- BA
-
bile acid
- LPS
-
lipopolysaccharide
- SRB
-
sulphate-reducing bacteria
- TMA
-
trimethylamine
- TMAO
-
trimethylamine-N-oxide
CVD is mediated by different risk factors both modifiable and non-modifiable. Age, sex and genetics, or particular genotypes (e.g. apoE4), represent non-modifiable risk factors which impact directly on an individual's likelihood of developing CVD. CVD risk is also shaped by a number modifiable largely environmental risk factors often linked to diet and lifestyle, e.g. smoking, chronic low-grade systemic inflammation (sometimes called metabolic endotoxemia), dyslipidaemia, high blood pressure, diabetes and insulin resistance, metabolic syndrome, overweight/obesity( Reference Gensini, Comeglio and Colella 1 , Reference Negi and Anand 2 ). Recent studies in animal models and in human subjects have identified another extra-genomic contributor to CVD risk, the gut microbiota. Aberrant gut microbiota profiles have been associated with obesity, type 1 and type 2 diabetes, non-alcoholic fatty liver disease, certain cancers and various autoimmune diseases( Reference Ley, Turnbaugh and Klein 3 – Reference Markle, Frank and Mortin-Toth 10 ). Recent experimental studies have shown that transfer of faecal microbiota can induce metabolic disease and obesity, indicating that the gut microbiota possess an intricate relationship with mammalian physiological processes linked to CVD risk. In a paradigm-shifting experiment, Bäckhed et al.( Reference Bäckhed, Ding and Wang 11 ) showed that the obese phenotype, and associated insulin resistance and inflammatory profiles could be transferred along with faecal microbiota from obese animals to lean germ-free counterparts. More recently, Vrieze et al.( Reference Vrieze, Van Nood and Holleman 12 ) showed that faecal transfer from lean healthy human donors to male metabolic syndrome subjects could increase insulin sensitivity 6 weeks after the transfer and also increase the levels of butyrate-producing intestinal bacteria. Such intriguing experiments illustrate clearly that the gut microbiota may at once contribute to CVD risk and also represent a realistic therapeutic target( Reference Wang, Klipfell and Bennett 13 – Reference Conterno, Fava and Viola 15 ).
Diet appears to critically influence both the relative abundance of different gut microorganisms and their metabolic output. Animal and a limited number of human studies have shown that diets high in fat or animal protein can radically remodel the gut microbiota, reducing the relative abundance of bifidobacteria and butyrate-producing bacteria, considered beneficial to health, and increasing the concentration of harmful microbially derived metabolites( Reference Wang, Klipfell and Bennett 13 – Reference Fava, Gitau and Griffin 18 ). On the contrary, other studies have shown that supplementing high-fat diets with fermentable fibres or prebiotics can increase the relative abundance of bifidobacteria and butyrate-producing bacteria and SCFA production, all seen as beneficial activities( Reference Cani, Neyrinck and Fava 19 – Reference Wolever 23 ). These fermentable fibres and prebiotics, including inulin, oligofructose, β-glucan, resistant starch and pectin, have long been studied in animal models for their ability to reduce insulin resistance, plasma cholesterol levels and arteriosclerotic plaques( Reference Wolever 23 – Reference Fava, Lovegrove and Gitau 26 ). This work is also supported by epidemiological data demonstrating an inverse association between the consumption of dietary fibre and CVD risk( Reference Toeller, Buyken and Heitkamp 27 , Reference Ludwig, Pereira and Kroenke 28 ). Moreover, recent human dietary interventions have shown that with carful choice of probiotic strain, it is possible to reduce blood cholesterol, an indirect marker of CVD risk, by augmenting microbial involvement in the enterohepatic circulation of bile acids (BA) and reducing fat absorption from the intestine( Reference Jones, Martoni and Parent 29 , Reference Jones, Martoni and Prakash 30 ).
An anthropology of diet: microbe interactions within the human gut
The human intestinal tract is colonised by a microbiota of some 1000 different microbial species. Each microorganism within the gut encodes unique metabolic functions which taken together comprise the gut microbiome. Indeed, the metabolic potential of the gut metagenome, or the sum of microbial encoded genes is about 100-fold that of the human genome, and even with functional redundancy between different microbial species, represents a metabolic potential rivaling that of the liver both in chemical diversity and impact on host health( Reference Tuohy, Gougoulias and Shen 31 – Reference Lepage, Leclerc and Joossens 33 ). The close association between host physiology and this diverse intestinal microbiota is clearly demonstrated by the critical gut microbiota contribution to the establishment and maintenance of a tolerogenic and well functioning immune system, a process greatly impacted by both diet and microbial passengers in the diet( Reference Ouwehand, Isolauri and Salminen 34 – Reference Hand and Belkaid 37 ). However, signals between the gut microbiota and human immune system too can get lost in translation leading to the activation of pathological processes. Certain microbial proteins for example share the considerable structural homology to human proteins including leptin and gut satiety hormones involved in controlling food intake and to which autoantibodies are raised in metabolic disease( Reference Zhou, Ten and Sinha 38 – Reference Proal, Albert and Marshall 42 ). Such observations raise the intriguing possibility that aberrant gut microbiota profiles may be more than coincidental consequences of poor diet, but may actually play a pathological role in these chronic diet-associated diseases.
The human genome has evolved closely with its microbial counterpart over the course of evolution, resulting in many shared or co-metabolic pathways. Through these shared metabolic pathways the mammalian host derives energy and nutrients from its diet which otherwise would be lost in faeces. For example, many complex plant polysaccharides require the activities of both human encoded enzymes and microbial enzymes in the colon to release sugar monomers and SCFA later upon fermentation( Reference Gill, Pop and Deboy 43 ). Similarly, up to 95 % of plant polyphenols escape digestion in the upper gut and reach the colon where they are transformed by the resident microbiota into biological available and often biologically active intermediates( Reference Fava, Lovegrove and Gitau 26 , Reference Clifford 44 , Reference Lu, Xiao and Zhang 45 ). In addition, the gut microbiota also plays a critical role in the enterohepatic circulation of BA, a process closely linked to circulating cholesterol levels.
