1 Introduction
A high-repetition-rate single-longitudinal -mode laser with a narrow pulse width and a high pulse energy is promising for applications in the fields of fast ignition lasing radiation[ Reference Zhang, Cai, Zhou, Dai, Shan, Xu, Chen, Ge, Tang, Zhang, Wei, Liu, Gu, Du, Bi, Wu, Li, Lu, Zhang, Zhang, He, Yu, Yang, Wang, Zhang, Cui, Yang, Wu, Qi, Cao, Li, Liu, Yang, Ren, Tian, Yuan, Zheng, Cao, Zhou, Zou, Gu, Du, Ding, Zhang, Zhu, Zhang and He 1 ], space debris detection[ Reference Fan, Qiu, Tang, Bai, Kang, Ge, Wang, Liu and Liu 2 ], LIDAR Thomson scattering diagnosis[ Reference Zhang, Dai, He, Zhao, Huang, Li, Huang and Cao 3 ], etc. Stimulated Brillouin scattering (SBS) is a simple and efficient pulse-compression technology[ Reference Lian, Wang, Zhang, Han, Yu, Qi, Luan, Bai, Wang and Lü 4 – Reference Shi, Xu, Guo, Luo, Li and He 7 ] that can compress long nanosecond pulses with a high energy to sub-nanosecond pulses. By combining SBS technology and main oscillation power amplification technology, a pulse laser with the four parameters of high repetition rate, high energy, sub-nanosecond pulses and high efficiency can be achieved[ Reference Lü, Peng, Wang, Zhao, Zhu and Leng 8 – Reference Qiu, Tang, Fan and Wang 12 ].
In SBS pulse compression, the liquid medium used for Brillouin scattering has the advantages of no high pressure, a small absorption coefficient and a high laser damage threshold compared with gas and solid media, and is most suitable for SBS with high injection energies and long interaction distances[ Reference Feng, Xu and Diels 9 – Reference Xu, Feng and Diels 11 , Reference Li, Wu, Zuo, Zeng, Wang, Wang, Mu, Hu and Su 13 – Reference Kang, Fan, Huang, Zhang, Ge, Li, Yan and Zhang 16 ]. Therefore, SBS pulse compression based on the liquid medium is the most promising technical method for obtaining a high-energy sub-nanosecond laser with a kilohertz-level repetition rate.
However, most of the work in this field has focused on SBS pulse-compression characteristics for xenon lamp pumped lasers, whose laser repetition rates are generally low (below tens of hertz)[ Reference Shi, Yang, Cao, Zhang, Wan, He and Chen 17 – Reference Tarasov and Chu 21 ]. Few studies have focused on high-repetition-rate SBS pulse compression based on a liquid medium[ Reference Shilov and Kulagin 22 , Reference Kang, Zhang, Yan, Lang, Bai and Fan 23 ]. For 100-Hz operation, Shilov and Kulagin[ Reference Shilov and Kulagin 22 ] obtained a 350-ps compressed pulse with 6.5 mJ and finally amplified the pulse to 36 mJ using a phase-conjugation-pulse-compression mirror with a single-cell structure. For 200-Hz operation, Kang et al. [ Reference Kang, Zhang, Yan, Lang, Bai and Fan 23 ] adopted multi-layer membrane circulating ultrafiltration technology to control the impurity-particle size below 0.1 μm. The laser repetition rate of SBS pulse compression was increased to 200 Hz with a 376-ps pulse width using a generator-amplifier double-cell structure, and there was no optical breakdown in the generator cell. Our team has also conducted remarkable research on high-repetition-rate SBS pulse compression; we obtained a sub-nanosecond-pulse output with a 1000-Hz repetition rate[ Reference Wang, Cha, Kong, Wang and Lu 24 , Reference Wang, Cha, Wang, Kong and Lu 25 ]. There are two main reasons for the scarcity of studies on high-repetition-rate SBS pulse compression based on a liquid medium. Firstly, the thermal effects caused by the heat accumulation due to the transmission of the high-power laser beam through liquid media limit the operating repetition rate[ Reference Andre, Andreev, Kulagin, Palashov, Pasmanik, Rodchenkov and Powell 26 , Reference Yoshida 27 ]. Secondly, the construction of high-energy nanosecond-pulse single-longitudinal-mode lasers with a tunable repetition rate is complex and expensive. One goal of researchers is to maximize the operating repetition rate of SBS pulse compression based on the liquid medium with a stable output.
Researchers have developed methods for alleviating thermal effects. The effective thermal mitigation methods in the literature can be divided into two categories. The first is the liquid purification method to remove large impurity particles in the liquid medium[ Reference Kang, Fan, Huang, Zhang, Ge, Li, Yan and Zhang 16 , Reference Hasi, Lü, He, Wang and Liu 28 ], which can appropriately reduce the total heat absorption generated by the laser absorption within the medium and reduce the optical-breakdown probability. The second category is the method involving flowing liquid and a wedge-based moving focal spot[ Reference Yoshida 27 , Reference Park, Cha, Oh, Lee, Ahn, Churn and Kong 29 ], which are used to alleviate the local focusing heat accumulation. However, these methods have only been verified separately through experiments at repetition rates of less than 1 kHz, and there have been few studies on these methods that involved higher repetition rates or systematic research.
