Bone mass changes over the life cycle(Reference Weaver, Gordon and Janz1). In childhood and adolescence, there is a rapid increase in bone size, with a relatively large need for dietary calcium and protein. Peak bone mass (PBM) is achieved after age 20 years and is maintained through the mid-years (i.e. thirties to forties)(Reference Weaver, Gordon and Janz1). In women, there is a rapid loss of bone during the menopause due to loss of oestrogen(Reference Karlamangla, Burnett-Bowie and Crandall2), which leads to lower bone strength post-menopause (osteoporosis)(Reference Ishii, Cauley and Greendale3) and increased fracture risk(Reference Jones and Boelaert4). In men, there is a slower decline in bone mass(Reference Weaver, Gordon and Janz1), leading to osteoporosis at a more advanced age(Reference Adler5). It is important to achieve a good PBM in early life in order to prepare for the loss of bone with ageing(Reference Bachrach6). Apart from rare cases of pathologically high bone mass, generally, the higher the PBM, the less the likelihood of the occurrence of osteoporosis and bone fracture in later life(Reference Ferretti, Cointry and Capozza7). Suboptimal lifestyle factors may reduce the initial PBM and predispose the individual to increased risk of osteoporosis(Reference Weaver, Gordon and Janz1) (Fig. 1).

Fig. 1. Bone mass across the lifespan with optimal and suboptimal lifestyle choices. Source: Reproduced unmodified from Weaver et al.(Reference Weaver, Gordon and Janz1). Creative Commons Attribution-Non Commercial 4⋅0 International License (http://creativecommons.org/licenses/by-nc/4.0/).
Hormonal factors (sex hormones and insulin-like growth factor 1 (IGF-1)); nutritional factors (dietary nutrients such as calcium, protein, phosphorus and potassium) and mechanical factors (physical activity, bone shape and bone material properties) affect PBM(Reference Heaney8) (Fig. 2). Genetic factors are also important but are unmodifiable(Reference Bay, Levy and Janz9,Reference Chanpaisaeng, Reyes Fernandez and Fleet10) . It is therefore important for public health interventions to focus on modifiable factors such as achieving adequate intakes of bone-supporting nutrients, ensuring adequate exercise and a healthy body weight.
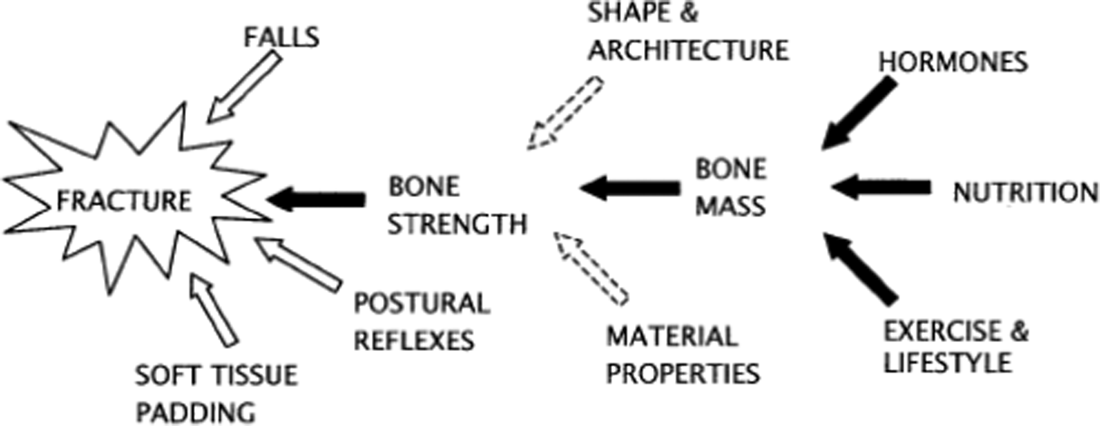
Fig. 2. Factors contributing to osteoporosis fracture risk. Source: Reprinted from Heaney(8). Copyright (2020), with permission from Elsevier. https://www.sciencedirect.com/science/article/pii/S8756328203002369?via%3Dihub
Dietary protein is crucial for the maintenance of bone tissue as well as for bone growth. Bone is 35% protein and requires a supply of amino acids to be used for protein turnover. The mechanostat is a process whereby bone remodels itself in response to elastic deformation acting on it, one large provider of this force is muscle mass(Reference Frost11). This explains observations that higher muscle mass is associated with increased bone mass(Reference Chalhoub, Boudreau and Greenspan12). Adequate protein ensures an adequate muscle mass, so is an important determinant of bone health. The current internationally recommended protein intake guideline for adults of all ages is 0⋅83 g/kg/d(13) although values of 0⋅8 g/d are used by many agencies with some recommending higher values for the elderly(14–18). Requirements for infants, children and adolescents vary by country, but are higher than that in adults due to the need for growth. For example, in the UK, the recommended nutrient intake is 12⋅5 g/d in those aged 0–3 months(17) which translates to over 2 g/kg/d. Western populations are generally dietary protein sufficient. For example, in the UK National Diet and Nutrition Survey (2014–2016, aged 19–64 years), the median protein intake was 74 g/d. Based on a UK average body weight of 72 kg for women and 85 kg for men(18), this suggests a median intake of over 1 g/kg/d.
