Insulin resistance and hypertension are core metabolic abnormalities in highly prevalent diseases such as type 2 diabetes, the metabolic syndrome and obstructive sleep apnoea(Reference Kashyap and Defronzo1). Drug development for these diseases has focused on reversal strategies, while preventive interventions are mainly related to lifestyle changes. One of the lifestyle modifications advised is to limit caffeine intake, based on several studies describing that caffeine acutely increases blood pressure(Reference Riksen, Rongen and Smits2) and lowers insulin sensitivity(Reference Moisey, Kacker and Bickerton3, Reference Keijzers, De Galan and Tack4). Recently, the benefits of caffeine withdrawal have been questioned, since a number of epidemiological studies showed no association between long-term coffee consumption, type 2 diabetes risk(Reference van Dam, Willett and Manson5, Reference van Dam and Feskens6) and high blood pressure(Reference Noordzij, Uiterwaal and Arends7–Reference Conde, Pinto and Bárbara10), which confirms that acute and chronic caffeine intakes have opposite effects(Reference Arnlov, Vessby and Riserus11, Reference Greenberg, Boozer and Geliebter12).
Besides the discussion on the protective v. deleterious effects of coffee in type 2 diabetes and hypertension, there is also controversy concerning the nature of the compound involved in it. Some authors claim that the beneficial effects of coffee are mediated by caffeine(Reference van Dam, Willett and Manson5, Reference Zheng, Sayama and Okubo13, Reference Lopez-Garcia, van Dam and Rajpathak14) while others support that coffee components, apart from caffeine, are responsible for the protection against type 2 diabetes and cardiovascular disease(Reference Noordzij, Uiterwaal and Arends7, Reference Greenberg, Axen and Schnoll15, Reference Wu, Willett and Hankinson16). Regarding the protective role of caffeine intake in the development of type 2 diabetes, the primary mechanism proposed is weight loss attributed to increased thermogenesis, lipolysis and fat oxidation induced by the drug(Reference van Dam, Willett and Manson5, Reference Zheng, Sayama and Okubo13, Reference Lopez-Garcia, van Dam and Rajpathak14, Reference Cheung, Lee and Ng17); still this hypothesis remains debatable since it has also been observed that long-term coffee consumption does not cause significant weight reduction(Reference Astrup, Breum and Toubro18).
Sporadic caffeine intake has also been correlated with elevated blood pressure(Reference Riksen, Rongen and Smits2, Reference Sung, Whitsett and Lovallo19–Reference Pincomb, Lovallo and McKey21) due to sympathetic over-activation by increased catecholamine levels and down-regulation of β-receptors(Reference Benowitz22), antagonism of adenosine receptors(Reference Fredholm, Battig and Holmen23), increased noradrenaline release via direct effects on the adrenal medulla and activation of the renin–angiotensin system(Reference Geleijnse9). However, though caffeine administration raises blood pressure acutely, tolerance to this effect develops rapidly, and heavy coffee drinkers are less likely to show an increase in blood pressure after caffeine intake(Reference Robertson, Wade and Workman24, Reference Myers25). In fact, recent prospective studies even suggest a protective role of high coffee intake against hypertension(Reference Geleijnse9).
In the present study, we tested the effects of long-term caffeine intake, at a dose equivalent to moderate human consumption that was previously shown to produce plasma caffeine levels comparable with those in moderate consumers of caffeinated beverages(Reference Holtzman and Finn26, Reference Gasior, Shoaib and Yasar27) in the prevention of diet-induced insulin sensitivity and hypertension in two pathological animal models, the high-sucrose diet rat and the high-fat diet rat. We found that chronic caffeine consumption prevented the development of insulin resistance and hypertension and led to a decrease in circulating catecholamines in the pathological animal models tested. The present results also showed that the pharmacological inhibition of the sympathetic nervous system with carvedilol (CVD), an antagonist of α1, β1 and β2 adrenoceptors, prevented diet-induced insulin resistance in high-sucrose diet and high-fat diet rats similarly to caffeine. We propose that long-term caffeine administration has a protective effect on the development of diet-induced insulin resistance and hypertension through a decrease in circulating catecholamines.
Methods
Animals and experimental procedures
Experiments were performed in male and female Wistar rats (weight 200–420 g), aged 3 months, obtained from the vivarium of the Faculdade de Ciências Médicas. The animals were kept under temperature and humidity control (21 ± 1°C; 55 ± 10 % humidity) with a 12 h light–12 h dark cycle.