In this study, using the HT270 liquid medium, high-repetition-rate SBS pulse compression using a combination of the medium purification and rotating off-centered lens methods was experimentally investigated. The applicable conditions of the rotating off-centered lens in SBS pulse compression and the variations in the SBS output parameters with respect to the laser repetition rate were examined. The remainder of this paper is organized as follows. Section 2 introduces the experimental device for SBS pulse compression based on the rotating off-centered lens and the parameters of the Kumgang pump laser. Section 3 presents the theoretical analysis of the thermal problem of high-repetition-rate SBS pulse compression, as well as the SBS output characteristics enhanced by thermal effects. Section 4 presents the results and discussions, and Section 5 concludes the paper. This work has experimental reference value for the application of SBS technology to high-power laser systems.
2 Experimental setup
The experimental setup for SBS pulse compression employed in this study is shown in Figure 1. The laser propagated from left to right, and the injected pump beam imaged the laser image plane at the position of lens L3 through a pair of imaging lenses. The imaging system was composed of lenses L1 and L2 with focal lengths of 0.2 and 0.4 m, respectively. A combination of a half-wave plate (HWP1) and a polarization beam splitter (PBS1) was used to control the input beam energy. The pump light was introduced into an amplifier cell with a length of 1 m through a quarter-wave plate (QWP) and lens L3 with a focal length of 2 m. The beam was then focused into a 0.2-m generator cell using a rotating off-centered lens (L4). In a previous study[ Reference Wang, Cha, Kong, Wang and Lu 30 ], we fabricated a custom lens, which we referred to as an ‘off-centered lens’, by cutting a plano-convex lens. Its center was 6.4 mm from that of the original lens. The diameter of the off-centered lens was 1 inch (1 inch = 2.54 cm). A rotating off-centered lens was used to reduce the heat accumulation at the focal spot for high-repetition-rate operation and improve the beam quality with less coma aberration. The Stokes beam generated by SBS was backscattered light. The reflected beam was separated by a system consisting of a QWP and PBS1. The reflected beam pattern was relayed by a relay imaging system composed of L5 and L6. These lenses relayed the image from the object plane at the QWP to the image plane on a camera (WinCamD-LCM, DataRay, Inc.). The sub-nanosecond pulse was detected by a Thorlabs DET02 photodetector (bandwidth: 1.2 GHz; rise time: 50 ps; fall time: 250 ps). The signal waveform was recorded by a digital oscilloscope with a bandwidth of 3 GHz.

Figure 1 Schematic of the experimental setup for SBS pulse compression based on a rotating off-centered lens (HWP1 and HWP2, half-wave plates; L1–L6, lenses).
The light source used for high-repetition-rate SBS pulse compression was a Kumgang laser system with a tunable repetition rate[ Reference Kong, Park, Cha, Ahn, Lee, Oh, Lee, Choi and Kim 31 ]. The laser system included a kilohertz-level main oscillator seed source, a Nd:YAG regenerative amplifier, and a pre-amplifier. With the pre-amplifier, a pump power of 70 W (35 mJ at 2 kHz) was obtained, and the output wavelength was 1064 nm. The pump pulse widths of the Kumgang laser at different repetition rates are shown in Figure 2. With an increase in the repetition rate from 100 to 2000 Hz, the pump pulse width increased from 8.26 ± 0.22 to 9.80 ± 0.21 ns.

Figure 2 Pump pulse widths of the laser system with different repetition rates.
3 Theoretical analysis
3.1 Thermal problem of high-repetition-rate SBS pulse compression
In the case of the liquid medium, the main issues limiting SBS pulse compression under high-repetition-rate operation and high-pump-power application are the thermal effects and optical breakdown[ Reference Andre, Andreev, Kulagin, Palashov, Pasmanik, Rodchenkov and Powell 26 – Reference Park, Cha, Oh, Lee, Ahn, Churn and Kong 29 ]. The laser absorbed by the medium is converted into heat, causing serious thermal deposition within the medium, an uneven spatial distribution of the medium temperature, significant thermal convection, changes in the medium density gradient, laser-beam deflection and other phenomena, as shown in Figure 3. These phenomena interact with each other, exhibiting strong coupling characteristics, and cause severe beam distortion, affecting the quality of the compressed beam, as shown in the lower left corner of Figure 3. The beam intensity profiles in Figure 3 were measured using the HT110 medium at a repetition rate of 600 Hz, and they exhibit severe optical distortion. In Figure 3, the beam profile inside the amplifier cell was measured by employing another shorter one with equivalent length. With an increase in the laser repetition rate, the thermal absorption is prone to increase the probability of optical breakdown at the focal spot in the SBS generator cell. The optical-breakdown phenomenon is a highly complex plasma formation process caused by avalanche ionization in the medium. The impurity suspended particles in the liquid medium can absorb a large amount of laser energy under a high laser power, which causes a sharp temperature increase. If it is too late for the accumulated heat to diffuse, the energy is deposited continuously, resulting in ionization or micro-explosion, accompanied by flashes and bubbles, as shown in the lower right corner of Figure 3. Optical breakdown can significantly reduce the energy reflectivity, the phase conjugate fidelity and the stability of SBS pulse compression. Therefore, the thermal effects and optical breakdown induced by the laser heating of the liquid medium can limit the high-repetition-rate SBS pulse-compression quality.