For the vast majority of evolutionary time, human subjects existed as hunter gatherers, collecting diverse wild species of fruit, vegetables, seeds and root tubers rich in starch, inulin and other complex polysaccharides, and hunting wild game( Reference Tuohy, Gougoulias and Shen 31 ). This ancient diet, close to extant traditional diets based on high intake of whole-plant foods, e.g. the Mediterranean diet, is radically different from the current prevailing Western-style diet characterised by foods rich in fat, animal protein, flavouring agents, particularly sugars, salt and monosodium glutamate, and low in fibre, plant phytochemicals, beneficial fats, minerals and vitamins. Considering the ability of plant polyphenols to inhibit certain gut bacteria and stimulate the growth of others( Reference Tzounis, Rodriguez-Mateos and Vulevic 46 , Reference Queipo-Ortuño, Boto-Ordóñez and Murri 47 ), the ability of these same antioxidant polyphenols to alter redox potential within the gastrointestinal tract thereby reducing the absorption of oxidised cholesterol species( Reference Natella, Macone and Ramberti 48 ), and the key role of fermentable fibres/prebiotics in shaping the composition and metabolic activity of the gut microbiota( Reference Tuohy, Brown and Klinder 22 , Reference Tuohy, Gougoulias and Shen 31 , Reference Petschow, Doré and Hibberd 49 ), it is no wonder that the chronic diet-associated diseases, both metabolic and autoimmune in nature which are reaching epidemic proportions in the developed world are virtually unheard of in populations following these more traditional diets in diverse regions of the world( Reference Willcox, Willcox and Todoriki 50 – Reference Moore and Moore 54 ). In a study using 16S rRNA-based metagenomics to characterise the composition and metabolic output of the faecal microbiota from children growing up in urban Florence, Italy and rural Burkina Faso in Africa it was found that the profile of bacteria was strikingly different between healthy, age-matched children growing up in the two very different settings( Reference De Filippo, Cavalieri and Di Paola 55 ). In the African children, following a typical traditional rural African diet consisting principally of whole-plant foods, cereals and fermented fruits, supplemented very occasionally with bush meat and insect protein, a gut microbiota dominated by Bacteroidetes phylum and especially the Prevotella group, indicative of high capacity for carbohydrate fermentation was observed. Conversely, the gut microbiota of the Italian children following a fairly typical Western-style diet, was dominated by Firmicutes and enriched with γ-Proteobacteria. This modulation of Bacteroitedes: Firmicutes ratio was described earlier in obese human subjects and animal models of obesity, with the obese having higher proportions of Firmicutes. The Gram-negative γ-Proteobacteria enriched in the Italian children included Escherichia coli, Salmonella, Shigella and Klebsiella, bacteria which include some of the most common human gastrointestinal pathogens. In addition, the African children appeared to have enhanced production of SCFA, with about 3-fold the concentration of acetate, butyrate and propionate in their faeces compared with the Italian children. Although SCFA concentrations in faeces are poor indicators of SCFA production in the gut, their potential to modulate disease mechanisms linked to CVD are well reported( Reference Conterno, Fava and Viola 15 , Reference Schwiertz, Taras and Schäfer 56 – Reference Teixeira, Grześkowiak and Franceschini 59 ). Similar results were observed by Yatsunenko et al.( Reference Yatsunenko, Rey and Manary 60 ) who studied the effect of age and geography on the faecal microbiota of populations in the USA, Amazonia and Malawai. These authors confirmed the dominance of bifidobacteria within the infant gut microbiota irrespective of geography, the trade-off between Bacteroides and Prevotella within the phylum Bacteroidetes in Westerners compared with traditional populations, and that the Westernised-type microbiota was enriched for simple sugar and protein metabolism in both infants and adults.
Consumption of whole-plant foods, fruit, vegetables and whole-grain cereals has long been at the core of healthy eating guidelines. Current recommendations in the USA encourage consumers to ‘increase vegetable and fruit intake’, and to ‘consume at least half of all grains as whole grains. Increase whole-grain intake by replacing refined grains with whole grains’( 61 ). In the UK, the Food Standards Agency ‘Eatwell Plate’ states that ‘starchy foods such as bread, cereals, rice, pasta and potatoes are a really important part of a healthy diet. Try to choose whole-grain varieties whenever you can’, and to eat ‘plenty of fruit and vegetables’( 62 ). Much of the evidence for these dietary guidelines comes from large-scale epidemiological studies and recent metanalyses of intervention studies showing that both food groups, fruit and vegetables and whole-grain cereals, are inversely associated with CVD risk( Reference Jacobs, Meyer and Kushi 63 – Reference Koteish and Mae Diehl 98 ). However, many of these foods, and their functional molecular components, especially fermentable fibres and polyphenolic compounds have recently been shown to modulate the human gut microbiota towards a more health-promoting profile. Fermentable fibres, especially the prebiotics, increase the relative abundance of bifidobacteria, lactobacilli and butyrate-producing bacteria, all considered beneficial bacteria. Many have anti-inflammatory properties, some have been shown to improve gut wall barrier function and many also possess bile salt hydrolase activity with the ability to impact on BA signalling both within the gut and systemically. Fermentation of these compounds also leads to the production of SCFA, which as discussed later, impact on important physiological processes linked to CVD. The 95% of dietary polyphenols, which reach the colon and become available for microbial transformation represent a chemically diverse class of food molecules with potential to impact on CVD. While many studies have focused on the ability of the specific plant polyphenols to reduce CVD risk by impacting inflammation, oxidative stress, cholesterol/BA binding, platelet function, vasodilation, foam cell formation, cholesterol and glucose homoeostasis and thermogenesis, a few have examined the impact of the microbial catabolites of these plant polyphenols. This is an important distinction, as most plant polyphenols are not absorbed and it is their microbial catabolites which have potential to act systemically( Reference Fava, Lovegrove and Gitau 26 , Reference Del Rio, Rodriguez-Mateos and Spencer 70 ). Interestingly, certain whole fruits and polyphenol-rich fruit extracts have also been recently shown to increase the relative abundance of bifidobacteria, lactobacilli and butyrate-producing bacteria within the human gut microbiota( Reference Tzounis, Rodriguez-Mateos and Vulevic 46 , Reference Queipo-Ortuño, Boto-Ordóñez and Murri 47 , Reference Costabile, Klinder and Fava 71 ). However, a detailed description of these polyphenol-related activities is beyond the scope of this present review but has been discussed elsewhere( Reference Clifford 44 , Reference Del Rio, Rodriguez-Mateos and Spencer 70 ). It is worth noting though, that polyphenols and fermentable fibres as they occur in whole-plant foods may act in tandem or even synergistically often through the gut microbiota to improve different physiological processes linked to CVD risk and that the biological activity of whole-plant foods appears to be greater than the sum of their biologically active components.
