississauga, ON, Canada) for the measurement of bone mass including whole body, lumbar spine, femur and tibia using a small animal program and dual-energy X-ray absorptiometry (DXA, QDR 11.2, 4500A series; Hologic, Bedford, MA, USA). Whole body composition values were obtained for lean mass, fat mass and fat percentage. Whole body length from tip of nose to base of tail was measured in the anaesthetised state using a non-stretchable measuring tape. All scans were performed in singlet with the rat in the posterior position with limbs extended. The DXA measurements have been validated using Hologic hardware and software for rats that are 130 g and higher in weight for whole body assessments, and in rodents as small as in mice(Reference Wlodarski and Dickson38) for high-resolution regional scans.
Offspring oral glucose tolerance test
Oral glucose tolerance tests (OGTT) were conducted at 8 and 12 weeks of age according to published methods(Reference Kawa, Taylor and Przybylski39) and before the DXA scan. Briefly, after 10 h without food, blood was collected via saphenous vein for the 0 time point. Immediately following, rats were fed an oral dose of 70 % glucose solution (1 g glucose/kg body weight) using a syringe(Reference Kawa, Taylor and Przybylski39). Blood was collected accordingly at 7·5, 15, 30 and 60 min after administration of the glucose solution, and blood glucose was determined using a glucometer (One Touch® Ultra®). The area under the curve (AUC) was calculated from OGTT results using the trapezoidal method(Reference Purves40), which has been validated for glucose tolerance testing(Reference Allison, Paultre and Maggio41).
Tissue collection
At 12 weeks of age, rats were anaesthetised with isoflurane gas (PrAErrane; Baxter Corporation) and followed by exsanguination via cardiac puncture. Blood was collected, and stored on ice until centrifuged at 1500 rpm for 15 min at 4°C. Liver was collected and flash frozen; serum and liver were stored at − 80°C until analysis.
Liver fatty acid analysis
To reflect fatty acid status in response to maternal treatments, liver fatty acids were analysed in dams (postpartum week 4) and offspring (PN weeks 4 and 12). Total lipids from tissues were extracted according to the method from Folch et al. (Reference Folch, Lees and Sloane Stanley42). Briefly, after adding an internal standard (C17 : 0), liver lipid was extracted in chloroform–methanol (2:1, v/v) containing butylated hydroxytoluene. Lipid extracts were transmethylated in methanolic HCl at 80°C for 1 h. Fatty acids from twelve to twenty-two carbons were quantified (mg/g tissue) using GC (Varian Star 3400, Mississauga, ON, Canada). Only values for AA and DHA are reported in view of their importance in maternal–fetal transfer. Additionally, DHA has a stronger effect on bone mineral accretion than EPA in growing rats(Reference Kruger and Schollum43).
Statistical analysis
Data are presented as groups by maternal treatment and diet, offspring sex and age; and they are expressed as means with their standard errors. Main and interaction effects were identified using a mixed (repeated measurements) model procedure of the Statistical Analysis Systems program (version 9.1; SAS Institute, Cary, NC, USA), as it is suitable for the randomised 3 × 2 factorial design, and recommended by the University of Manitoba Statistical Advisory Service. Significant differences (P < 0·05) among means of groups by maternal treatments or any interaction effects were assessed using Bonferroni's post hoc test.
The correlations between dam mean gestational glucose (mmol/l) and offspring OGTT, DXA scan outcome measurements were detected using Pearson's correlation coefficient r to quantify the strength of the relationship. Correlations between liver AA and DHA (mg/g tissue) from dams and offspring of all ages with offspring OGTT, DXA scan outcome measurements of all ages were examined. Values at 4 weeks of age were averaged per litter per sex to examine longitudinal relationships with same-sex littermates. Values at 8 and 12 weeks of age were available for all offspring, and thus individual values were used in correlation analyses.
Results
Experimental system
Induction and control of diabetes were successful as indicated by the mean gestational glucose concentrations at targeted ranges (Table 2). No significant difference was observed among the mean number of pups per litter/group (P>0·05). There were no differences in feed intake between different groups at any given days from mating to delivery (range: 21–36 g/d) or during lactation (39–58 g/d) regardless of treatment and diet.
