During childhood and adolescence, bone mass rapidly increases, such that approximately 90 % of bone mass is acquired by age 20 years(Reference Henry, Fatayerji and Eastell1, Reference Recker, Davies and Hinders2). Thereafter, bone mass enters a period of relative stability before beginning an age-related decline as we enter later middle age. This response occurs in both men and women but, in general, men have greater bone mineral density (BMD) than women, while women also have a slightly higher rate of age-related BMD decline, particularly during the early postmenopausal period(Reference Hendrickx, Boudin and Van Hul3). A normal rate of bone loss does not tend to present a major clinical problem unless the individual did not generate a high enough peak bone mass during childhood and adolescence; under these circumstances, the development of osteopenia or osteoporosis can become clinically relevant issues. Even with a reasonable degree of bone accrual during childhood and adolescence, these conditions can still develop during older age with an accelerated rate of bone loss, which can occur as a result of an imbalance between osteoclast-mediated bone resorption and osteoblast-mediated bone formation; whereby the rate of bone resorption exceeds the rate of bone formation(Reference Demontiero, Vidal and Duque4).
Osteoporosis is a progressive systemic skeletal disease characterised by low bone mass and micro-architectural deterioration of bone tissue, with consequent increase in bone fragility and susceptibility of fracture(5) and is usually indicated by comparing BMD values to young healthy individuals of the same sex, thus generating a T-score. To standardise the diagnosis of osteoporosis, the WHO categorised a T-score of −1 or more as normal, with a score of between −1 and −2·5 being indicative of osteopenia and a score of −2·5 or below defining osteoporosis(5). A z-score can also be calculated, usually in older individuals to indicate a severity of osteoporosis, by comparing an individual's BMD to that of age-matched individuals with normal bone mass(Reference Blake and Fogelman6). Areal BMD, as generated using dual-energy X-ray absorptiometry, only accounts for about 60–70 % of the variance in bone strength(Reference Ammann and Rizzoli7), however, and there is a need to consider volumetric BMD, bone geometry and bone architecture in the context of bone strength, as highlighted by the WHO definition.
Twenty-two million women and 5·5 million men in the European Union(Reference Hernlund, Svedbom and Ivergård8) are affected by osteoporosis, which, in itself, is not necessarily a major clinical problem, but does increase the risk of developing an osteoporotic fracture; a major clinical problem affecting both the quality and quantity of one's life(Reference Cauley9). There were 3·5 million osteoporotic fractures in the European Union in 2010; 620 000 of which were hip fractures, 520 000 of which were vertebral fractures, 560 000 of which were forearm fractures and 1800 000 of which were classified as other fractures(Reference Hernlund, Svedbom and Ivergård8). The UK Office for National Statistics predicted, in 2016, that the prevalence of osteoporosis will increase in the coming decades as a direct result of population ageing, with over one third of the UK population aged over 50 years(Reference Park10). Additionally, failure to meet physical activity guidelines is common place in today's society, with negative implications for numerous chronic health conditions(Reference Blair, Sallis and Hutber11), including reduced BMD(Reference Chastin, Mandrichenko and Helbostadt12, Reference Langsetmo, Hitchcock and Kingwell13). Should current societal trends towards reduced physical activity, and increased sedentary behaviours continue, the prevalence of lifestyle-associated conditions, such as osteoporosis, might also increase.
There are a number of non-modifiable (e.g. genetics, age, sex and race) and modifiable (e.g. exercise, diet and smoking) factors that influence both bone accrual and loss. Among the modifiable risk factors, the mechanical loading achieved through some types of exercise undoubtedly has the largest positive effect on the bone, with high-impact, multi-directional-type activities generally considered to provide the greatest osteogenic stimulus(Reference Frost14, Reference Lima, De Falco and Baima15). In contrast, smoking is clearly deleterious(Reference Wong, Christie and Wark16). With regards to nutrition, the macronutrients (e.g. carbohydrate, fat and protein) and many micronutrients (e.g. calcium, vitamin D, vitamin K, magnesium, potassium, phosphorus, etc.) are known to modulate bone(Reference Ilich and Kerstetter17). Of these, perhaps one of the most interesting nutrients is protein, partly because it has been suggested to exert both positive and negative effects.
Protein makes up about half the bones volume and about 33 % of its mass(Reference Heaney18) and the structural matrix of bone consists of protein encased in a crystalline mineral(Reference Zimmerman, Busse and Ritchie19). Given this and the fact that collagen and non-collagenous proteins form the organic matrix of bone, it would seem logical to suppose that there might be an important role for dietary protein intake on bone accrual during childhood and adolescence and in the maintenance of bone health in older age. In contrast, however, early findings(Reference Sherman20) have suggested that there might be a negative impact of a high dietary protein intake on bone, largely due to a greater loss of calcium from the skeleton in order to offset an increase in acid load.
Theoretical evidence exists to support the fact that there might be both positive and negative effects of protein on bone, but there is limited consensus on whether protein is, in fact, a bone-protective or harming nutrient. The aim of this review is to summarise the potential mechanisms that may lead to either a positive or a negative influence of protein on bone. We will subsequently consider evidence on the influence of dietary or supplementary protein intake on indicators of bone health, thus evaluating the net effect of these, at times conflicting, pathways. Finally, we will consider situations whereby higher than recommended protein intakes may be advisable, as well as making recommendations for ongoing research and practice in this area.