Figure 3 Schematic of thermal problems in the process of SBS pulse compression.
At the initial time of the amplifier cell, radiation absorption and heat conduction are the main mechanisms, and the temperature field and radiation field of the medium are radially symmetric around the optical axis. The laser heating effect changes the density of the liquid medium, and the temperature changes with the space along the radial direction. Therefore, the density gradient leads to a spatial change in the liquid buoyancy. The flow of the liquid layer leads to continuous heat diffusion from the beam area into the SBS liquid around the beam spot, and this convective motion leads to the upper and lower asymmetry distribution of temperature in the liquid medium, as shown in Figure 3 (A-A). The intensity of thermal convection in a liquid is affected by the viscosity of the liquid and the buoyancy force generated by laser heating. To suppress thermal convection and obtain good beam patterns, the viscosity force and buoyancy force must be in equilibrium. As the repetition rate increases, the total heat absorbed by the medium increases, increasing the temperature and the buoyancy force. For maintaining an equilibrium state, it is necessary to increase the viscosity force correspondingly, that is, increase the kinematic viscosity coefficient of the medium. SBS liquid media with a large kinematic viscosity coefficient were used in the experiment of the present study. Two dimensionless parameters, the Grashof number (Gr) and the Rayleigh number (Ra), are used to describe the heat transfer form and fluid state of the liquid medium. The heat exchange and flow state of the liquid medium are estimated according to the following three reference data: (1) the physics properties of HT270[ 33 ]; (2) the criterion of dominant heat transfer for conduction and convection is Gr = 2860[ Reference Nepomnyashchy, Legros and Simanovskii 34 ]; (3) the critical value of the Rayleigh number is 108, borrowed from ANSYS-FLUENT software. Based on the spot size measured experimentally, numerical results show that the HT270 liquid medium is dominated by heat conduction at temperatures below 52°C and starts convective heat transfer at temperatures above 52°C, and the flow state is laminar flow below the boiling point.
According to the experimental and theoretical analyses, there are four methods for improving the average injection power of SBS pulse compression. (1) The first method is to remove the large impurity particles in the liquid medium that absorb the radiation. Filtration or distillation can be used to remove the large impurities in the medium and reduce the probability of optical breakdown. (2) To alleviate the heat accumulation near the focal spot in the generation cell, the effective operating repetition rate of SBS pulse compression can be reduced using the rotating off-centered lens method. (3) The third method is to increase the kinematic viscosity coefficient of the liquid medium. An SBS liquid medium with a large kinematic viscosity coefficient can be used to improve the non-uniformity of beam profiles caused by thermal convection in the experiment. (4) The fourth method is to increase the boiling point of the SBS liquid medium. A high local temperature at the focal spot can exceed the boiling point and cause jiggling of the beam pattern, affecting the SBS process.
At room temperature, the kinematic viscosity and boiling point of the Galden HT110 medium are 0.77 cSt and 110°C, respectively, and those of the Galden HT270 medium are 11.7 cSt and 270°C, respectively. The HT270 liquid medium with a large kinematic viscosity and a high boiling point was selected to improve the non-uniformity of beam profiles and avoid the jiggling effect of beam patterns caused by thermal problems for the high-power SBS pulse-compression experiment in the present study.
3.2 Theoretical analysis of SBS output characteristics enhanced by thermal effects
The theoretical model of SBS pulse compression has been described in previous works. The optical field of the input beam E
L and reflected beam E
S and the acoustic field
$\rho$
obey the following three coupled differential equations[6,18], which are solved via the split-step method:



Here,
${\gamma}^{\mathrm{e}}=\left({n}^2 - 1\right)\left({n}^2+2\right)/3$
is the electrostrictive coupling constant, which is determined by the refractive index n; c represents the speed of light;
${\rho}_0$
represents the unperturbed density; and
$\alpha$
is the absorption coefficient.