There are some groups in western societies, such as frail older people, who are at risk of a low-protein intake. For example, in one study, 32% of frail older people did not meet the 0⋅8 g/kg/d requirement(Reference Mendonca, Kingston and Granic19). Conversely, in another study older care home residents had sufficient protein intake, with 95 % attaining 0⋅8–1 g/kg/d(Reference Engelheart, Brummer and Berteus Forslund20), and an analysis of the UK National Diet and Nutrition Survey of protein intakes of the elderly after trimming for under-reporting indicated median intakes of 1⋅24 g/kg/d with a negligible prevalence of deficiency(Reference Millward21). Low-protein intakes are important due to the association of low-protein intakes and frailty in older people(Reference Rahi, Colombet and Gonzalez-Colaco Harmand22).
Also, protein-energy malnutrition is still very common throughout the developing world. For example, 22⋅2 % of children aged 0–59 months globally have stunted growth and 7⋅5 % of children have wasting(23), although actual protein deficiency per se is rare with growth deficits more likely to reflect enteric infections from a poor environment(Reference Millward24).
The present paper will now discuss the proposed anabolic and catabolic actions of protein on bone health. It will exclude discussion of weight-loss studies as protein metabolism may differ in this situation.
Anabolic associations of dietary protein with bone health
Protein intake stimulates the release of the hormone IGF-1(Reference Rizzoli, Ammann and Chevalley25), which increases muscle mass(Reference Rizzoli, Ammann and Chevalley25) and bone growth(Reference Rizzoli, Bonjour and Chevalley26). Accordingly, lower protein intake leads to lower IGF-1(Reference Rizzoli, Ammann and Chevalley25,Reference Switkowski, Jacques and Must27) which in turn leads to a lower bone mass(Reference Rizzoli, Ammann and Chevalley25–Reference Yakar, Werner and Rosen28). This could result in a higher fracture risk, with studies finding a negative association between IGF-1 concentration and predicted fracture risk(Reference Rizzoli, Ammann and Chevalley25,Reference Boker, Volzke and Nauck29,Reference Ohlsson, Mellstrom and Carlzon30) . Correcting low-protein intake theoretically leads to a variety of musculoskeletal health benefits in older individuals (Fig. 3)(Reference Rizzoli, Ammann and Chevalley25).