Against: mechanisms through which protein may negatively impact bone
For many years, the role of protein in bone health has been questioned, with many postulating that high dietary protein intakes could be detrimental to bone, due to the acidic load that this may impose on the body(Reference Kraut and Coburn21, Reference Barzel and Massey22). This has been termed the acid-ash hypothesis and is summarised in Fig. 1. The body requires a close to neutral pH for optimal function, and deviations from this homeostatic set-point can have widespread metabolic and physiological consequences(Reference Robergs23, Reference Aoi and Marunaka24). Accordingly, the body has a wide range of mechanisms designed to regulate pH and to prevent large deviations towards either an acidic or alkaline environment(Reference Levitsky25, Reference Hamm, Nakhoul and Hering-Smith26). It has long been recognised that the metabolism of foods results in the production of an acidic or alkaline residue, and therefore usual dietary intake can theoretically influence the pH of the body. The potential renal acid load (PRAL) of an individual's habitual dietary intake can be calculated using validated algorithms(Reference Remer, Dimitriou and Manz27–Reference Remer and Manz29), and this calculation provides an indication of the net endogenous acid production within the body. PRAL is proportional to acid-producing elements, including protein and phosphorus, and inversely related to alkaline elements, including potassium, calcium and magnesium. It has long been suggested that protein, and mainly animal proteins that have a high content of sulphur-containing amino acids, have an acidic effect on the body, while fruit and vegetables generally have an alkaline influence. Thus, a diet high in animal proteins, and low in fruit and vegetables, has been proposed to induce a state of low-grade metabolic acidosis, with wide-ranging consequences for various metabolic processes(Reference Carnauba, Baptistella and Paschoal30). One of the main physiological processes thought to be impacted by low-grade metabolic acidosis is bone metabolism(Reference Krieger, Frick and Bushinsky31). The reason for this is that an excess intake of acid-producing foods requires a proportionate amount of alkaline substances in order to neutralise this effect. If these alkaline substances are not present in the diet, they must be attained from another source. Bone tissue has numerous physiological roles within the body, one of which is to act as a reservoir of minerals, most of which have alkaline properties. It has been proposed, therefore, that during a state of low-grade metabolic acidosis, as may occur with high dietary protein intakes, minerals such as magnesium, potassium and calcium will be excreted from the bone into the blood stream, thus allowing for neutralisation of excess acid and a return to neutral pH(Reference Carnauba, Baptistella and Paschoal30, Reference Krieger, Frick and Bushinsky31).
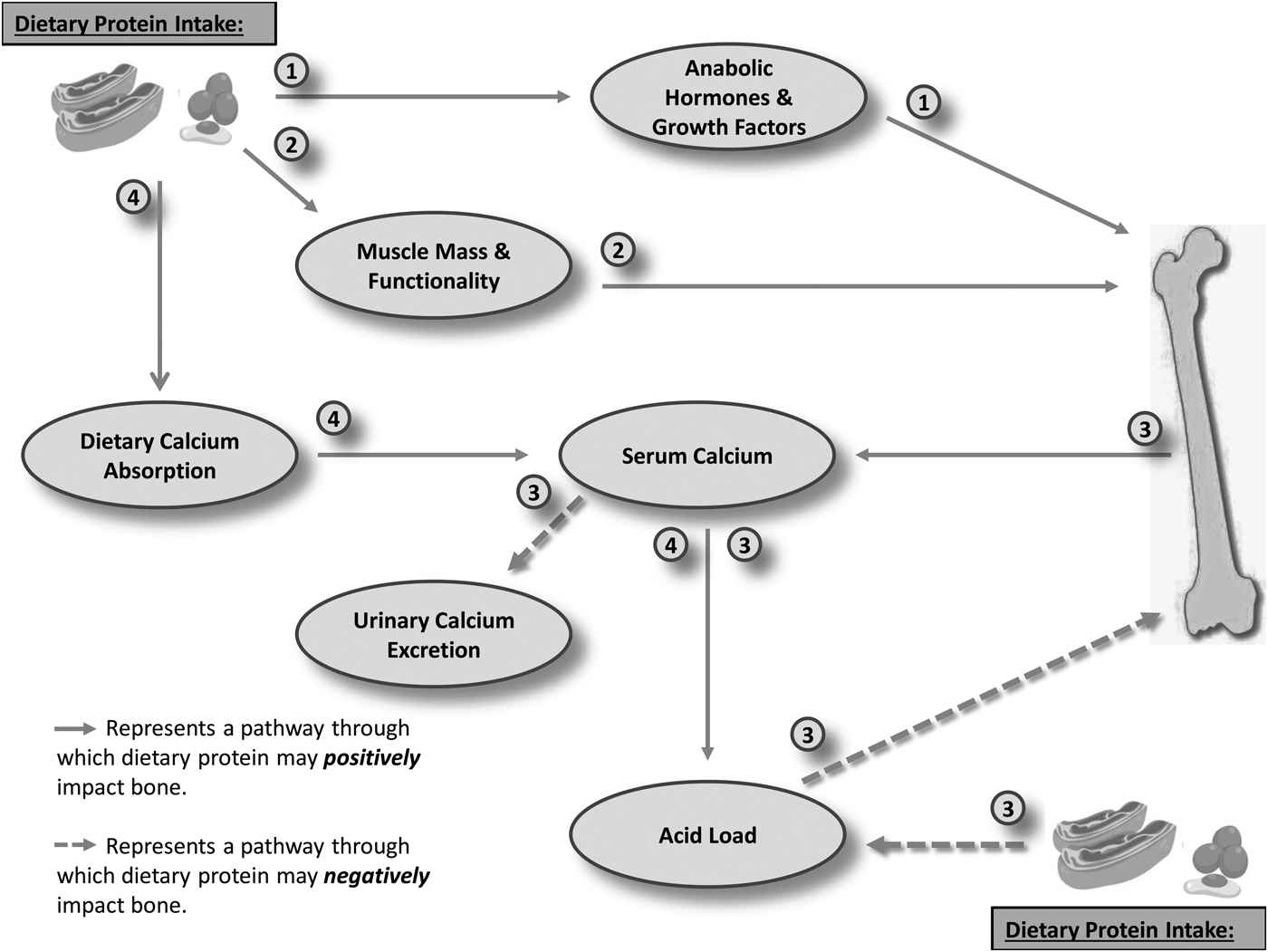
Fig. 1. Mechanisms through which protein may impact bone. Pathways: (1) Dietary protein up-regulates the activity of various anabolic hormones and growth factors (e.g. insulin-like growth factor 1; androgens; oestrogens or incretins), which in turn exert an osteogenic influence. (2) Dietary protein positively impacts muscle mass and functionality, with indirect benefit to bone through the increased mechanical loading that this provides. (3) Dietary protein increased the renal acid load, inducing a state of low-grade metabolic acidosis. Ca2+ and other alkaline minerals are leached from the bone in order to neutralise pH, thus reducing acid load. Ca2+ is subsequently lost through an increased urinary excretion, thus causing bone demineralisation. (4) Dietary protein increases dietary calcium absorption, thus increasing serum calcium availability, allowing for pH neutralisation, without undue detriment to bone.