Rats were divided into six groups: (1) control group; (2) caffeine group (Caff); (3) high-fat diet group (HF), a model of the metabolic syndrome(Reference Shearer, Severson and Su28); (4) high-fat diet combined with caffeine intake group (HFCaff); (5) high-sucrose diet group (HSu), a lean model of combined insulin resistance and hypertension(Reference Ribeiro, Lautt and Legare29); (6) the high-sucrose diet combined with caffeine intake group (HSuCaff).
The control group was fed standard chow (7·4 % fat +75 % carbohydrate (4 % sugar) +17 % protein; SDS diets RM1; Probiológica, Sintra, Portugal); the HF group was fed a lipid-rich diet (45 % fat +35 % carbohydrate +20 % protein; Mucedola, Settimo Milanese, Italy) for 21 d; the HSu group was fed standard chow and drank 35 % sucrose (Panlab, Lisbon, Portugal) for 28 d. We chose to submit the HSu rats to a 28 d high-sucrose treatment, since at 21 d the animals were neither insulin resistant nor hypertensive (data not shown).
Caffeine (1 g/l) was administered in drinking water during the last 15 d of the experimental protocol(Reference Gasior, Jaszyna and Munzar30). In the HSuCaff group, sucrose and caffeine were administered in the same bottle.
A second set of experiments was performed to test if the effect of sympathetic blockade on insulin sensitivity was similar to the effect of caffeine in the pathological animal models tested. CVD, an antagonist of α1, β1 and β2 adrenoceptors, was the drug of choice and it was administered at a dose of 0·2 g/l in a 0·5 % methylcellulose solution in the drinking bottles, during the last 15 d of the experimental protocol. Methylcellulose was used to facilitate dissolution of CVD and absorption of the drug(Reference Rodriguez-Perez, Losada and Anabitarte31).
Rats were also divided into six groups: (1) the control group administered the vehicle, 0·5 % methylcellulose in drinking water (MC); (2) the CVD group; (3) the HF group administered the vehicle (HFMC); (4) the HF group treated with CVD (HFCVD); (5) the HSu group administered the vehicle (HSuMC); (6) the HSu group treated with CVD (HSuCVD). Individual daily liquid intake was measured to monitor caffeine and CVD ingestion. Body weight was assessed twice per week. On the last day of the experimental protocol, rats were fasted overnight and allowed free access to caffeine solutions or water. Afterwards, the animals were anaesthetised with intraperitoneal sodium pentobarbital (60 mg/kg) and transferred to a heating pad to maintain body temperature at 37·5 ± 0·5°C throughout the experiment. Animals were euthanised by an intracardiac overdose of pentobarbital.
Principles of laboratory care were followed in accordance with the European Union Directive for Protection of Vertebrates Used for Experimental and Other Scientific Ends (86/609/CEE). Experimental protocols were previously approved by the Ethics Committee for Animal Care and Use at the Faculty of Medical Sciences, New University of Lisbon (FCM-UNL; Faculdade de Ciências Médicas, Universidade Nova de Lisboa).
Measurement of insulin sensitivity
The insulin tolerance test (ITT) was used to measure insulin sensitivity. The ITT is one of the earliest methods developed to assess insulin sensitivity in vivo and provides an estimate of overall insulin sensitivity, correlating well with the ‘gold standard’ hyperinsulinaemic–euglycaemic clamp(Reference Monzillo and Hamdy32). The ITT consists of the administration of an intravenous insulin bolus of 0·1 U (4·5 μg)/kg body weight, after an overnight fast, followed by the measure of the decline in plasma glucose concentration over 15 min at 1 min intervals. The constant rate for glucose disappearance (KITT) was calculated using the formula 0·693/t1/2. Glucose half-time (t1/2) was calculated from the slope of the least-square analysis of plasma glucose concentrations during the linear decay phase. Blood samples were collected by tail tipping and glucose levels were measured with a Glucose Analyzer (1500 YSI Sport; Yellow Springs Instruments, Yellow Spring, OH, USA).
Measurement of blood pressure
To measure mean arterial pressure (MAP), the femoral artery was cannulated under a dissection microscope and the catheter was connected to a pressure transducer and amplifier (model 603; HSE-HA GmgH, Harvard Apparatus, Madrid, Spain). MAP data were acquired with HSE-Harvard PULMODYN W software (Harvard Apparatus).