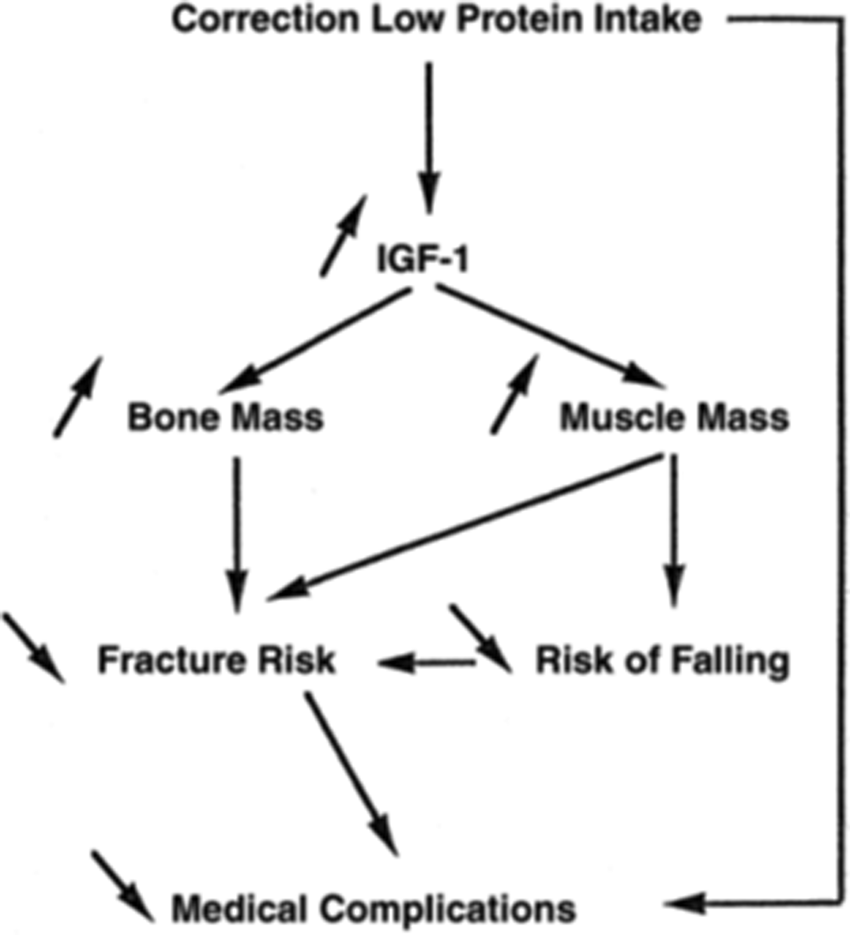
Fig. 3. Effects of correcting protein deficiency in older individuals. Source: Reprinted from Rizzoli et al.(Reference Rizzoli, Ammann and Chevalley25). Copyright (2020), with permission from Elsevier. https://www.sciencedirect.com/science/article/pii/S1297319X01002950?via%3Dihub. IGF-1, insulin-like growth factor 1.
Observational studies have shown a beneficial association between a higher protein intake and improved bone health. For example, in children and adolescents, cross-sectional analyses have associated a higher protein intake with a higher bone mineral content (BMC)(Reference Bounds, Skinner and Carruth31,Reference Ekbote, Khadilkar and Chiplonkar32) and a larger bone area(Reference Ekbote, Khadilkar and Chiplonkar32,Reference Hoppe, Molgaard and Michaelsen33) . In longitudinal research, studying children with high physical activity levels, a higher protein intake was associated with an increase in femoral neck bone mineral density (BMD) z score between age 7 and 15 years(Reference Chevalley, Bonjour and van Rietbergen34). However, lower protein intake was associated with a reduction in femoral neck BMD z score during the same time period(Reference Chevalley, Bonjour and van Rietbergen34). In older adults (over 60 years), higher protein intake has been associated, in cross-sectional studies, with higher spinal BMD(Reference Chiu, Lan and Yang35,Reference Rapuri, Gallagher and Haynatzka36) , total body BMD(Reference Rapuri, Gallagher and Haynatzka36) and femoral neck BMD in women(Reference Devine, Dick and Islam37). Higher protein intake has also been associated with higher total hip BMD in men and women(Reference Devine, Dick and Islam37,Reference Coin, Perissinotto and Enzi38) . Conversely, studies have found no difference in protein intake between women with normal BMD and women with osteopoenia or osteoporosis(Reference Gunn, Weber and Kruger39), and no association between protein intake and spinal or femoral neck BMD in older women(Reference Lau, Kwok and Woo40).