In high-repetition-rate SBS pulse compression, the temperature of the medium increases with the laser repetition rate; thus, the thermal effects on the output parameters are not negligible. The steady-state Brillouin gain
${g}_{\mathrm{B}}$
[
Reference Park, Lim, Yoshida and Nakatsuka
32
] considering the change in the medium temperature in SBS pulse compression is given as follows:

When the wavelength is determined, the speed of sound
$v$
in the medium can be expressed in terms of the Brillouin frequency shift
${v}_{\mathrm{B}}$
:
$v=\lambda {v}_{\mathrm{B}}/\left[2n\sin \left(\theta /2\right)\right]$
, where
$\theta$
represents the scattering angle. The Brillouin linewidth is expressed as
${\Gamma}_{\mathrm{B}}(T)=\left[4{\pi}^2\eta (T)\right]/{\lambda}^2$
. For the HT270 medium, the kinematic viscosity coefficient
$\eta$
changes significantly with changes in the temperature[
33
]. Thus, the Brillouin gain coefficient
${g}_{\mathrm{B}}(T)$
of the HT270 medium is affected by the medium temperature T. In the present study, the medium density ρ is 1.85 × 103 kg/m3, the refractive index n is 1.283 and the sound speed v is 705 m/s. In this paper, the calculation of the gain coefficient employed the thermal characteristic parameters at room temperature. In fact, the other parameters of the liquid medium except for the kinematic viscosity are temperature-dependent and may affect the performance of SBS pulse compression.
Figure 4 shows the SBS gain coefficient of the HT270 medium with respect to the medium temperature. The black and red curves indicate the kinematic viscosity coefficient and calculated gain coefficient, respectively, with respect to the temperature. The kinematic viscosity coefficient of the HT270 medium decreased with an increase in the temperature, and the phonon lifetime and gain coefficient of the SBS medium were inversely proportional to the kinematic viscosity coefficient. Phonon lifetime is the reciprocal of the Brillouin linewidth of the medium. With an increase in the medium temperature, both the gain coefficient and phonon lifetime of the SBS medium increased, resulting in changes in the SBS output parameters. It can be seen from the theoretical simulation in Figure 4 that the gain coefficient of HT270 medium at 60°C will increase to 7.6 × 10–11 m/W, and the medium at this temperature has a larger gain coefficient compared with the other HT series media reported in the literature at room temperature. According to the coupled differential equation (1), the dependence of the SBS energy efficiency and gain coefficient can be simulated numerically, as shown in Figure 5. In Figure 5, the SBS medium with a larger gain coefficient yielded a higher SBS energy efficiency. The SBS energy efficiency increased with the gain coefficient.

Figure 4 Kinematic viscosity of the HT270 medium and the calculated gain coefficient with respect to the temperature.

Figure 5 Calculated SBS energy efficiency with respect to the gain coefficient.
According to the foregoing theoretical analysis, when the laser repetition rate increases, the total laser energy absorbed by the medium causes the temperature to rise, reducing the kinematic viscosity of the HT270 medium and thus increasing the SBS gain coefficient of the medium. The SBS medium with a larger gain coefficient yields a higher energy efficiency and narrower compression pulse. When the medium gain coefficient is increased sufficiently by the rising temperature, the positive thermal effects on SBS pulse compression outweigh the negative effects. Therefore, in some cases, the thermal effects do not limit the quality of SBS pulse compression and can enhance the output characteristics of SBS pulse compression, for example, increasing the energy efficiency.
4 Experimental results and discussion
To improve the average injection power of SBS pulse compression, the problems of optical breakdown and thermal effects in the SBS cell must be solved. According to the experimental and theoretical analyses, there are three methods for improving the high-repetition-rate operation. (1) The method of ultrafiltration can remove the large impurity particles in the liquid medium that absorb the radiation light in the experiment. (2) The rotating off-centered lens method can alleviate the heat accumulation near the focal spot in the generator cell. (3) An SBS liquid medium with a high kinematic viscosity and high boiling point can be used to improve the non-uniformity of beam profiles caused by thermal convection in the experiment.
4.1 SBS pulse compression based on medium purification
It is generally agreed that the thermal effects mainly arise from the heat converted by the laser absorption of impurity particles in the medium. To remove the impurity particles in the liquid, a vacuum purification system was designed. The liquid successively passed through organic filter membranes with pore sizes of 250 and 25 nm made of cellulose and nitrocellulose, respectively. The membrane with a 250-nm pore size was used to filter large impurity particles in the purification system, and the 25-nm membrane was used to filter small impurity particles in the SBS liquid medium. Because the pore size of the smaller filter membrane was 25 nm, the maximum impurity-particle size in the filtered medium was considered to be 25 nm. Finally, the purified SBS liquid medium was pressed into a glass cell. The output parameters of SBS pulse compression before and after the medium purification were compared.