Plasma caffeine quantification
In the caffeine-treated groups, plasma samples, collected by heart puncture into EDTA precoated tubes, were pre-treated for protein precipitation with perchloric acid (30 %) and neutralised with KOH (4 m)–2-amino-2-hydroxymethyl-propane-1,3-diol (Tris; 0·4 m). Caffeine and its metabolites theobromine, theophyline and paraxanthine were quantified by reverse-phase HPLC with low-pressure gradient with UV detection at 274 nm. The HPLC system consisted of an LC 10-A solvent delivery pump (Shimadzu, Kyoto, Japan), an automatic injector SIL-20AC (Shimadzu), a SPD-6 AV UV-VIS wavelength detector (Shimadzu) and Shimadzu Class VP software to analyse the chromatograms. A low-pressure gradient was used: the initial mobile phase consisted of a solution of 50 mm-KH2PO4 (pH 4·58), with 9 % of methanol and 2 % of acetonitrile running at a flux of 1·5 ml/min. Concentrations of the organic solvents changed throughout the sample run. Standards of caffeine, theophylline, theobromine and paraxanthine were prepared in 5 % of bovine serum albumin and submitted to the same pre-treatment as the biological samples. Identification of the peaks in the biological samples was made by comparison with the retention times of the standards.
Quantification of blood glucose, insulin, NEFA, nitric oxide and catecholamines
In all the experiments, plasma and serum were collected by heart puncture. Insulin, caffeine and NO were determined in plasma; NEFA and catecholamines were determined in serum. Insulin was quantified with an ELISA kit (Mercodia Ultrasensitive Rat Insulin ELISA kit; Mercodia AB, Uppsala, Sweden) and NEFA with a colorimetric assay (Zen-Bio Inc., Research Triangle Park, NC, USA). For NO/NO3− determination, plasma samples obtained were deproteinised by adding two volumes of ethanol (0°C). NO/NO3− concentration was determined by using a selective and sensitive NO/ozone chemiluminescence technique (NO-Analyzer 280; Sievers Research Inc., Boulder, CO, USA). For the quantification of catecholamines in serum, 500 μl of serum samples were purified and catecholamines were extracted using 30 mg OASIS Hlb Wat cartridges (Waters, Waltham, MA, USA) and eluted in 500 μl of mobile phase. A quantity of 100 μl of the samples was directly injected into an HPLC system composed of a Waters 600 controller pump, a Waters C18 (particle size 4 μm) column, a Waters 717 plus autosampler and a Bioanalytical Systems LC-4A electrochemical detector (set at a holding potential of 0·65 mV and a sensitivity of 1 nA). An isocratic elution was used: the mobile phase consisted of a solution of 25 mm-Na2HPO4 with 6 % of methanol (pH 3·55), running at a flux of 1·0 ml/min. The signal coming out of the detector was fed to an analogue to digital converter controlled by Peak Sample Chromatography System Software (Buck Scientific, East Norwalk, CT, USA). Identification and quantification of catecholamines were done against external standards.
Measurement of total hepatic glutathione
Glutathione is the major intracellular non-protein thiol and an important protector against free radical damage. We tested the effects of caffeine on hepatic reduced glutathione stores since it is known that total glutathione is significantly depleted after exposure to oxidative stress and increases in the presence of antioxidant compounds. A sample of the medium lobe of the liver was collected after an abdominal laparotomy and stored at − 80°C in an ultra-low freezer (Heraeus, Madrid, Spain). Approximately 100 mg of the tissue was weighed and homogenised in metaphosphoric acid (5 %) using a Teflon pestle. The homogenate was centrifuged at 12 000 g for 10 min at 4°C and the supernatant fraction was collected and frozen at − 80°C for future use. Total hepatic glutathione was quantified using an enzymic method (HT Glutathione Assay Kit, no. 7511-100-K; Trevigen European, Amsbio, Abingdon, Oxon, UK).
Collection and weight of abdominal fat
Renal, genital and visceral fat was collected after an abdominal laparotomy and weighed.