In premenopausal women, some studies have found that increased protein intake is associated with higher hip or spine BMD(Reference Quintas, Ortega and Lopez-Sobaler41–Reference Chan, Woo and Lau43) or BMC(Reference Quintas, Ortega and Lopez-Sobaler41,Reference Lacey, Anderson and Fujita44) . However, other studies have found no association with radial, spinal or femoral neck BMC(Reference Freudenheim, Johnson and Smith45) or lumbar spine or femoral neck BMD(Reference Henderson, Price and Cole42,Reference Chan, Woo and Lau43,Reference Freudenheim, Johnson and Smith45,Reference New, Bolton-Smith and Grubb46) . The few studies assessing younger to middle-aged men have found a positive association between protein intake and BMD in black men(Reference Jaime, Latorre Mdo and Florindo47) and vertebral BMC in all men(Reference Orwoll, Weigel and Oviatt48). However, studies have also found no association between protein intake and BMD in white men(Reference Jaime, Latorre Mdo and Florindo47) and no association for all men for total hip and spine BMD(Reference Whiting, Boyle and Thompson49) or radial BMC(Reference Orwoll, Weigel and Oviatt48). However, it must be borne in mind that not all observational analyses are multivariate adjusted. Some associations between dietary protein and bone health will be due to confounding from dietary, lifestyle and demographic factors. The type of protein consumed, and the adequacy of calcium intake may also vary between studies. These factors could explain differing results.
Protein supplementation studies have shown an improvement in BMD, BMC or other indices or bone size or strength in some studies but not others. For example, one study found improved bone growth after protein supplementation in malnourished children(Reference Lampl and Johnston50). However, there have been no trials to date in non-malnourished children. In terms of older people, in a study of hospitalised adults with a hip fracture, there was a reduced femoral shaft bone loss in those supplemented with 20 g/d protein(Reference Tkatch, Rapin and Rizzoli51). Similarly, a study of older patients’ post-hip fracture found that 20 g/d protein supplementation was associated with reduced proximal femur bone loss(Reference Schurch, Rizzoli and Slosman52). However, a study in community-dwelling adults aged 70–80 years, found no effect of whey protein supplementation (30 g/d) on bone mass or strength(Reference Zhu, Meng and Kerr53). Therefore, benefits of supplemental protein on bone may be confined to frailer older people post-hip fracture.
In terms of bone markers, over all age groups, evidence from trials is also mixed. Some studies have found no difference in bone markers in participants allocated to high- or low-protein diets(Reference Cao, Johnson and Hunt54) or participants allocated to a protein supplement compared to placebo(Reference Cuneo, Costa-Paiva and Pinto-Neto55). However, some studies have found lower bone resorption in those supplemented with protein(Reference Dawson-Hughes, Harris and Rasmussen56,Reference Hunt, Johnson and Fariba Roughead57) .
Catabolic associations of dietary protein with bone health
To maintain life, extracellular fluid must strictly stay within the limits of pH 7⋅35–7⋅45 (hydrogen ions between 0⋅035 and 0⋅045 mEq). Each day, human subjects on a typical western diet produce 1 mEq/kg body weight(Reference Lemann58). This increased physiological acidity leads to a series of physiological responses to neutralise the acid (Fig. 4)(Reference Lanham-New, Alghamdi and Jalal59). The body instigates buffering of body fluids, including increased bicarbonate production. The lungs increase carbon dioxide loss, the kidneys excrete more acid and bone loses alkaline mineral into the body fluids(Reference Lanham-New, Alghamdi and Jalal59). The latter is achieved via increased activity of osteoclast cells(Reference Arnett and Dempster60), which break down and remodel bone tissue. There is also evidence for a direct dissolution of bone calcium carbonate under exposure to acidity(Reference Bushinsky and Lechleider61). Studies of acidic states such as ammonium chloride ingestion(Reference Osther62) and starvation(Reference Grinspoon, Baum and Kim63) have demonstrated a negative calcium balance and increased calciuria(Reference Lanham-New, Alghamdi and Jalal59,Reference New64) . This negative calcium balance could have a negative impact on bone health if it occurs over the long term.