Using HT270, the SBS output parameters with respect to the input energy were measured before and after medium purification at a repetition rate of 1000 Hz, as shown in Figure 6. The black solid squares and red solid circles represent the experimental results obtained using purified and unpurified media, respectively. Figure 6(a) shows the relationship between the output energy and the input energy. The reflected energy increased with the injected energy, and the output energy for the purified medium was significantly higher than that for the unpurified medium. When the injection energy was 45 mJ, the output energy for the unpurified medium was significantly reduced, owing to the severe optical breakdown at the focal spot in the generator cell. Figure 6(b) shows the relationship between the energy efficiency and the input energy. For the purified medium, the SBS energy efficiency increased with the injection energy, whereas for the unpurified medium, the energy efficiency essentially remained unchanged when the injection energy exceeded 20 mJ, indicating that the efficiency was saturated under the thermal effects. When the injection energy increased to 45 mJ, the reflected energy efficiency decreased sharply, indicating that the nonlinear effect of the optical breakdown suppressed the SBS. As shown in Figure 6(c), the compressed pulse width decreased with an increase in the input energy. The pulse width for the purified medium was smaller than that for the unpurified medium. For the unpurified medium, at 50 mJ, the compressed pulse width was significantly increased compared with the purified case, and the corresponding energy efficiency exhibited a sharp reduction. Figure 6(d) shows the relative standard deviation (RSD) of the compressed pulse width with respect to the injection energy. With an increase in the injection energy, the pulse-width RSD for the unpurified medium increased, whereas that for the purified medium decreased. Clearly, the nonlinear thermal effects and optical breakdown influenced the SBS reflected energy, compressed pulse width and its RSD.

Figure 6 Comparison of SBS output parameters before and after purification of the HT270 medium at a repetition rate of 1 kHz.
The experimental results indicated that the unpurified medium, which caused severe thermal effects and an increase in the optical-breakdown probability, reduced the SBS energy reflectivity, increased the pulse width, and improved the pulse-width RSD. This medium purification method can effectively remove large impurity particles from the SBS liquid medium, reduce the probability of optical breakdown at the beam focal spot, increase the SBS output energy and energy efficiency and reduce the RSD of the compressed pulse width.
4.2 SBS pulse compression based on a rotating off-centered lens
For a high-power and high-repetition-rate laser, the light absorbed by the medium is converted into heat energy at the focal point in the generator cell, increasing the temperature. The very high temperature above the boiling point in the limited space within the medium can cause jiggling of the beam pattern, affecting the SBS process. The absorption of the high-energy laser by microparticles in the liquid medium causes a sharp temperature increase, inducing optical breakdown. The thermal effects and the optical breakdown can significantly reduce the energy reflectivity, the phase conjugate fidelity and the stability of SBS pulse compression. To suppress the optical breakdown and heat accumulation at the beam focal spot, a moving focal spot method, that is, the rotating off-centered lens method, was used.
The variation trends of the SBS output power with respect to the pump power at different repetition rates with and without the rotation of the off-centered lens were compared, as shown in Figure 7. Here, the black squares and red circles represent the experimental results without and with the rotating off-centered lens, respectively. As shown in Figure 7, the SBS output powers increased approximately linearly with growth rates of 0.490, 0.453, 0.514 and 0.633 at the repetition rates of 100, 500, 1000 and 2000 Hz, respectively, with respect to the pump power using the rotating off-centered lens method; the output powers had the growth rates of 0.529, 0.398, 0.475 and 0.690 at the repetition rates of 100, 500, 1000 and 2000 Hz, respectively, using the normal focusing lens. At 500 Hz, the output power with rotation was higher than that without rotation. At 2000 Hz, the output power without rotation was slightly higher than that with rotation.

Figure 7 Measured dependence of the SBS output power on the pump power at repetition rates of (a) 100 Hz, (b) 500 Hz, (c) 1000 Hz and (d) 2000 Hz with and without the rotating off-centered lens.
The relationships between the SBS output energy and the compressed pulse width with respect to the injection pump energy at different repetition rates are shown in Figure 8. At the repetition rate of 500 Hz, the output energies had the growth rates of 0.453 and 0.398 with and without rotation, respectively, with respect to the pump energy, and the output energy with rotation was higher than that without rotation. At 2000 Hz, the output energies increased with the growth rates of 0.621 and 0.686 with and without rotation, respectively, with respect to the pump energy, and the output energy with rotation was lower than that without rotation. For the HT270 medium, with an increase in the repetition rate, the influence of the rotation on the SBS compression pulse width was negligible. At 34.2 mJ and 2000 Hz, the output energy and pulse width of SBS pulse compression without rotation were 16.75 ± 0.22 mJ and 1.09 ± 0.13 ns, respectively, and those with rotation were 16.70 ± 0.35 mJ and 1.12 ± 0.13 ns, respectively. The results indicate that the rotating off-centered lens method did not significantly affect the output parameters of SBS pulse compression at 2000 Hz.