Drugs and chemicals
Caffeine, paraxanthine, theobromine, theophyline, bovine serum albumin, KH2PO4, KOH, metaphosphoric acid, Na2HPO4, noradrenaline, adrenaline, perchloric acid and Tris were obtained from Sigma-Aldrich (Madrid, Spain). Sucrose was obtained from Panlab. Insulin was commercially available as Humulin® Regular (Lilly, Algés, Portugal) in a concentration of 100 UI/ml (4·5 mg/ml). CVD was kindly provided by Labesfal (Lisbon, Portugal).
Data analysis
Data were evaluated using Graph Pad Prism Software, version 4 (GraphPad Software Inc., San Diego, CA, USA) and was presented as mean values with their standard errors. The significance of the differences between the mean values was calculated by one- and two-way ANOVA with Dunnett's and Bonferroni multiple comparison tests, respectively. Potential relationships between the variables under analysis were explored using Pearson's correlation coefficient. Differences were considered significant at P ≤ 0·05.
Results
The ingestion of caffeine in drinking water (1 g/l) was stable throughout the experiments in all the groups since there were no significant changes in individual daily liquid intake per kg. Liquid intake in the Caff group was 6·78 (sem 0·50) ml/kg per d, which was not significantly different from control (7·23 (sem 0·27) ml/kg per d). Liquid intake in the HF and HSu groups was 5·83 (sem 0·25) and 8·31 (sem 0·40) ml/kg per d, respectively. Liquid intake in the HFCaff and HSuCaff groups was 6·01 (sem 0·19) and 8·08 (sem 0·68) ml/kg per d, respectively. No significant differences were observed within the groups. Food intake was also not significantly different (control, 57·78 (sem 2·05); Caff, 55·03 (sem 5·25); HF, 62·56 (sem 1·99); HFCaff, 60·58 (sem 2·79); HSu, 51·22 (sem 4·51); HSuCaff, 52·78 (sem 3·81) mg/kg per d).
As expected we did not detect the presence of caffeine or its metabolites in control, HF and HSu groups. Plasma caffeine concentration in the Caff group was 3·73 (sem 1·08) μg/ml, in the HFCaff group 3·23 (sem 1·09) μg/ml and 4·34 (sem 1·15) μg/ml in the HSuCaff group. These values were not significantly different and are within the range of 0·37–5·95 μg/ml previously described by Gasior et al. for doses of 0·25–1 mg/ml of caffeine in drinking water in rats(Reference Gasior, Jaszyna and Munzar30). Although we did not monitor the physical activity of the animals we did not observe any behavioural changes during the period of caffeine ingestion.
Fasting glycaemia was not significantly different in control and HF groups although the HSu diet significantly increased fasting glycaemia in comparison with the control group (P < 0·001) (Table 1). Chronic caffeine intake did not modify fasting glycaemia both in the control and HF rats but it reversed hyperglycaemia induced by the HSu diet (P < 0·05) (Table 1).
Table 1 Effect of chronic caffeine intake (1 g/l) on glycaemia and insulinaemia in control rats and in rats fed high-fat and high-sucrose diets
(Mean values with their standard errors for all the individual samples obtained from 9–15 rats)
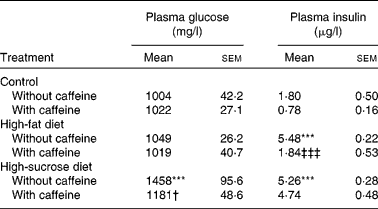
*** Mean value was significantly different from that of the control rats (P < 0·001; two-way ANOVA with Bonferroni multiple comparison test).
† Mean value was significantly different from that of the rats fed the high-sucrose diet without caffeine (P < 0·05; two-way ANOVA with Bonferroni multiple comparison test).
‡‡‡ Mean value was significantly different from that of the rats fed the high-fat diet without caffeine (P < 0·001; two-way ANOVA with Bonferroni multiple comparison test).
Fasting insulinaemia was 1·80 (sem 0·50) μg/l in control rats. Chronic caffeine intake did not significantly change basal insulin concentrations in control rats. Both the HF and HSu diets caused a significant increase in plasma insulin levels (P < 0·001; Table 1) in comparison with the control group. This effect was reduced to control values by chronic caffeine intake in HF (P < 0·001) but not in HSu rats (Table 1).