Diet can bring out the best of microbial behaviours: microbiome symbiosis
Microorganisms within the human intestine ferment carbohydrate sources, which reach the colon into the SCFA acetate, propionate and butyrate( Reference Conterno, Fava and Viola 15 ). These small organic acids have been shown in animal studies to regulate incretin or gut hormone production, thereby controlling satiety and food intake( Reference Conterno, Fava and Viola 15 , Reference Lin, Frassetto and Kowalik 72 , Reference Cani, Possemiers and Van de Wiele 74 ). They also stimulate the gut hormone glucagon-like peptide-2, which is involved in maintaining gut barrier function, a defense mechanism, which can impede the uptake of inflammatory compounds such as lipopolysaccharide (LPS) from the gut lumen that trigger the low-grade chronic inflammation and subsequent insulin resistance associated with obesity and CVD( Reference Tappenden, Albin and Bartholome 73 , Reference Cani, Possemiers and Van de Wiele 74 ). Similarly, SCFA have been shown to regulate adipocyte hormone production, not least of leptin( Reference Xiong, Miyamoto and Shibata 75 ), the obesity hormone, and to control inflammatory processes in adipose tissue( Reference Al-Lahham, Roelofsen and Rezaee 76 ), processes intimately involved in CVD risk, and possibly also impact on the way energy is stored or burnt in the body controlling adiposity and thermogenesis( Reference Conterno, Fava and Viola 15 , Reference Gao, Yin and Zhang 77 ).
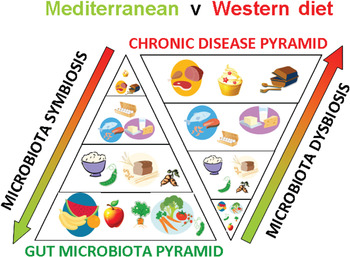
Fig. 1. (colour online) A schematic representation of how diet shapes the human gut microbiota and impacts on chronic disease risk.
Microbial deconjugation of BA and the enterohepatic circulation of BA is a core activity of the human gut microbiota and is thought to directly regulate cholesterol levels in the blood( Reference Jones, Begley and Hill 78 , Reference Jones, Tomaro-Duchesneau and Martoni 79 ). Conjugated BA are secreted into the small intestine to aid micelle formation and fat absorption. About 5 % of BA may pass to the distal ileum and colon where they undergo deconjugation by the gut microbiota reducing their absorbability and increasing their loss in faeces. Since BA synthesis from cholesterol is under tight control in the liver, BA lost in faeces must be replaced driving hepatic uptake and metabolism of circulating cholesterol( Reference Charach, Grosskopf and Rabinovich 80 , Reference Matsubara, Li and Gonzalez 81 ). Indeed, increased loss of BA or cholesterol in faeces has long been suggested as a mode of action of dietary fibres and whole-plant foods in lowering blood cholesterol( Reference Kay and Truswell 82 – Reference Sembries, Dongowski and Mehrländer 84 ). However, more recent studies are suggesting that this may not be the whole picture. Intriguingly, deconjugated BA may also be absorbed( Reference Jones, Martoni and Prakash 30 ). BA and deconjugated BA are emerging as important cell-signalling molecules, interacting with nuclear receptors such as Farnesoid X receptor (FXR), Pregnane X receptor (PXR) and Peroxisome proliferator-activated receptor alpha (PPARα) and G-protein-coupled receptors such as TGR-5, which regulate inflammation, xenobiotic detoxification, lipid metabolism, cholesterol biosynthesis and uptake, BA metabolism, glucose homoeostasis and thermogenesis( Reference Matsubara, Li and Gonzalez 81 , Reference Cyphert, Ge and Kohan 85 – Reference Sayin, Wahlström and Felin 88 ). However, the specific mechanistic studies in animals and human dietary interventions including analysis of the gut microbiota and their metabolic activities with foods capable of modulating the enterohepatic circulation of BA (e.g. probiotics, prebiotics, polyphenols and whole-plant foods) are needed to confirm which gut bacteria are involved in BA metabolism and how their modulation through dietary means alters BA profiles entering the enterohepatic circulation and any subsequent impact on CVD risk.