A large body of evidence exists that theoretically supports the acid-ash hypothesis. A meta-analysis provided strong evidence that diets with high PRAL are indeed associated with higher urinary calcium excretion rates(Reference Fenton, Eliasziw and Lyon32). Indeed, if these losses continued unchecked over time, reported calcium losses of 66 mg/d would lead to a loss of 24 g, or approximately 2 % of total skeletal mineral mass per year(Reference Fenton, Eliasziw and Lyon32). Large cross-sectional studies have reported an inverse relationship between net endogenous acid production and BMD, and a positive association between net endogenous acid production and indicators of bone resorption(Reference New, MacDonald and Campbell33, Reference Macdonald, New and Fraser34), thus strengthening the belief that an acidic diet may be detrimental to bone. In further support of the acid-ash hypothesis, was a 4 d acute, cross-over trial, which reported that an alkaline diet inhibited bone resorption, while an acidic diet promoted urinary calcium and c-telopeptide of type 1 collagen excretion, demonstrating that an acidic diet may disrupt bone metabolism towards a resorptive state(Reference Buclin, Cosma and Appenzeller35). The findings of these human studies are supported by in vitro evidence, which indicated that osteoblasts cultured at a pH of 7·4 are capable of abundant mineralisation that progressively declined with reduced pH until mineralisation halted at a pH of approximately 6·9(Reference Brandao-Burch, Utting and Orriss36). Similarly, osteoclast activity is stimulated by an acidic environment, thus elevating bone resorption(Reference Yuan, Xu and Li37).
The acid-ash hypothesis has led to widespread belief that an increased calcium excretion, as a result of high-protein intakes, will lead to subsequent bone demineralisation. Accordingly, traditional dietary advice has suggested that high dietary protein intake should be avoided in order to protect the structural integrity of bone tissue. The acid-ash hypothesis is, however, based upon the assumption that the excess calcium excreted when individuals consume a high-protein diet derives from skeletal demineralisation. Kerstetter et al. investigated this by administering doubly labelled calcium isotopes in conjunction with a moderate- and high-protein diet for 10 d; showing that the hypercalciuria induced by the high-protein diet actually derived from dietary calcium intake, and not, as previously assumed, from the bone(Reference Kerstetter, O'Brien and Caseria38). Increased calcium excretion during periods of high-protein intake may, in fact, derive from other sources, including a modulation of calcium renal handling, or an increase in gastrointestinal calcium absorption(Reference Calvez, Poupin and Chesnau39). Mangano et al. demonstrated the importance of nutrient-to-nutrient interactions between protein and calcium intakes and kinetics by investigating the relationship between dietary acid load, supplemental calcium and BMD in 1218 men aged >60 years. They showed an inverse relationship between PRAL and proximal femur BMD in men consuming <800 mg calcium daily, but no association between dietary acid load and BMD in men consuming >800 mg calcium daily(Reference Mangano, Walsh and Kenny40). Similarly, Dawson-Hughes and Harris showed that higher total protein intake was associated with improved BMD in a group that were supplemented with calcium and vitamin D, but not in those who were not supplemented. Consideration of the proportion of protein intake obtained from animal or plant sources did not alter these results, demonstrating that it was the total amount, and not the source, of protein that was related to the identified BMD changes(Reference Dawson-Hughes and Harris41). Thus, it appears that, although the acid-ash hypothesis has mechanistic merit, the actual influence of dietary acid load, and more specifically animal protein intake, on bone may be moderated by factors such as calcium availability and kidney function.