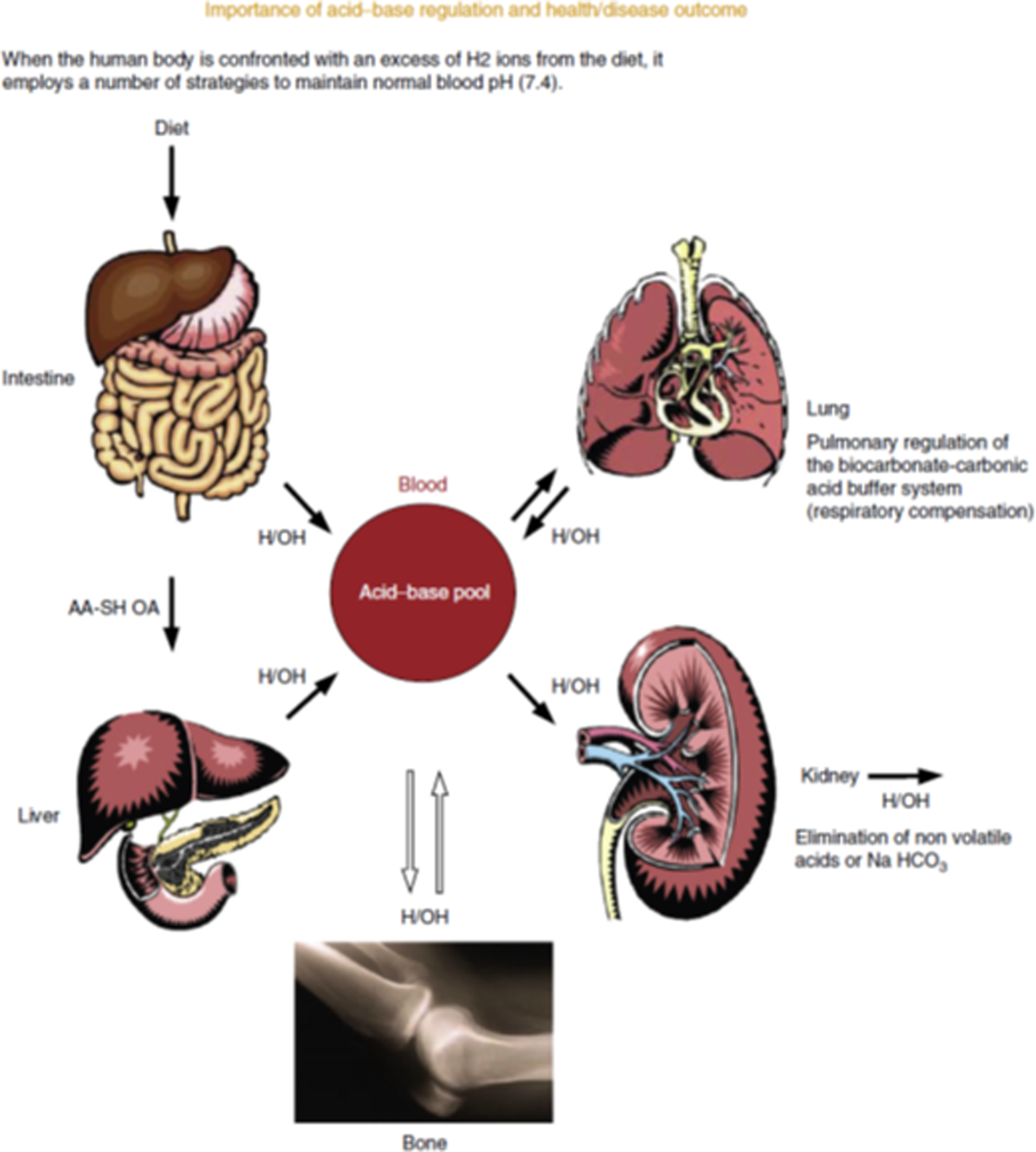
Fig. 4. (Colour online) Acid-base regulation. Source: Lanham-New et al.(Reference Lanham-New, Alghamdi and Jalal59). Reproduced with permission from Elsevier. https://www.sciencedirect.com/science/article/pii/B9780123750839000295. AA-SH OA, sulphur amino acids-organic acids; H2, hydrogen; OH, hydroxide; NaHCO3, sodium bicarbonate.