Other metabolites derived from microbial metabolism in the gut have also been shown to reduce the risk of metabolic disease, at least in animal studies. The neurotransmitter γ-aminobutyric acid is also produced by gut bacteria including certain lactic acid bacteria( Reference Barrett, Ross and O'Toole 89 ). Gamma-Aminobutyric acid given orally is also a strong regulator of inflammation and immune function, and has been shown to reduce the risk of metabolic disease by improving insulin sensitivity through reduced inflammation in laboratory animals on a high-fat, obesogenic diet( Reference Tian, Dang and Yong 90 ). Certain gut bacteria, most notably the bifidobacteria, have been shown to produce folate, a key metabolite C1 metabolism which lowers circulating levels of homocysteine, an independent risk factor of CVD( Reference D'Aimmo, Mattarelli and Biavati 91 ). Animal studies have shown that feeding folate-producing bifidobacteria can increase plasma folate concentrations, and that co-administration of the Bifidobacterium strain with a substrate for its growth within the intestine, the prebiotic inulin, can increase plasma folate concentrations further in a synergistic manner( Reference Pompei, Cordisco and Amaretti 92 ). Gut bacteria may also interact with dietary fats. We have shown that both the quantity and type of dietary fat can reshape the microbial community structure within the gut( Reference Cani, Amar and Iglesias 16 , Reference Fava, Gitau and Griffin 18 ). Conversely, it has been shown in a study at Teagasc in Ireland that bifidobacteria capable of biohydrogenation of fatty acids, when administered to pigs or mice along with α-linoleic acid can increase concentrations of beneficial fats, EPA, DHA and conjugated linoleic acid concentrations in extra-intestinal organs including adipose tissue, liver and brain( Reference Wall, Ross and Shanahan 93 , Reference Wall, Ross and Shanahan 94 ), indicating that at least certain bifidobacterial strains can alter dietary fats in vivo, and increase body stores of beneficial fats. Conjugated linoleic acid, EPA and DHA have long been studied for anti-inflammatory properties and association with reduced risk of CVD and other metabolic diseases( Reference Tricon, Burdge and Williams 95 , Reference Pfeuffer, Fielitz and Laue 96 ) (Fig. 1).
Diet can bring out the worst of microbial behaviours: microbiome dysbiosis
Choline-deficient diets have long been used to induce insulin resistance and metabolic disease in animal models and the gut microbiota is recognised to play an important role in the co-metabolic processing of choline and phosphatidylcholine in man( Reference al-Waiz, Mikov and Mitchell 97 , Reference Koteish and Mae Diehl 98 ). Dumas et al.( Reference Dumas, Barton and Toye 99 ) investigated the impact of choline metabolism disruption on the development of metabolic disease and non-alcoholic fatty liver disease in mice prone to disease (129S6) and in mice resistant to disease (BALBc) using NMR-based metabonomics. These investigators found that low plasma levels of phosphatidylcholine and increased urinary excretion of methylamines (dimethylamine, trimethylamine (TMA) and trimethylamine-N-oxide, (TMAO)) was associated with insulin resistance and fatty liver phenotype. Importantly these metabolites result from host:microbiota co-metabolic processing of choline and phosphatidylcholine( Reference al-Waiz, Mikov and Mitchell 97 ). Dumas et al.( Reference Dumas, Barton and Toye 99 ) demonstrated that microbial conversion of choline into methylamines is induced by high-fat feeding and mimics the disease effects of choline-deficient diets. Other studies have shown that high-fat, low fermentable fibre diets, typically used to induce metabolic disease in laboratory animals decimate the gut microbiota. Cani et al.( Reference Cani, Amar and Iglesias 16 ) showed that high-fat, low fermentable fibre feeding leads to significant die off in dominant and putatively beneficial gut bacteria including bifidobacteria, butyrate-producing Firmicutes and polysaccharide degrading Bacteroidetes. Interestingly, high-fat feeding did not change numbers of Gram-negative sulphate-reducing bacteria (SRB) in these experiments. Decimation of beneficial gut bacteria was accompanied by translocation of bacterial cell wall LPS across the gut wall, either through increased permeability due to reduced tight junction control or carried with fat absorbed from the gut. LPS is an inflammatory cell wall constituent of Gram-negative bacteria and triggers inflammatory cascade through Toll-like receptor-4 or CD14, and NF-κB, and in the high-fat-fed animals, plasma LPS triggers low-grade chronic inflammation and subsequent insulin resistance and body weight gain( Reference Cani, Amar and Iglesias 16 ). Interestingly, Zhang et al.( Reference Zhang, Zhang and Wang 17 ) more recently showed that in another murine model of metabolic disease (ApoA-I knockout mice on high-fat diet), the SRB, Desulfovibrionaceae, showed higher relative abundance in animals with insulin resistance, especially insulin-resistant animals fed high-fat diets. In this study too, numbers of bifidobacteria were decimated by high-fat feeding. SRB, which convert mainly dietary sulphate to H2S, are considered to play an important role in human H2S metabolism( Reference Deplancke, Finster and Graham 100 , Reference Carbonero, Benefiel and Gaskins 101 ), have been implicated in inflammatory bowel disease and their LPS is highly inflammatory. More recently, in common with certain other gut bacteria, SRB have also been shown to be capable of converting choline to TMA under anaerobic conditions, using a glycyl radical enzyme( Reference Craciun and Balskus 102 ). TMA in human subjects is further converted into TMAO by the hepatic enzyme flavin-dependent monooxygenase 3. Interestingly, TMA, the microbial precursor of TMAO, also acts as a substrate for methanogenesis, being metabolised to inoxious CH4, raising the intriguing possibility that modulation of the relative abundance of methanogens:SRB within the human gut might regulate the production of cardiotoxic TMAO or inoxious CH4 gas. The relative proportions and activity of methanogens:SRB have long been of interest but mainly in relation to explaining H2 disposal( Reference Gibson, Cummings and Macfarlane 103 ). Interestingly, Fernandes et al.( Reference Fernandes, Wang and Su 104 ) have recently confirmed that methane production and carriage of detectable Archaea (mainly methanogens in human subjects) are inversely related to BMI and that methanogenesis appears to allow more efficient production and absorption of SCFA from dietary fibre fermentation. While the ecological processes regulating the relative abundance of gut SRB and methanogens are not fully understood, ingestion of fermentable fibre increases methanogenesis in CH4-producing subjects, while sulphate or dietary protein appear to stimulate the numbers of SRB and presumably their metabolic activities within the gut microbiota( Reference Christl, Gibson and Cummings 105 – Reference Khalil, Walton and Gibson 109 ).