For: mechanisms through which protein may positively impact bone
In contrast to the widely held belief that high-protein intake may be detrimental to bone, there is evidence of various mechanisms, both direct and indirect, through which protein may be protective of bone(Reference Heaney18, Reference Bonjour, Ammann and Chevalley42). Proteins are carbon-, hydrogen-, oxygen- and nitrogen-containing molecules, comprising polymers of amino acids, of which there are twenty. The complexity of protein structure allows fulfilment of multiple and wide-ranging physiological roles, including functions in structural (collagen), contractile (myosin and actin), immune (antibodies) and regulatory (enzymes and hormones) processes(Reference Insel, Turner and Ross43). Many of these processes are essential to the maintenance of bone structure and functionality, and thus adequate protein intake may be essential to the development and maintenance of a healthy bone. Bone comprises a protein matrix encased in a crystalline mineral, and bone has been estimated to comprise approximately 50 % protein and 50 % mineral(Reference Zimmerman, Busse and Ritchie19). Thus, bone strength is not solely dependent upon mineralisation, but will also depend upon the integrity of its protein components. As such, protein has an essential and direct structural function to fulfil in bone metabolism.
In addition to its structural role, adequate protein intake is essential to stimulate the activity of anabolic hormones and growth factors(Reference Bonjour, Schuren and Chevalley44, Reference Atherton and Smith45), most of which have essential roles in the regulation of bone mass and micro-architecture(Reference Manolagas, O'Brien and Almeida46–Reference Guntur and Rosen49). For example, dietary protein intake contributes to the regulation of insulin-like growth factor 1 (IGF-1)(Reference Thissen, Ketelslegers and Underwood50), although given the effect of protein intake on circulating insulin concentrations, the independent effects are somewhat tricky to determine. The IGF are a group of pleiotropic growth factors, whose effects are in many ways mediated through the action of growth hormone(Reference Woelfle, Chia and Rotwein51), but which also exert direct anabolic influences(Reference Kaplan and Cohen52). These factors are widely recognised as having a key role to play in the processes linking dietary intake and growth(Reference Frystyk, Delhanty and Skjerbek53), and exert multiple influences on bone(Reference Kawai and Rosen48, Reference Guntur and Rosen49). These influences include chondrocyte proliferation and differentiation, as well as the stimulation of osteoblast activity(Reference Bonjour, Ammann and Chevalley42). Additionally, IGF-1 is purported to exert an influence on bone resorption(Reference Hofbauer, Khosla and Dunstan54), by mediating the stromal cell expression of osteoprotegerin and its ligand(Reference Mrak, Lanzi and Losa55). Given its potential role in the regulation of both bone formation and resorption, it has been suggested that IGF-1 may aid in the mediation of the complex coupling processes of bone remodelling(Reference Rubin, Ackert-Bicknell and Zhu56, Reference Ueland57), thus directly modulating the influence of nutritional intake on bone metabolism. IGF-1 may also indirectly act to regulate bone through a role in the moderation of calcium absorption(Reference Fleet and Schoch58). This influence may occur, at least in part, due to an increased renal conversion of the inactive 25 hydroxyvitamin D3 to its active form, 1, 25 dihydroxy-vitamin D3(Reference Zoidis, Gosteli-Peter and Ghirlanda-Keller59). It has also been suggested, however, that other non-vitamin D-related pathways may contribute to the influence of IGF-1 on calcium absorption, although research is ongoing to more fully elucidate these findings(Reference Fleet and Schoch58). Dietary protein intake has been reported to be inversely related to sex hormone-binding globulin concentration(Reference Longcope, Feldman and McKinlay60). Sex hormone-binding globulin is a plasma glycoprotein whose primary biological action is to bind, and thereby inactivate, many of the androgens and oestrogens(Reference Kahn, Hryb and Nakhla61). Both androgens and oestrogens are recognised as exerting pleiotropic osteogenic effects(Reference Manolagas, O'Brien and Almeida46, Reference Almeida, Laurent and Dubois47), and thus their bio-availability, as determined by sex hormone-binding globulin concentration, will exert multiple influences on bone metabolism. Indeed, sex hormone-binding globulin content has previously been reported to predict bone mass in a number of populations(Reference Dolan, McGoldrick and Davenport62, Reference Slemenda, Longcope and Peacock63).
Lean body mass exerts an important moderating influence on bone; thus dietary protein intake may indirectly influence bone through its impact on lean muscle mass. It is widely recognised that protein intake is an essential component governing lean muscle mass and functionality(Reference Witard, Wardle and MacNaughton64), and in determining the response of muscle to exercise and training(Reference Atherton and Smith45, Reference Biolo, Tipton and Klein65). In turn, lean body mass is recognised as one of the strongest predictors of bone mass(Reference Ho-Pham, Nguyen and Nguyen66). Additionally, physical loading is recognised as the primary determinant of bone mass and architecture(Reference Frost14, Reference Lima, De Falco and Baima15), with both gravitational and muscular loading known to stimulate the bone remodelling cycle, and ultimately to enhance bone(Reference Kohrt, Barry and Schwartz67). The strong body of evidence supporting a positive influence of protein intake on muscle mass and function is therefore likely to indirectly and positively influence bone.