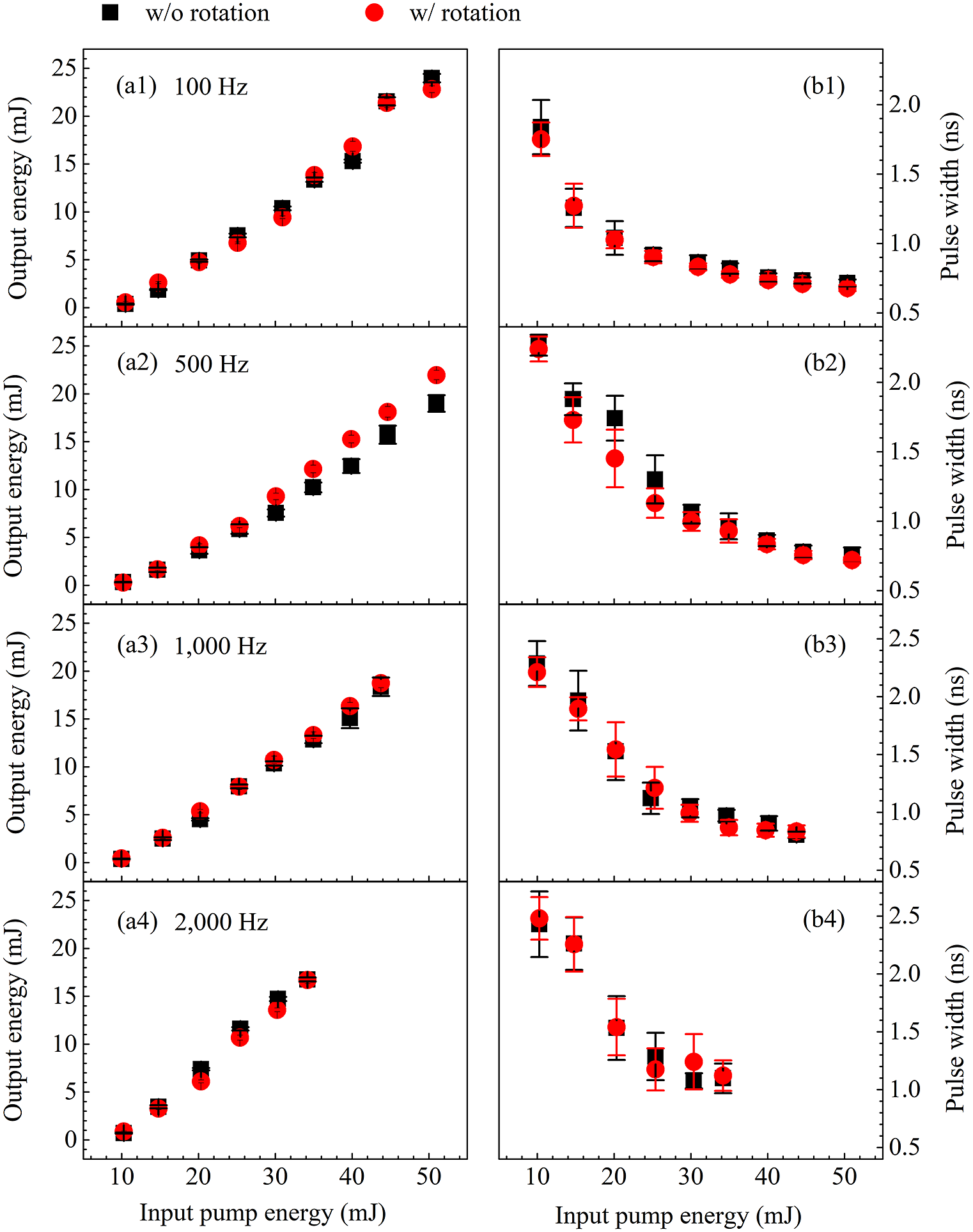
Figure 8 Measured dependences of the SBS (a1)–(a4) output energy and (b1)–(b4) compressed pulse width on the pump energy at different repetition rates with and without the rotating off-centered lens.
The experimental results indicated that with the liquid medium HT270, the rotating off-centered lens method alleviated the focusing thermal effect on the SBS pulse compression and improved the reflected energy at 500 Hz. When the repetition rate increased to 2000 Hz, the rotating method hardly affected the output characteristics of the SBS pulse compression. When the repetition rate increased above 2000 Hz, the rotating off-centered lens method no longer improved the output parameters of the SBS pulse compression.
4.3 Stability measurement of SBS pulse compression
For high-repetition-rate SBS pulse compression, the thermal effects and optical breakdown in the liquid medium suppress the SBS. The rotating off-centered lens method can alleviate the focusing thermal effect in the generator cell, but it also affects the output stability. Therefore, the effect of the rotating off-centered lens on the output stability of SBS pulse compression was experimentally studied. The output stability with the repetition rate of 2000 Hz is shown in Figure 9, where the red and black curves represent the normalized output powers with and without the rotating off-centered lens, respectively.

Figure 9 Stability of the SBS output energy at 2000 Hz with and without the rotating off-centered lens.