Table 2 Characteristics of dam and day 3 pup body weight
(Mean values with their standard errors)

S, saline injection; C, control diet; A, arachidonic acid diet; STZ, streptozotocin; STZ/GC, STZ-induced diabetes with good control (glucose < 13 mmol/l); STZ/PC, STZ-induced diabetes with poor control (glucose 13–20 mmol/l); TRT, treatment.
a,b.c Mean values within a row with unlike superscript letters were significantly different (P < 0·05); capital superscript letters (X, Y, Z) are used for main effect of maternal treatment when both diet groups are combined, and the lower case superscript letters (a, b) are used for diet and treatment interaction.
Offspring body composition
Total weight gain (from day 3 to 12 weeks) was greater in the STZ/GC offspring compared with STZ/PC offspring regardless of maternal diet (587·1 v. 499·5 %, P = 0·009), while the saline-placebo offspring were intermediate (543·7 %, P = 0·252). Main effects of sex and age were detected in body weight, body length (data not shown), lean mass and fat mass (Table 3, all P < 0·0001). The sex and age effects were manifested as values in male rats being higher than female rats and values at 4 and 8 weeks of age being less than those at 12 weeks of age. There were no main effects (all P>0·05) of maternal diabetes or AA diet on offspring body weight, body length, lean mass or fat mass.
Table 3 Body composition measured at 4, 8 and 12 weeks of age*
(Mean values with their standard errors)

S, saline-placebo; C, control diet; A, arachidonic acid diet; STZ, streptozotocin; STZ/GC, STZ-induced diabetes with good control (glucose < 13 mmol/l); STZ/PC, STZ-induced diabetes with poor control (glucose 13–20 mmol/l); TRT, treatment; M, male; F, female; WB, whole body.
* Sample size was different between 4 weeks and 8, 12 weeks as a result of tissue sampling at 4 weeks.
Offspring bone area, bone mineral content and density
Maternal diabetes and gestational glucose control had significant effects on bone area, as STZ/PC offspring had smaller bone area than offspring of saline-placebo and STZ/GC at 4 weeks in whole body (P ≤ 0·044) and at 12 weeks in tibia (P ≤ 0·028) (Fig. 1). Femur area of STZ/PC offspring at 8 weeks was less (P = 0·024) than that of STZ/GC offspring, while there was no difference between offspring of STZ/PC and saline-placebo. There was no effect of maternal treatment on offspring bone area in lumbar spine (P>0·05, data not shown). There was no effect of maternal treatment on offspring bone mineral content (BMC) and bone mineral density (BMD) at any age (P>0·05). Main effects of offspring sex and age were observed for all bone area, BMC and BMD (data not shown) measurements with values increasing over time (P < 0·05). Values for all treatment groups combined were higher for males than females at 12 weeks for whole body BMC (12·09 (sem 0·15) v. 8·35 (sem 0·11) g, P < 0·05) and whole body BMD (0·155 (sem 0·003) v. 0·149 (sem 0·002) g/cm2, P < 0·05). There was no effect of maternal diet on offspring bone area, BMC and BMD.

Fig. 1 Bone area (cm2) of whole body (4 weeks of age), femur (8 weeks of age) and tibia (12 weeks of age). STZ/GC, STZ-induced diabetes with glucose < 13 mmol/l; STZ/PC, STZ-induced diabetes with glucose 13–20 mmol/l. Values are means with their standard errors, which are represented by vertical bars and graph, with unlike letters are significantly different (P < 0·05). Sample size is as follows: (A) at 4 weeks, saline-placebo n 52, STZ/GC n 57, STZ/PC n 61; ((B) and (C)) at 8 and 12 weeks, saline-placebo n 27, STZ/GC n 30, STZ/PC n 33.
Offspring oral glucose tolerance test
No main effects (P>0·05) of maternal treatment, diet or offspring sex were detected on the OGTT tested at 8 and 12 weeks of age. However, glucose AUC during OGTT (AUC glucose0–60) was greater at 8 weeks than at 12 weeks (443·7 (sem 4·8) v. 385·2 (sem 4·5) mmol/l × min, P < 0·0001) regardless of maternal treatment and diet.