In fact, a myriad of mechanistic pathways exist, which may govern the influence of dietary protein intake on bone. These include the influence of protein on the calcium/vitamin D/parathyroid axis, moderation of various nutrient-regulated hormones, including the androgens, oestrogens and incretins, along with its influence on the absorption and action of other nutrients, e.g. calcium, that directly impact bone. Additionally, the individual protein components, namely isolated amino acids, also act to regulate bone metabolism through a wide range of mechanisms(Reference MacDonell, Hamrick and Isales68). An in-depth discussion of all of these factors is beyond the scope of this review, but the examples provided herein do, however, serve to highlight how dietary protein intake may act to mediate the actions of hormones and growth factors that regulate bone metabolism, and ultimately, its strength and functionality.
The influence of dietary or supplementary protein intake on bone
It is clear from the information described in previous sections, that protein intake has the capacity to influence bone through a wide range of mechanisms, and that this influence may theoretically be either positive or negative. But what is the net effect of these pleiotropic, and at times conflicting, mechanisms on bone? A significant body of literature, based on diverse designs and populations, has evaluated the net effect of dietary or supplemental protein intake on bone. In the interest of conciseness, and to focus on studies that have been deemed to be of high quality, and with low risk of bias, we will focus our discussion on the results of meta-analyses that have been conducted to synthesise and evaluate the influence of dietary or supplemental protein intake on bone. For further information on this topic area, readers are referred to the recent comprehensive summary by Rizzoli et al.(Reference Rizzoli, Biver and Bonjour69).
Meta-analyses directly investigating the acid-ash hypothesis
A number of meta-analyses have been conducted to specifically test the elements of the acid-ash hypothesis(Reference Fenton, Eliasziw and Lyon32, Reference Fenton, Lyon and Eliasziw70–Reference Fenton, Tough and Lyon72). Briefly, and as described in the ‘Against: mechanisms through which protein may negatively impact bone’ section, this hypothesis states that a prolonged and high intake of acid-forming foods, such as animal proteins, may cause a state of low-grade metabolic acidosis within the body. This may subsequently lead to bone demineralisation, as calcium and other minerals are excreted from the bone in order to neutralise excess dietary acid, and restore the neutral pH, which the body requires for optimal function. In support of this hypothesis, Fenton et al. conducted a meta-analysis to assess the relationship between net acid and calcium excretion. The authors identified a linear relationship between urinary acid and calcium excretion, consistent with the proponents of the acid-ash hypothesis(Reference Fenton, Eliasziw and Lyon32). They also raised an important point, however, in that the linear relationship identified between net acid and calcium excretion does not provide any evidence related to the source of excess calcium excretion, and therefore the results of that particular meta-analysis could not be taken to infer bone loss as a result of a high acid-producing diet(Reference Fenton, Eliasziw and Lyon32). Indeed, the same group subsequently conducted investigations regarding the influence of diet acid load on calcium balance(Reference Fenton, Lyon and Eliasziw70), and on the influence of supplemental dietary phosphate on indicators of calcium balance and bone metabolism(Reference Fenton, Lyon and Eliasziw71). Despite the linear relationship between diet acid load and calcium excretion reported in their first meta-analysis, Fenton et al. subsequently reported that diet acid load had no influence on net calcium balance, nor on bone resorption, as assessed by N-telopeptides(Reference Fenton, Lyon and Eliasziw70), demonstrating that, although an increased dietary acid load did cause increased calcium excretion, this did not influence overall net calcium balance. This likely occurred due to other influences of protein on bone, such as an increase in dietary calcium absorption(Reference Calvez, Poupin and Chesnau39). Additionally, a meta-analysis of all data that reported the effect of manipulated dietary phosphate on bone outcomes indicated that dietary phosphate consumption caused a reduction of urinary calcium excretion, even when the phosphate salt used had a high acid load(Reference Fenton, Lyon and Eliasziw71). This finding was in direct opposition to the acid-ash hypothesis, given that it considers phosphate to be one of the main acid-forming components of our diets, suggesting that this should have led to an increase in calcium excretion and bone demineralisation. Further disputing the acid-ash hypothesis, were meta-analytic data from Shams-White et al., who investigated the differential impact of soya v. animal-based proteins on calcium balance and bone outcomes, reporting no difference between these dietary protein sources(Reference Shams-White, Chung and Fu73), thus disproving the widely held belief that animal proteins convey a greater acidic load, and subsequently, a higher degree of bone demineralisation, than plant-based proteins. Finally, Fenton et al. published a comprehensive meta-analysis, in which they applied Hill's epidemiological criteria for causality model to conclusively evaluate the state of science regarding the influence of dietary acid load on bone outcomes(Reference Fenton, Tough and Lyon72). Hill's model considers causality in relation to five criteria, namely temporality, strength, biological gradient, plausibility, consistency and experiment. The authors considered fifty-five studies of varying designs, all of which were deemed to be of high quality and with low risk of bias. They concluded that there was no causal association between dietary acid load and osteoporotic disease and, as such, that an alkaline diet was not protective of bone health(Reference Fenton, Tough and Lyon72). Indeed, pH regulation is essential for usual metabolic function, and accordingly, the body has a wide range of mechanisms designed to maintain the internal environment of the body fluids, with the kidneys having an essential role in regulating the acid–base environment of the body(Reference Bonjour74). Smith(Reference Smith75) stated that ‘the composition of the body fluids is determined not by what the mouth takes in, but what the kidneys keep’, and the scientific evidence collectively indicates that the maintenance of acid–base balance can be achieved without undue detriment to bone, due to the wide range of regulatory mechanisms that have evolved in order to protect the neutral environment of our bodies.