In a large clinical cohort, Wang et al.( Reference Wang, Klipfell and Bennett 13 ) showed in 2011 that plasma concentrations of choline, TMAO and betaine, all metabolites of phosphatidylcholine metabolism, are predictive of CVD. While all three up-regulated multiple macrophage scavenger receptors, choline and TMAO feeding induced arteriosclerosis in mice. Moreover, the authors confirmed the essential role of the gut microbiota in TMAO production from dietary choline in germ-free animals, while broad spectrum antibiotics lowered macrophage cholesterol accumulation, foam cell formation and aortic lesions in high-fat-fed conventional animals. Koeth et al.( Reference Koeth, Wang and Levison 14 ) more recently showed that another compound, l-carnitine with a TMA structure similar to that of choline and commonly found in meat, may also be converted to TMA by the gut microbiota and contribute to the increased CVD risk associated with high red meat consumption( Reference Bernstein, Sun and Hu 110 , Reference Micha, Michas and Mozaffarian 111 ). They confirmed microbial involvement in TMA(O) formation from dietary l-carnitine using a stable isotope dilution method and broad spectrum antibiotic microbiota suppression in five healthy omnivorous subjects and in germ-free and antibiotic-treated animals. Conversion of dietary l-carnitine to TMAO did not appear to occur in long-term (>1 year) vegans or vegetarians, suggesting an adoptive response of the gut microbiota in omnivores to TMA production from l-carnitine derived from red meat. Compared with the vegans and vegetarians, omnivores had elevated plasma TMAO concentrations and significantly higher relative abundance of faecal clostridia and peptostreptococci, groups of bacteria commonly associated with protein fermentation. Furthermore, the authors confirmed the direct and dose-dependent association between fasting plasma l-carnitine levels and prevalent coronary artery disease in a large cohort of elective cardiac evaluation subjects (n 2595) and elevated l-carnitine was significantly associated with risk of major adverse cardiac events. When corrected for plasma TMAO these associations disappeared suggesting that TMAO mediated disease risk rather than l-carnitine itself. TMAO also impacted on BA metabolism in the liver at multiple levels including cholesterol transporters and suppression of BA synthetic enzymes Cyp7a1 and Cyp27a1 and animals fed TMAO had a significantly lower BA pool compared with those fed standard rodent chow.
These studies have provided a clear pathological mechanism involving the gut microbiota: mammalian co-metabolic processing of choline, phosphatidylcholine and l-carnitine which appears responsive to red meat consumption but which is much reduced or absent in vegetarians on high-fibre diets. This novel mechanism also fits neatly into existing data from epideamiological studies implicating red meat consumption in CVD risk, and whole-plant food or fibre with low risk of CVD. It also fits with the important role of the enterohepatic circulation of BA in regulating cholesterol metabolism and CVD risk via the activities of nuclear receptor FXRα and G-protein-coupled receptor TGR-5 (Fig. 1).
Reducing CVD risk with probiotics
Probiotics are defined as ‘live microorganisms which when administered in adequate amount confer a health benefit on the host’ and represent a very direct means of modulating the composition of the gut microbiota by adding exogenous microorganisms( 112 ). The most common probiotics are lactobacilli and bifidobacteria( Reference Tuohy, Probert and Smejkal 113 ). The notion that probiotics may impact on CVD risk stems from early suggestions that yoghurt consumption may reduce blood cholesterol levels in nomadic dairying peoples( Reference Hepner, Fried and St Jeor 114 , Reference Mann, Spoerry and Gray 115 ). Some studies from the 1970s and 1980s found that cholesterol levels could be reduced following consumption of large volumes of yoghurt or fermented milk( Reference Hepner, Fried and St Jeor 114 – Reference Rossouw, Burger and Van der Vyver 116 ). For example, Mann et al.( Reference Mann, Spoerry and Gray 115 ) found that massive (4 l/d) consumption of milk fermented with a ‘wild’ strain of Lactobacillus, resulted in a significant reduction in serum cholesterol in twenty-four Maasai warriors. However, many of these early studies used milk or pasteurised yoghurt as control, confounding interpretation and also, did not characterise the microorganisms used to ferment the milk to any great extent. Similarly, studies were often conducted in healthy subjects within normal cholesterol ranges who do not alter the cholesterol levels readily in response to dietary change.
Later studies in the 1990s began to recognise that milks fermented with different lactic acid bacteria may elicit different effects (Table 1). For example, Andersson and Gilliland( Reference Anderson and Gilliland 117 ) found a significant reduction in total cholesterol in fourteen hypercholesterolaemic subjects when consuming Lactobacillus acidophilus L1, a human-derived strain, compared with L. acidophilus ATCC 43121, isolated from the porcine gut. Agerholm-Larsen et al.( Reference Agerholm-Larsen, Bell and Grunwald 124 ) reported a metanalysis of studies using the probiotic fermented milk Gaio®, containing Enterococcus faecium (with the potential probiotic activity) and two strains of Streptococcus thermophilus for milk acidification. From six studies using this product, the authors concluded that short-term intake (4–8 weeks) of this fermented milk produced significant lowering of total and LDL-cholesterol (by 4 and 5%, respectively) but that further studies were required to confirm any long-term effects. Hlivak et al.