Liver arachidonic acid and DHA
Dams from STZ/GC plus control diet had significantly lower liver AA than dams from saline-placebo plus AA diet and STZ/PC plus control diet, while those receiving STZ/GC plus AA diet and saline-placebo plus control diet were not different from any group (Table 4). The STZ/GC dams had lower (P = 0·04) liver DHA than saline-placebo dams, whereas STZ/PC dams were intermediate.
Table 4 Liver arachidonic acid (AA) and DHA (mg/g tissue) measured at postpartum week 4 of dams and offspring at 4 and 12 weeks of age
(Mean values with their standard errors)

S, saline-placebo; C, control diet; A, arachidonic acid diet; STZ, streptozotocin; STZ/GC, STZ-induced diabetes with glucose < 13 mmol/l; STZ/PC, STZ-induced diabetes with glucose 13–20 mmol/l; TRT, treatment.
Mean values within a row with unlike superscript letters were significantly different (P < 0·05); capital superscript letters (X, Y) are used for main effect of maternal treatment when both diet groups are combined; capital superscript letters (A,B) are used for main effect of maternal diet when treatments are combined; and the lower case superscript letters (a,b) are used for diet and treatment interaction.
At 4 weeks, the AA diet offspring had higher (P < 0·004) liver AA than control diet offspring. No difference was observed in offspring liver DHA regardless of dams’ treatment and diet.
For 12-week-old rats, no difference was observed in offspring liver AA regardless of dams’ treatment and diet. The STZ/GC offspring had higher (P = 0·023) liver DHA than offspring of STZ/PC and saline-placebo dams. The AA diet offspring had lower (P = 0·030) liver DHA than control diet offspring.
At 4 weeks, male rats had higher liver AA and DHA than female rats, while at 12 weeks, the female rats had higher liver DHA than male rats (all P < 0·04).
Associations between dam treatment, diet and offspring outcome measurements
Glucose AUC of OGTT (AUC glucose0–60) in 8- and 12-week-old offspring did not correlated to any variables that examined including dams’ mean gestational glucose, dam (postpartum week 4) and offspring liver AA and DHA (4 and 12 weeks) (data not shown).
Dams’ gestational glucose concentration had a significant relationship with bone mass of their offspring at 4 weeks of age. Maternal glucose was negatively correlated with whole body (male: r − 0·41; P = 0·039; and female: r − 0·51; P = 0·013) and lumbar spine (female: r − 0·42; P = 0·047) bone area (Fig. 2), as well as whole body BMC (male: r − 0·41; P = 0·039; and female: r − 0·53; P = 0·010). A negative correlation appeared at 12 weeks in male rats (r − 0·43; P = 0·038) between maternal glucose and tibia bone area (Fig. 2). In addition, dams’ gestational glucose concentration was negatively correlated with offspring whole body fat (male: r − 0·44; P = 0·030; and female: r − 0·49; P = 0·018) and body weight in female rats (r − 0·45; P = 0·032) at 4 weeks, but not thereafter at 8 or 12 weeks.
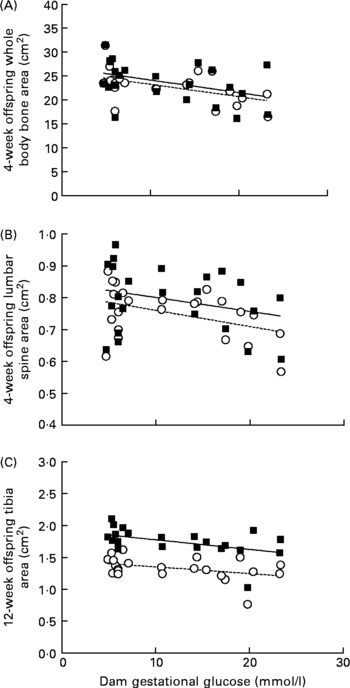
Fig. 2 Correlations between mean gestational glucose concentration (mmol/l) of dams and offspring bone area (cm2) of whole body and lumbar spine at 4 weeks and tibia at 12 weeks of age. Sample size is as follows: at 4 weeks, male (M (■)) n 90, female (F (○)) n 80; at 12 weeks, male n 48, female n 42. (A) M (r − 0·41, P = 0·039), F (r − 0·51, P = 0·013); (B) M (r − 0·28, P = 0·192), F (r − 0·42, P = 0·047) and (C) M (r − 0·43, P = 0·038), F (r − 0·39, P = 0·071).