Meta-analyses investigating the influence of protein on BMD and fracture risk
The meta-analyses described earlier indicate that dietary acid load is unlikely to lead to bone demineralisation, as postulated by the acid-ash hypothesis. These investigations do not, however, describe the potential of protein to influence BMD, or fracture risk, both of which are important indicators of bone strength and functionality. Although it has its limitations, BMD assessed by dual-energy X-ray absorptiometry scanning is commonly accepted as the principal diagnostic tool for bone disorders such as osteoporosis(Reference Kanis, Borgstrom and De Laet76). Meta-analyses investigating the influence of dietary protein intake collectively indicate a positive, albeit small, effect of higher dietary protein intakes on BMD at various sites(Reference Darling, Millward and Torgerson77–Reference Wallace and Frankenfeld79). Darling et al. reported a positive association between dietary protein intake and BMD at all sites, although the estimated effect was small, with dietary protein intake only accounting for 1–2 % of the total variation in bone density(Reference Darling, Millward and Torgerson77). In relation to studies investigating the influence of supplemental protein, an effect was identified at the lumbar spine site only(Reference Darling, Millward and Torgerson77). More recently, Shams-White et al. conducted a comprehensive meta-analysis of sixteen high-quality randomised controlled trials and twenty prospective cohort studies, and reported a positive effect of higher protein intake on BMD at the lumbar spine, but not at the other sites investigated (total hip, femoral neck and total body). In addition, they did not show any effect of higher protein intake on bone turnover marker concentrations(Reference Shams-White, Chung and Du78). In agreement with the findings of Darling et al.(Reference Darling, Millward and Torgerson77), the effect of protein on BMD was small, with a net percentage change of 0·52 % (95 % CI 0·06, 0·97 %(Reference Shams-White, Chung and Du78)). Collectively, these meta-analyses indicate a beneficial, albeit small, influence of higher protein intakes on BMD. Ultimately, however, the main outcome of interest when assessing the influence of dietary protein on bone health is the susceptibility of the individual to fracture. Fracture risk is a complex and multi-factorial phenomenon, and there is no one outcome measure that can conclusively indicate who will fracture and who will not. As such, randomised controlled trials investigating the influence of supplemental or increased dietary protein are not available, and meta-analyses in this area have focused their attention on prospective cohort studies that have investigated the relationship between dietary protein intake and the occurrence of fracture(Reference Darling, Millward and Torgerson77–Reference Wu, Sun and Lv80). These meta-analyses have reported mixed results, with two large meta-analyses reporting no influence of higher protein intakes on fracture risk(Reference Darling, Millward and Torgerson77, Reference Shams-White, Chung and Du78), while two others concluded that there was some evidence that higher protein intakes could reduce hip fracture risk(Reference Wallace and Frankenfeld79, Reference Wu, Sun and Lv80).
Collectively, the available meta-analyses, which represent the highest level of evidence currently available, indicate no adverse effect of higher protein intakes on bone. Conversely, the available evidence appears to indicate a small but beneficial influence of higher protein intakes on BMD, along with a potential reduction in hip fracture risk. It is important to identify that the meta-analyses described herein generally focused on variation in protein intake within recommended ranges. As such, they were not designed to identify whether higher protein intakes, above the recommended daily intakes, are protective or harmful to bone. This is important, as it is generally recognised that most nutrients tend to exert a biphasic response, whereby optimal intakes exert a stimulatory and beneficial response, while lower or higher intakes may be harmful or inhibitory. Wallace and Frankenfeld investigated this topic, by conducting a meta-analysis of those randomised controlled trials, and prospective cohort studies, that specifically investigated the influence of dietary protein intake above the current US RDA of 0·8 g/kg/d(Reference Wallace and Frankenfeld79). The authors critically synthesised the evidence from sixteen randomised controlled trials and thirteen prospective cohort studies, and concluded that protein intakes above the current RDA could be beneficial in reducing fracture risk and preventing bone loss. No adverse effect of protein intakes above the current RDA was identified. Further disputing the notion that very high-protein intakes may be harmful to bone was evidence from a recent original study that reported no influence of 6 months of dietary protein intakes far in excess of the current RDA (>2·2 g/kg/d) on total body or lumbar spine BMD in well-trained women(Reference Antonio, Ellerbroek and Evans81).
Situations in which bone potentially requires higher protein intakes: the influence of lifespan, reduced energy availability and weight loss
As described earlier, there is no evidence of an adverse effect of higher protein intakes on bone, while some evidence of a positive influence on fracture risk and BMD exists. Recommendations related to the optimal protein intake to support bone health is an ever-evolving topic, and a myriad of factors must be considered when assessing the protein requirements of any one individual. Notwithstanding this complexity, there is some evidence to support an osteogenic influence of protein intakes above the current RDA of 0·8 g/d in certain situations; namely childhood, adolescence and old age, and in situations characterised by reduced energy availability.