With the rotating off-centered lens, the average reflected power and standard deviation within 20 min were 24.24 and 4.57 W, respectively, and the RSD was 18.85%. Without the rotating off-centered lens, the corresponding values were 27.09 and 1.24 W, respectively, and the RSD was 4.58%. As shown in Figure 9, the average reflected power without rotation was higher than that with rotation, and the stability of the output power without rotation was significantly better than that with rotation. It can be inferred that the negative effect of the rotating off-centered lens on the SBS output stability outweighed its mitigation of the focusing thermal effect.
One of the main reasons for the poor stability of the SBS output with the rotating off-centered lens method is the optical breakdown. Laser-induced optical breakdown is a process of multi-photon or avalanche ionization in the medium due to a focused high-energy pulse laser. Numerous ions and free electrons appear near the focus, which destroys the dielectric material. Optical breakdown can occur in liquid media – particularly those containing high-absorption impurity particles. As the repetition rate increases, two phenomena occur when the impurity particles are heated by the laser: (1) an increase in the particle concentration due to thermal convection and (2) optical breakdown. Optical breakdown affects the SBS, reduces the reflected energy and increases the compressed pulse width. Therefore, the occurrence frequency of optical breakdown is an index that must be evaluated for SBS pulse compression.
In the experiment, the occurrence frequency of optical breakdown was measured using the audio frequency, as shown in Figure 10. Figures 10(a) and 10(b) show the audio amplitude over 600 s with and without the rotating off-centered lens, respectively. It can be calculated from Figure 10 that the occurrence probability of optical breakdown with rotation was 5.6 times larger than the case without rotation for a 600-s duration, owing to the impurity-particle size and concentration. A larger impurity-particle size and higher concentration corresponded to a higher optical-breakdown probability. The spatial area of the beam focus with rotation was 4πRω10, and that without rotation was π(ω10)2. In this experiment, R was 6.4 mm, and the focal spot radius ω10 was 0.08 mm. The calculated spatial areas with and without rotation were 6.43 and 0.02 mm2, respectively. Assuming that the number of impurity particles per unit volume was certain, within the same time period, the number of impurity particles encountered by the focal spot with rotation was more than 300 times that without rotation. Therefore, the optical-breakdown probability with rotation was significantly higher than that without rotation. This was the main reason for the poor stability of the SBS output with the rotating off-centered lens.

Figure 10 Audio amplitude of the SBS output (a) with and (b) without the rotating off-centered lens at 2000 Hz.
For the high-repetition-rate laser, the experimental results indicated that the rotating off-centered lens method increased the optical-breakdown probability in the generator cell and reduced the stability of SBS output energy. The negative effect of the rotation on the SBS output characteristics outweighed the mitigation of the focusing thermal effect when the laser repetition rate increased above 2 kHz. If the optical-breakdown problem in the liquid medium can be resolved, the rotating off-centered lens method is also an effective method for alleviating the heat accumulation at focal spot in the generator cell for the high-repetition-rate laser.
4.4 Experimental results with different repetition rates
Using the experimental device shown in Figure 1, the relationship between the SBS energy efficiency and the injection pump energy using a conventional lens was examined, as shown in Figure 11. Under the same pump energy, the SBS energy efficiencies at repetition rates of 100, 1000 and 1500 Hz were similar, whereas the energy efficiency was significantly reduced at 500 Hz and significantly improved at 2000 Hz.

Figure 11 Measured dependence of the SBS energy efficiency on the pump energy at different repetition rates.
To intuitively study the relationships between the SBS output parameters and the repetition rate, the reflected powers, reflected energies, energy efficiencies and compressed pulse widths were measured with respect to the repetition rate at pump energies of 20 and 35 mJ, as shown in Figure 12. Here, the black squares and red circles indicate the SBS output parameters at pump energies of 20 and 35 mJ, respectively. With the increasing repetition rate, the reflected power increased, the reflected energy efficiency first decreased and then increased and the compressed pulse width increased slightly. In Figure 12(a), under the same pump energy, the reflected power increased approximately linearly with the increase of the repetition rate, because the input pump power increased linearly with the repetition rate. The reflected energy and energy efficiency of SBS decreased first and then increased with the increasing repetition rate. In Figure 12(c), at the pump energy of 35 mJ, the energy efficiencies of SBS are 38.0%, 29.3% and 49.0% at repetition rates of 100, 500 and 2000 Hz, respectively. The experimental results showed that the energy efficiency at a 2000-Hz repetition rate was 30% and 70% higher than those at 100 and 500 Hz at a 35-mJ pump energy, respectively. The results indicated that the energy efficiency of SBS pulse compression was improved at the repetition rate of 2000 Hz; thus, the thermal effects enhanced the output parameters of SBS pulse compression, as described in Section 3.2 .

Figure 12 Measured dependences of the SBS (a) reflected power, (b) reflected energy, (c) energy efficiency and (d) compressed pulse width on the repetition rate at pump energies of 20 and 35 mJ.