Maternal liver AA and DHA did not correlated with any measure of bone in 4-week-old offspring. However, in female offspring, maternal DHA was negatively correlated with femur (r − 0·48; P = 0·030) and tibia BMC (r − 0·47; P = 0·038) and tibia BMD (r − 0·50; P = 0·026) at 12 weeks. The only relationships observed between liver fatty acids and bone of the offspring were positive correlations in males between liver AA and lumbar spine BMD (r 0·30; P = 0·05) and liver DHA with lumbar spine BMD (r 0·30; P = 0·049) at 4 weeks of age. In male offspring at 4 weeks, liver AA and DHA were positively correlated (r 0·86; P < 0·0001). No long-term associations between offspring liver AA were observed with bone area, BMC or BMD (data not shown).
Discussion
The most novel observation of the present study is the long-term adverse relationship between maternal hyperglycaemia and skeletal size of adult offspring. Specifically, offspring from poorly controlled diabetic dams (STZ/PC) had significantly smaller bone area of whole body, femur and tibia than offspring from diabetic dams in good control (STZ/GC). The maternal glucose concentration during pregnancy was inversely related to offspring whole body BMC and bone area at 4 weeks of age and persisted to 12 weeks for tibia area in male rats. This is particularly important in view of the fact that peak bone mass is considered to be well established by 12 weeks of age in rats(Reference Sengupta, Arshad and Sharma44), and therefore suggests that skeletal size is programmed by fetal exposure to maternal hyperglycaemia. Similarly, human infants born to mothers with DM have low BMC at the distal radius(Reference Mimouni, Steichen and Tsang25), and osteoblastic activity(Reference Verhaeghe, Van Herck and Bouillon26) and BMC are inversely related to maternal glucose(Reference Mimouni, Steichen and Tsang25). Furthermore, altered Ca and Mg homoeostasis exists in newborn infants of mothers with DM(Reference Tsang, Kleinman and Sutherland24–Reference Verhaeghe, Van Herck and Bouillon26) that persists beyond infancy to at least 16 weeks in rats(Reference Bond, Hamilton and Balment45) and 10 years in children(Reference Mughal, Eelloo and Roberts46). While it is postulated that this is a compensatory mechanism to support BMD, it appears that cortical density of femur becomes normal while reduced trabecular density persists to 16 weeks in rats(Reference Bond, Hamilton and Balment45, Reference Bond, Sibley and Balment47). Correspondingly, at the completion of our 12-week study, BMC and BMD of the STZ offspring were not different from control offspring, although bone architecture was not examined. The present study adds important new information that maternal hyperglycaemia has long-term consequences to bone size of the offspring, implying an altered growth trajectory.
The inverse association between maternal liver DHA with tibia mineral content and density in 12-week-old female offspring is also consistent with the fetal origins of disease hypothesis(Reference Sayer and Cooper48). Even though this hypothesis considers both fetal and neonatal factors, our data suggest that the observations are more closely linked to fetal events since the 12-week measures of BMC and BMD did not relate to liver DHA in neonatal offspring. The mechanism(s) by which DHA exposure in utero might limit BMC and BMD later in life is elusive. Although various eicosanoids act on bone, the PG seem to be the primary mediators of bone cell function(Reference Watkins, Lippman and Le Bouteiller49). PGE2 exhibits biphasic effects on bone, enhancing formation at moderate concentrations but inhibiting formation at both high and low concentrations(Reference Raisz and Fall50). There is evidence in adult guinea pigs that PG synthesis is programmed by fetal exposure to long-chain PUFA(Reference Aprikian, Reynaud and Pace-Asciak51). DHA is known to inhibit osteoclastogenesis(Reference Yuan, Akiyama and Nakahama52, Reference Poulsen, Wolber and Moughan53) that is required for modelling of bone during growth(Reference Narducci, Bareggi and Nicolin54). Since bone area was not associated with DHA, it is likely that the lower BMC and BMD are linked to altered architecture. Indeed, feeding maternal diets (linseed oil) to elevate fetal exposure to DHA compared to a balance in n-3:n-6 fatty acids (soyabean oil) results in lower femur length and lower cortical cross-sectional area and cortical BMC at the mid-diaphysis in 30-week-old female offspring(Reference Korotkova, Gabrielsson and Holmang21, Reference Korotkova, Ohlsson and Hanson34). Even in human subjects, mothers with higher erythrocytes DHA have neonates with lower spine and femur BMC(Reference Weiler, Fitzpatrick-Wong and Schellenberg55). These three studies imply that higher amounts of DHA in maternal tissue, through an unidentified mechanism in fetal development, programme the fetus for lower BMC and BMD that is apparent as early as infancy in human subjects(Reference Weiler, Fitzpatrick-Wong and Schellenberg55) and continues to adulthood as evidenced in rats(Reference Korotkova, Ohlsson and Hanson34).