Lifespan
It is generally recognised that there are three distinct phases of bone development throughout the lifespan, namely: (1) bone accrual (birth to about 30 years); (2) relative bone stability (about 30–45 years) and (3) bone loss (about >45 years)(Reference Rosen, Rosen, Bouillon, Compston and Rosen82). Phases (1) and (3) are critical points in the overall maintenance of bone health, and optimisation of bone accrual, followed by minimisation of age-related bone losses, is essential to prevent subsequent development of bone disorders, such as osteoporosis(Reference Rizzoli, Bianchi and Garabedian83). Physical activity, and the subsequent muscular and gravitational loads that it conveys on bone(Reference Frost14), is recognised as an essential determinant of bone accrual and maintenance throughout the lifespan(Reference Santos, Elliott-Sale and Sale84). Additionally, it seems that higher protein intakes may support these processes. Chevalley et al. reported that higher than median protein intakes enhanced the positive impact of physical activity on bone accrual in prepubertal boys(Reference Chevalley, Bonjour and Ferrari85). Accordingly, children and adolescents have higher RDA for protein than adults, namely, 1–3 years: 1·2 g/kg/d; 7–14 years: 1 g/kg/d; 15–18 years: 0·9 g/kg/d, with all other groups, apart from infants and athletes, recommended to intake 0·8 g/kg/d(86). Dairy products are often promoted as an ideal whole food to promote bone accrual in early years(Reference Heaney87) due to their nutritional composition, which comprises a high proportion of high-quality protein, with the term high-quality referring to a protein source containing all essential amino acids. Additionally, dairy foods are abundant in micronutrients deemed essential to bone, including calcium, magnesium and phosphorus(Reference Gaucheron88). Indeed an adequate intake of dairy products, typically defined as 2–3 servings of dairy daily, along with weight-bearing activity, have been recommended as important strategies to optimise bone accrual in the earlier stages of the lifespan(Reference Rizzoli, Bianchi and Garabedian83).
Bone loss and a subsequent increase in fracture risk is a well-known complication of ageing. Indeed osteoporotic fractures are associated with a wide range of adverse social and economic consequences(Reference Cauley9). Many of the pharmacological interventions intended to prevent or reverse bone loss have numerous adverse effects, limiting their long-term use(Reference Drake, Clarke and Khosla89). Accordingly, lifestyle strategies to protect and maintain bone throughout the lifespan are desirable. Exercise and physical activity habits are considered important to this process. Protein intakes may be particularly relevant for older adults to negate the negative consequences of senescence, and higher than the currently recommended daily protein intakes have been suggested to be required to protect bone in older adults(Reference Surdykowski, Kenny and Insogna90), as well as to enhance muscle mass and function(Reference Traylor, Gorissen and Phillips91).
Reduced energy availability and weight loss
A key factor in the regulation of bone is the amount of available energy for this process. Strong evidence exists supporting a negative impact of both acute and chronic exposure to reduced energy availability on bone health(Reference Papageorgiou, Dolan and Elliott92). Markers of bone formation have been reported to be reduced in response to low-energy availability (defined as <126 kJ (<30 kcal)/kg lean body mass/d(Reference Ihle and Loucks93, Reference Papageorgiou, Elliott-Sale and Parsons94)), and this is thought to occur in an attempt to preserve energy for more immediately essential functions, such as respiration, thermoregulation and necessary movement(Reference Loucks, Kiens and Wright95). Although the negative bone consequences of this phenomenon have primarily been investigated in athletes who have very high levels of training-related energy expenditure(Reference Mountjoy, Burke and Ackerman96), or individuals suffering from chronic eating disorders(Reference Robinson, Aldridge and Clark97), it may also have relevance for those undergoing weight loss interventions. There is a long-held belief that obesity may be protective of bone health, which is based on the positive associations reported between absolute body mass and bone mass(Reference Michaelsson, Bergstrom and Mallmin98, Reference Gerdem, Ringsberg and Akesson99), along with evidence that some weight loss interventions may also lead to bone loss(Reference Hunter, Plaisance and Fisher100). This likely occurs as a result of reduced energy availability, along with a concurrent loss of lean muscle mass. Accordingly, strategies to protect both bone and lean mass during weight loss are essential. Recently, we reported that increased adipose mass in overweight or obese populations is negatively correlated with bone mass, but only when accompanied by a relative reduction in lean mass, highlighting the importance of optimizing the relative proportion between adipose and lean mass when considering interventions to protect bone during weight loss(Reference Dolan, Swinton and Sale101). Exercise-based interventions appear to be the most logical way to achieve this. Importantly, evidence supports the efficacy of higher protein intakes to protect the bone during exercise- and diet-induced weight loss(Reference Josse, Atkinson and Tarnopolsky102). Josse et al. investigated the influence of a higher intake of dairy foods, dietary calcium and protein during diet- and exercise-induced weight loss on a range of bone metabolic markers(Reference Josse, Atkinson and Tarnopolsky102). They reported that higher protein and calcium intakes were protective of bone health, while still allowing equivalent weight loss due to the hypoenergetic diet under investigation. This study did not allow isolation of the independent effects of protein and calcium, although it is widely recognised that these nutrients are likely to have interactive osteogenic effects. Additionally, higher protein intakes are recognised as being protective of muscle mass during periods of reduced energy availability(Reference Phillips and Van Loon103). As described earlier, muscle mass is an important mediator of bone remodelling, which occurs due to the mechanical loads that muscle conveys to bone.