( Reference Hlivak, Odraska and Ferencik 125 ) more recently, using another strain of Enterococcus faecium, M-74 in combination with selenium, found a 12% reduction in serum cholesterol after a 1-year intervention in forty-three volunteers who consumed the probotic or placebo. Interestingly, not only is this one of the few long-term studies to confirm the cholesterol-lowering effects of probiotics, but it was also conducted with the probiotic in lyophilised powder form delivered in a capsule containing 2×109 colony-forming units of E. faecium M-74. The test product however, also contained 50 mg/dose of organically bound selenium, which may independently lower serum cholesterol( Reference Rees, Hartley and Day 126 ). However, many other early studies have also reported no effect of probiotic intervention in lowering serum cholesterol levels. In general, early probiotic studies have rarely been conducted in large cohorts presumably because of costs associated with culture-based faecal microbiology, and seldom reached the statistical power necessary to adequately test the cholesterol-lowering potential of even the most promising strains( Reference Jackson and Lovegrove 127 ). Moreover, it has become apparent that probiotics or probiotic effects are strain specific with particular probiotic health effects, e.g. immune modulation, production of antimicrobial compounds or the ability to lower cholesterol being present in one strain and absent in another strain belonging even to the same species. A number of mechanisms have been proposed including binding of cholesterol within the gut lumen or incorporation of cholesterol into bacterial cell walls, production of SCFA which might modulate cholesterol synthesis in the liver or increased deconjugation of BA impacting on cholesterol absorption or increasing faecal excretion of bile driving hepatic BA synthesis from circulating cholesterol( Reference Pereira and Gibson 128 ). Although a few of these putative mechanisms have been shown in human subjects, they have been used in vitro as screening tools in an attempt to improve probiotic efficacy. Jones et al.( Reference Jones, Martoni and Parent 29 ) investigated the ability of a Lactobacilus reuteri strain NCIMB 30242 microencapsulated in a yoghurt formulation to reduce the cholesterol levels in hypercholesterolaemic individuals (n 114). Importantly, this strain had been selected as a putative cholesterol-lowering probiotic because of its bile salt hydrolase activity. These authors found that consuming the yoghurt containing the microencapsulated probiotic L. reuteri NCIMB 30242 twice daily for 6 weeks with a combined daily dose of about 1011 viable bacteria, decreased serum total cholesterol and LDL-cholesterol by 4·81 and 8·92%, respectively, compared with the placebo. Non-HDL cholesterol and apoB-100 were also significantly reduced compared with the placebo group. In a second study, this time using the same strain in the lyophylised form, the authors confirmed the cholesterol-lowering ability in 127 hypercholesterolaemic individuals( Reference Jones, Martoni and Prakash 30 ). Upon probiotic supplementation for 9 weeks, LDL-cholesterol, total cholesterol, non-HDL cholesterol and apoB-100 were significantly reduced by 11·64, 9·14, 11·30 and 8·41%, respectively. Moreover, the subjects receiving the probiotic had lower serum levels of plant sterols, a surrogate marker for cholesterol absorption and higher plasma levels of deconjugated BA, leading the authors to suggest a novel cholesterol-lowering mechanism for this strain through reduced cholesterol absorption via the action of deconjugated BA on hepatic FXRα, a nuclear receptor responsible for controlling lipid absorption from the gut by regulating the expression of lipid transporters on the gut wall. Thus it appears that by right choice of probiotic strain it may be possible to lower CVD risk through improved profiles of disease biomarkers especially plasma cholesterol levels.
Table 1. Selected studies where recognised probiotic strains, delivered in fermented milk/yoghurt or pure form, have been investigated for cholesterol-lowering effects in hypercholesterolaemic individuals (after( Reference Jackson and Lovegrove 127 ))
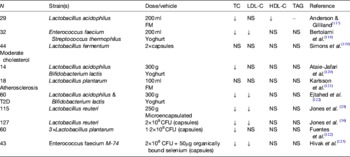
CFU, colony forming units; FM, fermented milk; TC, total cholesterol; T2D, type 2 diabetes.
Reducing CVD risk with prebiotics
A certain class of fermentable carbohydrates has been identified as of particular interest for their ability to modulate the relative abundance of gut bacteria considered beneficial for human health. Prebiotics are selectively fermented ingredients that result in specific changes in the composition and/or activity of the gastrointestinal microbiota, thus conferring benefit(s) upon host health( Reference Petschow, Doré and Hibberd 49 , Reference Gibson and Roberfroid 129 ). Common prebiotics include the fructans, inulin and oligofructose, lactulose, galatooligosaccharides, arabinogalactan, arabinoxylan, pectic-oligosaccharides, β-glucan and resistant starches, all of which have been shown to increase the relative abundance of bifidobacteria within the human gut microbiota( Reference Tuohy, Brown and Klinder 22 ). Bifidobacteria are a group of bacteria particularly equated with gut health because of their saccharolytic nature, history of use as probiotics and because they dominate the gut microbiota of breastfed infants( Reference Gibson and Roberfroid 129 ). In parallel, many of these compounds have long been under investigation for their ability to modify blood lipid profiles mainly because of their ability to bind cholesterol or BA in the upper gut and increase sterol excretion, or for some, through their gel-forming nature causing a bulking effect in the intestine and triggering satiety( Reference Delzenne, Daubioul and Neyrinck 130 , Reference Slavin 131 ). Animal studies have consistently shown that dietary intervention with prebiotics, especially the fructans inulin and oligofructose, can reduce serum TAG( Reference Delzenne, Daubioul and Neyrinck 130 ). Elevated TAG, together with elevated total and LDL-cholesterol, low HDL-cholesterol and elevated total cholesterol:HDL-cholesterol characterise dyslipidaemia, and represent an important modifiable risk factor for CVD. Brigenti( Reference Brighenti 132 ) conducted a metanalysis of human studies investigating the ability of fructans to impact on serum TAG