Dietary composition influences the acid–base status of the body. The consumption of sulphur amino acids from animal protein increases physiological acidity, as does phosphate from dietary phytates in grains. This means some cereal proteins produce as much, or more physiological acidity than animal proteins. For example, oatmeal, walnuts and whole wheat are higher producers of acidity than are chicken, beef and cheddar(Reference Massey65). Consumption of green vegetables and fruit leads to increased alkalinity. This is because they contain alkaline potassium salts of weak organic acids such as citrate, lactate and malate.
A higher protein:potassium ratio is undesirable, as demonstrated by the finding that it is associated with increased higher renal net acid excretion(Reference Frassetto, Todd and Morris66). A higher protein:potassium ratio is associated with higher potential renal acid load(Reference Frassetto, Todd and Morris66). Therefore, high protein, without adequate protective potassium, will increase physiological acidity. The net endogenous acid production in modern western diets could have negative implications for bone health, if the acidity is large enough and for long enough. An analysis of the net endogenous acid production of modern and preagricultural diets found that modern diets had an average of +48 mEq/d compared with −88 mEq/d for the preagricultural diets(Reference Sebastian, Frassetto and Sellmeyer67). Therefore, today we consume more acidic diets than was previously the case.
In terms of epidemiology, some ecological studies in the 1990s have suggested that higher protein intakes are associated with a detriment to bone health. For example, two studies found a positive association between animal protein intake per capita and hip fracture incidence(Reference Abelow, Holford and Insogna68,Reference Frassetto, Todd and Morris69) . However, ecological studies are prone to bias due to the methodology used. Moreover, few, if any, cross-sectional, cohort studies or randomised controlled trials have found an association between higher protein intake and poorer indices of bone health.
It is known that calcium excretion may rise with increased protein intake suggesting a detriment to bone mass. However, evidence shows that calcium absorption may increase, offsetting calcium loss. One study, using a within-subjects study design, gave research participants a low-protein diet (0⋅7 g/kg/d) and a high-protein diet (2⋅1 g/kg/d). They found increased urinary calcium during the high-protein diet, but calcium absorption also increased(Reference Kerstetter, O'Brien and Insogna70). However, another intervention trial showed no difference in calcium absorption, urinary calcium excretion or level of bone resorption markers when consuming the RDA of protein compared with consuming three times the RDA(Reference Cao, Pasiakos and Margolis71). This suggests no detrimental effect of higher protein intake on calcium metabolism and bone markers. However, this was only a short-term trial in only a small sample size, and it is unclear what the effect would be on bone metabolism in the long term.
Baseline calcium intake may also be important. For example, in the Framingham study, the increased fracture risk associated with higher animal protein intake was only present in the participants with lower calcium intake (<800 mg/d)(Reference Sahni, Cupples and McLean72). There was no association between higher animal protein intake and fracture risk when calcium intake was sufficient (≥800 mg/d)(Reference Sahni, Cupples and McLean72). This suggests adequate calcium intake may offset any detrimental effects of a high animal protein diet.
Systematic reviews and meta-analyses on protein intake and bone
There are conflicting findings from systematic reviews and meta-analyses on dietary protein and bone health. Meta-analyses of protein supplementation have found either no overall effect(Reference Darling, Manders and Sahni73) or a tiny beneficial effect(Reference Darling, Millward and Torgerson74,Reference Shams-White, Chung and Du75) on bone health, with no evidence of a detrimental effect in any of the systematic review and meta-analyses published to date. Meta-analyses of cross-sectional studies assessing the relationship between dietary protein and bone health generally show a positive association(Reference Darling, Manders and Sahni73,Reference Darling, Millward and Torgerson74) , although the association is often not present when analysing only multivariate-adjusted studies(Reference Darling, Manders and Sahni73).
Meta-analyses of cohort studies have found either a beneficial association with fracture risk(Reference Groenendijk, den Boeft and van Loon76,Reference Wallace and Frankenfeld77) or no association with fracture risk(Reference Darling, Manders and Sahni73,Reference Darling, Millward and Torgerson74) . Therefore, any small gains in BMD may not translate into fracture risk in the long term(Reference Darling, Manders and Sahni73). The association between protein intake and bone health in observational studies is stronger in case–control studies compared with cohort studies(Reference Darling, Manders and Sahni73). This could be due to case–control studies having significant inherent bias(Reference Kopec and Esdaile78). Overall, the message across these meta-analyses is that there is no evidence of a detrimental association between protein intake and bone health. As evidenced earlier, some meta-analyses suggest a benefit of protein to bone health, but others suggest no association.