In the present study, maternal diabetes and diet did not affect glucose tolerance in the offspring when measured at 8 and 12 weeks postnatally. To mimic the clinical management of diabetes in pregnant women(Reference Walker56), insulin was used to control glucose, and the offspring were nursed by their natural dams. Thamotharan et al. (Reference Thamotharan, McKnight and Thamotharan57) has reported glucose intolerance, as well as increased food intake and obesity in 60- and 180-day-old STZ offspring who were fostered to normal dams, but not in those fostered to diabetic dams. It is possible that our proven method for OGTT(Reference Kawa, Taylor and Przybylski39) was not sufficient (1 g glucose/kg body weight) to detect early signs of diabetes in comparison to other studies that used higher dosages and bypassed the gastrointestinal tract (2 g/kg intraperitoneally or intravenously)(Reference Han, Xu and Long58). While it is conceivable that the normal glucose tolerance observed in the present study might have been accompanied by hyperinsulinaemia, a subgroup analysis using 8-week data showed that insulin was 200·0 v. 183·4 pmol/l at 30 min into the OGTT in saline-placebo and STZ/GC groups. Thus, hyperinsulinaemia was not a factor in the present study. Future studies should confirm these observations using higher dosages or more sensitive tests such as the euglycaemic–hyperinsulinaemic clamp that readily identified glucose intolerance in 12-week-old STZ offspring reared by the biological dams(Reference Holemans, Aerts and Van Assche59).
In addition to the benefits of glucose control in the mother, many studies have postulated that dietary long-chain PUFA will also confer long-term benefits to the offspring. In rats, inclusion of fish oil in the maternal diet during pregnancy offsets the adverse effects of a low-protein diet (12 %, w/w) that otherwise causes insulin resistance in the offspring at 11 months of age(Reference Joshi, Rao and Golwilkar60). Feeding diabetic rat dams with diets containing 35 % of fat as EPA and 6 % of fat as DHA limited the development of insulin resistance in 12-week-old adult offspring compared to the control diet that had a n-6:n-3 ratio of 28:1(Reference Soulimane-Mokhtari, Guermouche and Yessoufou22). In contrast, in the OGTT, we did not observe any maternal diet effects. In addition to maternal insulin treatment, this could also be ascribed to the standard protein (20 % in experimental diets and 23 % in regular chow) in all groups and the lower n-6:n-3 ratio of 7:1. In the present study, the level of dietary supplementation of AA in the dams diet (0·5 % of fat) was based on Otto et al. (Reference Otto, van Houwelingen and Hornstra61). The present results suggest that such supplementation is safe in regard to long-term growth, body composition and glucose tolerance.
In summary, the present study suggests that maternal hyperglycaemia in pregnancy has a long-lasting adverse effect on skeletal growth in the offspring. Specifically, maternal diabetes was linked to lower tibia area. Regardless of maternal diabetes, higher maternal DHA indirectly related to lower tibia BMC and BMD female offspring at adult ages, suggesting programmed architecture of long bone. Whether the mechanism is ascribed to DHA or eicosanoid metabolism requires further study.
Acknowledgements
We would like to acknowledge the assistance of Sarah L. Finch, Andrea Maclntosh and Lisa Maximiuk on OGTT, and Gerald Nolette and Denise Borowski for providing diligent animal care and support in University Manitoba Health Sciences Centre animal facility. Each author was involved in the study design, whether in relation to conception of the study or to method development, and was fully involved in study conduct (J. Z.), data analysis (J. Z.) and the final manuscript (all authors). All authors have no conflict of interest. The present study was supported by grants from the Canadian Institute Health Research and the Manitoba Institute of Child Health.