and concluded that 81% of fifteen trials with inulin or oligofructose reduced serum TAG. Although this effect was small (about 7·5% reduction in serum TAG) and only statistically significant in five of the interventions, the effect appeared to be independent of gender, type of prebiotic, background diet, overweight, dyslipidaemia or diabetes. However, the low number of studies considered in the metanalysis limited the power to adequately assess the impact of these confounders. More recently, Jackson and Lovegrove( Reference Jackson and Lovegrove 127 ) reviewed the current evidence on the ability of prebiotics (as well as probiotics and synbiotics) to alter blood lipids including cholesterol levels. They found that the majority of prebiotic studies were conducted with either inulin or oligofructose (a hydrolytic product of inulin) and that despite ample evidence for TAG lowering and often cholesterol-lowering effects of prebiotic dietary supplementation in animals, prebiotic trials in human subjects gave favourable if inconsistent modulation in lipid levels( Reference Jackson and Lovegrove 127 ). Table 2 summarises dietary intervention studies in human subjects with recognised prebiotics( Reference Jackson and Lovegrove 127 ). Although eight out of the twenty-one studies showed that prebiotic intervention did not change the blood lipids levels, the remainder showed statistically significant lowering of lipid biomarkers of CVD risk, usually TAG and/or total cholesterol. Although, prebiotics appeared to be more efficacious in the hyperlipidaemic, more studies are needed in the specific target groups, especially those fulfilling the criteria for the metabolic syndrome and in the obese. Fermentation of prebiotics, as with other fermentable fibres, leads to the production of SCFA. Demigné et al.( Reference Demigné, Morand and Levrat 153 ) showed that in vitro, propionate was an efficient inhibitor of cholesterol biosynthesis in rat hepatocytes when acetate was the main substrate available. This in vitro observation was confirmed later in vivo. However, acetate only becomes the main substrate for cholesterol biosynthesis in the liver when animals are fed high-fibre diets. This indicates that SCFA produced during high fibre diets are unlikely to increase circulating cholesterol levels despite increasing availability of the substrate for cholesterol( Reference Jackson and Lovegrove 127 ). This explains also why prebiotic interventions do not always lead to lower cholesterol in human studies, as hepatic cholesterol biosynthesis from acetate in human subjects, especially those on a low fibre, high-fat Western-style diet, is likely to contribute very little to circulating cholesterol levels( Reference Jackson and Lovegrove 127 ). Kok et al.( Reference Kok, Morgan and Williams 154 ) showed that oligofructose induces the gut hormones glucose-dependent insulinotropic polypeptide and glucagon-like peptide-1 of rats fed normal chow and that this contributed to improved glucose homoeostasis and lipid metabolism. Glucose-dependent insulinotropic polypeptide induces insulin and stimulates lipoprotein lipase in adipocytes, while glucagon-like peptide-1 stimulates insulin secretion, improves insulin sensitivity and contributes to satiety by reducing gastric emptying. More recently, Cani et al.( Reference Cani, Neyrinck and Fava 19 ) have shown that the prebiotic oligofructose can reverse metabolic endotoxemia by reducing gut permeability and LPS leakage into circulation. They also proposed the term ‘metabolic endotoxemia’ to describe the chronic low-grade systemic inflammation characteristic of obesity and type 2 diabetes( Reference Cani, Amar and Iglesias 16 ). Improved gut barrier activity resulted from prebiotic induction of the gut hormone glucagon-like peptide-2, a result confirmed in a later animal experiment( Reference Jones, Begley and Hill 78 ). More recently, Everard et al.( Reference Teixeira, Grześkowiak and Franceschini 155 ) confirmed Cani's earlier work that the prebiotic oligofructose could reduce gut permeability and associated metabolic endotoxemia and obesity in high-fat-fed animals through induction of intestinal endocannabinoids. They also found that prebiotic oligofructose-induced microbiota modulation also resulted in increased relative abundance of Akkermansia muciniphila, a mucus dwelling and degrading intestinal commensal. The authors found that the same physiological effects could be brought about by ingestion of Ak. muciniphila alone as a ‘probiotic’, but that the effect depended on microbial viability.
Table 2. Dietary interventions investigating the ability of common prebiotics (inulin, oligofructose/fructooligosaccharides and galactooligosaccharides) to improve blood lipid profiles in human subjects (after( Reference Jackson and Lovegrove 127 , Reference Brighenti 132 ))
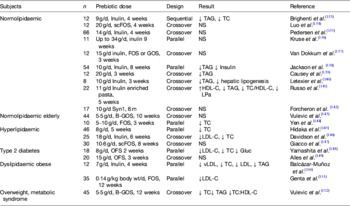
TC, total cholesterol; FOS, fructooligosaccharides; scFOS, short-chain FOS; OFS, oligofructose; GOS, galactooligosaccharides; B-GOS, Bi2muno GOS; Gluc, glucose; LPa, lipoprotein(a).
However, it must be emphasised that it is often difficult to extrapolate animal data to the human situation, where the genetic background is much more diverse, diets are open and vary greatly between individuals and where other life-style factors are sometimes not reported or underreported (e.g. alcohol intake, drugs and smoking, and high fat, high sugar convenience foods). Moreover, the quantities of prebiotic used in animal studies targeting metabolic disease and cholesterol are much higher than those routinely used in human dietary interventions. 10% w/w is the typical dose used in mouse studies and equates to more than 50 g/d prebiotic for human subjects, using mean European adult male body weight( Reference Reagan-Shaw, Nihal and Ahmad 156 ). Interestingly, this level of fermentable fibre intake in human subjects correlates well with estimates of dietary fibre intake for ancient diets and traditional high-fibre diets in the Mediterranean, Africa and China( Reference Tuohy, Gougoulias and Shen 31 ).