Towards a synthesised view of dietary protein and bone health
There have been recent efforts to synthesise the anabolic and catabolic mechanisms of dietary protein on bone health. A key review(Reference Thorpe and Evans79) discusses how the positive aspects of dietary protein intake, including increased calcium absorption and IGF-1 induced bone formation, work in tandem with the negative effects. Particularly, they discuss how protein may benefit bone health if consumed as part of a diet containing enough dietary calcium, and alkalising fruit and vegetables(Reference Thorpe and Evans79).
This synthesised approach may explain some complex findings of research studies. For example, in one study, higher dietary protein was associated with larger bone size (periosteal circumference and cortical area), and higher BMC and polar strength strain index(Reference Alexy, Remer and Manz80). However, children in the same study with a high dietary potential renal acid load had a lower BMC and cortical area than those with a lower dietary potential renal acid load(Reference Alexy, Remer and Manz80).
A low protein:potassium ratio is likely to be ensured by consuming a balanced diet. Indeed, there is an argument for a whole diet approach for bone health(Reference Massey65), which includes a balanced intake of nutrients such as protein, potassium, calcium and phosphate. As discussed earlier, one way of increasing potassium intake is to consume more fruit and vegetables. Adequate calcium intake may also help compensate for any sulphur amino acid-induced bone loss(Reference Dawson-Hughes and Harris81). Adequate protein intake ensures enough amino acids for growth and repair of body tissues but should not be in excess. Other food constituents such as soya isoflavones and caffeine may also have potential effects on bone health(Reference Massey65). Soya isoflavones are known to have oestrogen-like effects on the body. Therefore, theoretically they may have beneficial effects on bone. Some studies have found a benefit of soya isoflavone supplementation on BMD(Reference Taku, Melby and Takebayashi82,Reference Wei, Liu and Chen83) , but most studies have found no benefit(Reference Liu, Ho and Su84–Reference Tai, Tsai and Tu86). Higher caffeine intake has been associated with poorer bone health(Reference Poole, Kennedy and Roderick87), which could be due to a small caffeine-induced reduction in calcium absorption(Reference Barger-Lux and Heaney88). However, this could also be due to consumption of caffeinated beverages being higher in individuals who have low calcium intakes(Reference Heaney89).
Conclusion
There is a long-standing debate as to whether high dietary protein intakes are beneficial or detrimental for bone health. We know that adequate dietary protein intake is essential to provide amino acids for building and maintaining bone tissue. It also has anabolic effects on bone by stimulating the release of IGF-1 and calcium absorption from the gut. However, some forms of dietary proteins may increase net physiological acidity because of their sulphur amino acid or phytate content. This could lead to increased bone loss in the long term in order to provide a source of alkaline mineral.
Research over the past 40 years has supported both anabolic and catabolic associations between protein intake and bone health. Data from cross-sectional studies support a positive association. However, cohort studies assessing fracture risk show both positive and negative associations, leading to null associations in meta-analyses. Intervention studies assessing BMD show no effect (or a tiny benefit) of protein intake for bone health in adults. There is a lack of research on this topic assessing children and adolescents, as well as adults with very low or very high intakes of dietary protein.
To make sense of the opposing effects of dietary protein on bone we are moving towards a synthesised view whereby dietary protein has both anabolic and catabolic effects on bone. The overall effect depends on the whole diet, as food components modify the net physiological pH. For example, calcium-containing foods, or the consumption of fruit and vegetables, may contribute to reduced physiological acidity from a higher protein diet.
Acknowledgements
A. L. D. is very grateful to the UK Nutrition Society for the opportunity to present at the Nutrition Society Live 2020 virtual conference.
Financial Support
None.
Conflict of Interest
None.
Authorship
The authors had joint responsibility for all aspects of preparation of the paper.