As the repetition rate increased from 100 to 500 Hz, a large amount of heat accumulated in the liquid medium, causing an uneven distribution of the temperature field and a large density gradient of the medium. The density gradient of the liquid medium generated a refractive-index gradient distribution and caused the thermal defocusing effect of the laser beam. The defocusing effect at 500 Hz yielded a larger focusing spot size than the case at 100 Hz, reducing the power density of the pump light at the focal point and thereby reduced the energy efficiency of the SBS. The resulting thermal defocusing and thermal blooming inhibited the SBS generation, negatively affecting the SBS output characteristics. Therefore, the energy efficiency at the 500-Hz repetition rate was lower than that at 100 Hz.
When the laser repetition rate increased above 1000 Hz, the defocusing effect becomes more serious, which reduces the energy efficiency of SBS pulse compression. However, the thermal effects can also enhance the output characteristics of SBS pulse compression, as described in Section 3.2. For HT270, the medium temperature increased with an increase in the laser repetition rate, leading to a decrease in the viscosity coefficient of the medium. An SBS medium with a smaller viscosity coefficient will have a larger gain coefficient, as shown in Figure 4, thereby improving the SBS energy efficiency according to the simulation of Figure 5. Therefore, as the laser repetition rate increases, the SBS energy efficiency will improve when the heat accumulation changed the medium parameters. At the 2000-Hz repetition rate, the reflected energy efficiency of SBS pulse compression was significantly improved compared with that at lower repetition rates and the same pump energy. There were two reasons for the improvement of the SBS energy efficiency at the high input pump power. The first was the pump pulse width, which increased with the repetition rate. A larger pump pulse width corresponded to a higher SBS energy efficiency. The second reason was the thermal effects on the HT270 medium parameters, in accordance with the theoretical analysis of the SBS output characteristics enhanced by thermal effects presented in Section 3.2. For HT270, the medium temperature increased with an increase in the laser repetition rate, and both the gain coefficient and phonon lifetime of the SBS medium increased with the temperature. The SBS energy efficiency improved with an increase in the gain coefficient. At the 2000-Hz repetition rate, the enhancement of the SBS pulse-compression output characteristics due to the thermal effects outweighed the negative effect of heat accumulation based on the HT270 medium. The thermal effects enhanced the output characteristics of SBS pulse compression by changing the medium properties through heat accumulation.
Figure 13 shows the waveforms and beam intensity profiles of the pump and SBS compressed pulses at a pump power of 70 W. A 10-ns pulse was compressed to 962 ns under a 35-mJ pump energy at 2000 Hz. A sub-nanosecond SBS pulse compression without beam pattern distortion was obtained at a 2000 Hz repetition rate in the liquid medium. Under high-power laser thermal effects enhancing the SBS pulse-compression characteristics, if the pump energy is further increased, the reflected power of SBS pulse compression may be further increased.

Figure 13 Waveforms and beam patterns of (a) the pump and (b) SBS compressed pulse at a pump power of 70 W.
5 Conclusion
With the HT270 liquid medium, the output characteristics of high-repetition-rate SBS pulse compression based on the medium purification and rotating off-centered lens methods were experimentally studied, and a sub-nanosecond-pulse laser output with a 2000-Hz repetition rate was obtained. At a repetition rate of 500 Hz, the rotating off-centered lens method alleviated the focusing thermal effects and improved the output energy efficiency of SBS pulse compression. When the repetition rate increased above 2000 Hz, the energy stability of the SBS output was reduced owing to the severe optical breakdown under the rotating off-centered lens method. At a pump energy of 35 mJ, the energy efficiency of SBS pulse compression was approximately 49% at the repetition rate of 2000 Hz without using the rotating method, which was 30% and 70% higher than those at 100 and 500 Hz, respectively. There were two main reasons for the improvement of the SBS energy efficiency at 2000 Hz: the increased pump pulse width and the thermal effects on the HT270 medium parameters. With the increase in the repetition rate, the temperature of the local medium increased, reducing the kinematic viscosity of the HT270 medium and increasing the gain coefficient of the medium. The SBS medium with a larger gain coefficient yielded a higher energy efficiency and a narrower compression pulse. The experiments and theoretical analyses indicated that for the HT270 medium, the thermal effects can enhance the output characteristics of high-power SBS pulse compression in some cases. The experimental results provide design guidance for high-power SBS technology. For example, the medium parameters can be changed to optimize the output characteristics of SBS pulse compression by controlling the medium temperature. In addition, only the dependence of the viscosity coefficient on temperature was considered in the simulation of the gain coefficient, and other temperature-dependent parameters of the refractive index and density need to be considered for a more complete physical model in the future.
Acknowledgments
This research was supported by the National Natural Science Foundation of China (No. 62105303), the Shanxi Scholarship Council of China (No. 2020-102), the Fundamental Research Program of Shanxi Province (No. 20210302124026), the Scientific and Technological Innovation Programs in Shanxi (No. 2020L0265), and the 2021 China-Korea Young Scientist Exchange Program.