Reducing CVD risk with whole-grain cereals: oats as a case study
Whole-grain cereals, and in particular oats and fibre fractions derived from oats, have long been considered as foods capable of reducing CVD risk by lowering blood cholesterol levels. Whole-grain oats comprise a number of different classes of biologically active molecule capable of modulating cholesterol metabolism in mammals, including mono- and di-unsaturated fatty acids, fibres such as β-glucan, arabinoxylans and resistant starch, phenolic compounds such as dehydroferulates and avenathramides, and lignans which are converted into the phytoeostrogens enterolactone and enterodiol by gut bacteria( Reference Ryan, Kendall and Robards 157 , Reference Borneo and León 158 ). However, early studies attributed the cholesterol-lowering effect of oats to their gel-forming nature within the gut and/or cholesterol/BA binding. β-Glucan is the major structural polysaccharides accounting for about 85% of oat cell wall and is a soluble fibre, which, depending on molecular length, forms viscous gels in aqueous solutions. Tiwari and Cummins( Reference Tiwari and Cummins 159 ) performed a recent metanalysis on the ability of β-glucans to reduce blood cholesterol and glucose levels. Considering 126 clinical studies with different doses of β-glucans from either oats or barley, the authors concluded that there was a significant inverse relationship between β-glucan intake and blood total cholesterol, LDL-cholesterol and TAG levels. Moreover, there was a significant dose–response between β-glucan ingestion and cholesterol-lowering activity, with 3 g/d of either oat or barley β-glucan being sufficient to lower blood total cholesterol (by −0·30 mm). β-Glucan from either oats or barley are one of the few fibres to have attained health claims status with the European Food Safety Authority( 160 ). While the ability of oats and β-glucans to increase excretion of BA and cholesterol in faeces appears to be well established in the literature, the underlying mechanism still remains to be determined. β-Glucans have been proposed to alter the viscosity of the unstirred layer lining the intestinal mucosa thus reducing the flow of BA towards the epithelial cell surface and reducing BA/cholesterol absorption. The gel-forming nature of β-glucans has been suggested to trap BA/cholesterol micelles thereby reducing the efficiency of cholesterol absorption, and finally, β-glucans, especially those of small molecular weight, have been suggested to bind BA, directly inhibiting their reabsorption in the small intestine and driving them distally into the colon where they are deconjugated/hydrolised by the resident microbiota( Reference Gunness and Gidley 161 ). Whether one or all of these mechanisms holds is still a matter of debate. However, other possibilities also exist, not least the ability of oats or β-glucan to impact on the gut microbiota and their metabolic activities. Andersson et al.( Reference Andersson, Axling and Xu 162 ) investigated the ability of oat bran (27%) to lower blood cholesterol in two substrains of C57BL/6 mice on artherogenic diet (low choline diet), the C57BL/6 NCrl and the C57BL/6JBomTac strains, compared with control fibres. In the C57BL/6 NCrl mice, dietary intervention with oat bran reduced blood cholesterol with a concomitant increase in faecal BA excretion and increased hepatic BA synthesis. No change in cholesterol, BA excretion or synthesis was observed in the C57BL/6JBomTac. However, the C57BL/6JBomTac mice carried significantly lower intestinal populations of Enteorbacteriaceae, Akkermansia and Bacteroides fragilis than the C57BL/6 NCrl mice, and changes within the relative abundance of the gut microbiota appeared to predict cholesterol response to oat bran. This in vivo experiment suggests that the gut microbiota play a critical role in the cholesterol-lowering ability of oat bran. In vitro we have shown that different oat preparations can mediate a prebiotic modulation of the human gut microbiota, giving significant increases in bifidobacteria, lactobacilli and SCFA( Reference Hughes, Shewry and Gibson 163 – Reference Connolly, Tuohy and Lovegrove 167 ). We also showed that β-glucan increases the relative abundance of bifidobacteria in C57BL/6 mice fed high-fat diet, and regluates food intake by suppressing neuronal signals in appetite centres of the brain( Reference Arora, Loo and Anastasovska 20 ). The BA sequestrant, colestyramine has been shown to trigger satiety via reduced gastric emptying in healthy human subjects( Reference Psichas, Little and Lal 168 ), suggesting that factors controlling the enterohepatic circulation of BA may contribute to satiety as well as regulating blood cholesterol levels. Interestingly, recent studies showing that probiotic microorganisms can reduce cholesterol uptake from the intestine via modulation of BA signalling, discussed earlier( Reference Jones, Martoni and Parent 29 , Reference Jones, Martoni and Prakash 30 ) raise another possibility that oats and prebiotics in general (including certain polyphenols) may selectively stimulate gut bacteria capable of hydrolysing bile salts modifying the enterohepatic circulation of BA both in terms of quantity and chemical profile( Reference Nokoff and Rewers 39 , Reference Jones, Tomaro-Duchesneau and Martoni 79 , Reference Ooi, Ahmad and Yuen 169 – Reference Kim, Yi and Lee 171 ). Intestinal lactobacilli and bifidobacteria commonly encode bile salt hydrolysing enzymes and changes in their relative abundance wrought by probiotics, prebiotics and polyphenols could increase also their relative contribution to modifying the pool of BA returning from the gut and acting as signalling molecules in the liver, the gut wall and systemically through receptors FXRα and TGR-5 to impact on lipid absorption from the intestine, lipid and glucose homoeostasis, thermogenesis and inflammation( Reference Jones, Martoni and Prakash 30 , Reference Charach, Grosskopf and Rabinovich 80 , Reference Davidson, Maki and Synecki 146 , Reference Yamashita, Kawai and Itakura 148 , Reference Alles, de Roos and Bakx 149 ).
Conclusion
New observations on how diet shapes both the composition and metabolic output of the gut microbiota place developments in probiotics and prebiotics into an anthropological setting, establishing a scientific rationale for efficacious functional food design. Foods long known to be protective against CVD are emerging as efficacious microbiota modulators and suggest that diets high in fruit, vegetables and whole-grain cereals inherently rich in fermentable fibres, prebiotics and polyphenols may mediate at least some of their beneficial activities through the gut microbiota. Conversely, high-fat, high red meat and low-fibre diets, are associated with reduced microbiota diversity, increased relative abundance of undesirable microorganisms and increased production of toxic compounds including the cardiotoxicant TMAO. These observations raise the intriguing possibility that gut microbiome interactions with whole-plant foods, probiotics and prebiotics may be at the base of healthy eating pyramids advised by regulatory agencies across the globe, as summarised in Fig. 1. Moreover, recent studies showing that probiotic bacteria though modulation of the enterohepatic circulation of BA can raise plasma unconjugated BA concentrations and concomitantly reduce lipid absorption from the intestine and reduce blood cholesterol levels, suggests at least one unifying theory of probiotic, prebiotic and polyphenol mode of action, through BA sequestration and modified microbiota derived BA profiles and regulation of nuclear receptors such as FXRα and G-protein-coupled receptors such as TGR-5. These important physiological signalling molecules have been implicated in many aspects of CVD risk including BA, cholesterol and glucose homoeostasis, inflammation and arterial function. Taken together these observations suggest that at least one way to heart health may be through modulation of the gut microbiome.
Acknowledgements
The authors would like to thank the Nutrition Society and Royal Society of Medicine for allowing the corresponding author to present this paper at their Joint Winter Meeting on ‘Dietary Strategies for the Management of Cardiovascular Risk’, December 11–12, 2012, London, UK. In particular, we would like to sincerely thank the Organising and Scientific Committees especially Professor Julie Lovegrove, University of Reading.
Financial support
This paper was not supported by external finance.
Conflicts of interest
None.
Authorship
K. M. T. was the lead author and involved in all aspects of the review. F. F. and R. V. contributed to writing and editing the article, scientific content, presentation style and preparing the artwork.