Concluding remarks and perspectives
Even though evidence exists supporting pleiotropic mechanistic pathways through which protein intake may positively or negatively impact bone, the highest level of evidence available supports a net osteogenic influence of dietary protein intake on bone health. In the presence of adequate calcium intake along with normal kidney function, it appears that the PRAL induced by a diet high in protein, be it animal or plant, does not lead to bone demineralisation, as purported by the acid-ash hypothesis. In contrast, evidence exists to support a positive, albeit small, effect of protein intake on bone mass and fracture risk, which likely occurs due to the influence of protein on anabolic hormones and growth factors, which themselves directly mediate bone metabolism, in addition to the indirect influence of high-protein intake on lean muscle mass and function. Despite this, a number of important research questions remain, which must be answered before consensus regarding the optimal protein intake required to optimise bone health can be reached. Higher than recommended protein intakes appear to be supported in some situations, such as in athletes who have high, training-related energy expenditure, and a high requirement for musculoskeletal repair and adaptation, individuals who have reduced energy availability, with and without the need to reduce body mass, or those in the earlier or later stages of the lifespan. Although higher than the current recommended protein intake of 0·8 g/kg/d may be required in these situations, just how high these protein intakes should be is not clear. It is important that higher protein intakes do not occur at the expense of the adequacy of other nutrients, nor that they result in an inadvertent energy surplus, which may in itself negatively impact bone, particularly in sedentary individuals. It is widely recognised that physical loading is the main modifying variable that determines bone mass, strength and functionality. Surprisingly, very little is known about how protein intakes may moderate this effect, and this is an important area of future research. This may be particularly relevant in the earlier and latter stages of the lifespan. It is widely recognised that optimal bone accrual in the early years, and thus developing a homeostatic reserve to subsequently protect against age-related bone loss, is a key factor determining the subsequent development (or otherwise) of osteoporosis and associated fractures. The combined influence of activity programmes with protein intake in children and younger adults are therefore of importance. This assertion is supported by data from Chevalley et al., who reported that higher protein intakes were associated with enhanced benefits of physical activity on BMD in a group of prepubertal boys(Reference Chevalley, Bonjour and Ferrari85). Similarly, bone loss and fracture typically present themselves in the latter third of the lifespan, meaning that strategies to protect bone in older adults, including the adequacy of protein intake, are highly important in the older population.
Importantly, and as described in this review, protein intakes do not impact bone health in isolation, and their ultimate impact may depend upon interactions with a wide range of other nutrients and metabolic factors. Acknowledgement of the complexity of these processes is important. Well-designed and rigorously controlled studies are required to isolate the mechanistic pathways through which protein may act to influence bone remodelling. Additionally, it is widely recognised that individual variation exists in response to virtually all nutritional interventions. Consideration of the individual response to controlled interventions that investigate the influence of protein on bone(Reference Swinton, Stephens Hemingway and Saunders104) may allow for elucidation of factors that moderate this response, thus enhancing our understanding of the complex and potentially multifaceted influence of dietary or supplemental protein on bone. The results of these studies should be interpreted within the context in which they were investigated, however, and wider extrapolations avoided. Additionally, all proteins are not equal, nor should recommendation-based research focus solely on the quantity of protein required. We support a whole-food approach to nutrition and whole foods comprise a combination of macronutrients, micronutrients and phytochemicals, the combination of which may ultimately impact their net effect on bone. Therefore, research is needed to elucidate the influence of protein per se, as well as to investigate the potentially disparate influence of various whole-food protein sources. More recently, research attention has investigated the differential influence of the timing of protein intake, along with its distribution throughout the day. To date, little is known about how these factors may act to moderate bone response to protein intake, which represents another exciting area of ongoing research.
Knowledge related to the influence of protein intake on bone has exponentially increased in recent years, and it seems to be time to abandon the long-held belief that higher protein intakes lead to bone demineralisation, particularly in healthy individuals who have an adequate calcium intake. Ultimately, it seems clear that protein has the capacity to exert a protective influence on bone, and ongoing research, designed to more fully investigate mechanistic pathways through which this occurs, along with the clarification of optimal quantities, sources and timing, will allow for the optimisation of this protective influence, thus providing an effective, non-pharmacological and lifestyle-orientated strategy to protect bone health.
Acknowledgements
Vectors used in Fig. 1 were designed by Freepik (www.freepik.com).
Financial Support
E. D. is supported by a research grant from the Fundação de Amparo à Pesquisa do Estado de São Paulo (FAPESP: 2015/11328-2 and 2017/09635-0).
Conflict of Interest
E. D. has no conflict of interest to declare. C. S. has received a small honorarium from The Dairy Council for a seminar presentation in 2018 on dairy and bone health.
Authorship
The authors had joint responsibility for all aspects of preparation of this paper.