Effect of chronic caffeine intake on insulin sensitivity
Fig. 1 represents the effect of chronic caffeine intake (1 g/l) on insulin sensitivity in control, HF and HSu groups. The HF diet induced a decrease in KITT from a control value of 4·49 (sem 0·43) % glucose/min to 3·02 (sem 0·34) % glucose/min (P < 0·05). The HSu diet decreased KITT to 2·68 (sem 0·32) % glucose/min (P < 0·01). Chronic caffeine intake did not modify insulin sensitivity in control animals (KITT Caff = 4·52 (sem 0·23) % glucose/min). In both the HF and HSu diet groups, chronic caffeine intake completely reversed the insulin resistance induced by the diets (KITT HFCaff = 4·26 (sem 0·16) % glucose/min; KITT HSuCaff = 4·07 (sem 0·26) % glucose/min).

Fig. 1 Effect of chronic caffeine intake (1 g/l) on insulin sensitivity in control, high-fat (HF) and high-sucrose (HSu) diet rats, determined by the insulin tolerance test, expressed as constant rate for glucose disappearance (KITT). (□), Without caffeine (n 9–12); (■), with caffeine (n 12–15). Values are means, with standard errors represented by vertical bars. Mean value was significantly different from that of the control rats not given caffeine: *P < 0·05, **P < 0·01 (two-way ANOVA with Bonferroni multiple comparison test). Mean value was significantly different from that of the rats within the same diet group not given caffeine: † P < 0·05, †† P < 0·01 (two-way ANOVA with Bonferroni multiple comparison test).
Effect of chronic caffeine intake on mean arterial pressure
Fig. 2 displays the effect of chronic caffeine consumption on MAP in control, HF and HSu animals. HF and HSu administration induced a significant increase in MAP compared with controls (MAP control = 85·99 (sem 2·15) mmHg; MAP HF = 108·04 (sem 5·30) mmHg; MAP HSu = 104·69 (sem 2·72) mmHg). Chronic caffeine intake did not modify MAP in control animals; however, when administrated to HF and HSu rats, it completely prevented the hypertension induced by both diets (MAP Caff = 85·53 (sem 5·32) mmHg; MAP HFCaff = 86·92 (sem 3·96) mmHg; MAP HSuCaff = 92·13 (sem 1·82) mmHg).

Fig. 2 Effect of chronic caffeine intake (1 g/l) on mean arterial pressure (MAP) in control, high-fat (HF) and high-sucrose (HSu) diet rats. (□), Without caffeine (n 9–12); (■), with caffeine (n 9–15). Values are means, with standard errors represented by vertical bars. ** Mean value was significantly different from that of the control rats not given caffeine (P < 0·01; two-way ANOVA with Bonferroni multiple comparison test). Mean value was significantly different from that of the rats within the same diet group not given caffeine: †P < 0·05, ††P < 0·01 (two-way ANOVA with Bonferroni multiple comparison test).
Effect of chronic caffeine intake on body weight, visceral fat and NEFA
Fig. 3 represents the effects of chronic caffeine intake on weight gain (Fig. 3(a)), visceral fat (Fig. 3(b)) and serum NEFA (Fig. 3(c)). The HF diet caused a significant increase in weight gain per d compared with control animals, whereas the HSu diet did not (control = 1·53 (sem 0·26) g/d; HF = 4·32 (sem 0·45) g/d; HSu: 2·66 (sem 0·25) g/d). Caffeine intake did not significantly modify weight gain either in control or in HSu animals (Caff = 1·14 (sem 0·16) g/d; HSuCaff = 2·51 (sem 0·29) g/d). In contrast, caffeine administration significantly reduced weight gain to 2·39 (sem 0·36) g/d in HF animals (Fig. 3(a)).

Fig. 3 Effect of chronic caffeine intake (1 g/l) on body-weight increment, calculated as total weight variation during the experimental period (a), visceral fat, weighed post-mortem and corrected to body weight (b) and serum NEFA (c) in control, high-fat (HF) and high-sucrose (HSu) diet rats. (□), Without caffeine (n 10–12, n 9–12 and n 9–12 for body-weight increment, visceral fat and NEFA, respectively); (■), with caffeine (n 12–16, n 9–13 and n 10–15 for body-weight increment, visceral fat and NEFA, respectively). Values are means, with standard errors represented by vertical bars. Mean value was significantly different from that of the control rats not given caffeine: ** P < 0·01, *** P < 0·001 (two-way ANOVA with Bonferroni multiple comparison test). ††† Mean value was significantly different from that of the rats within the same diet group not given caffeine (P < 0·001; two-way ANOVA with Bonferroni multiple comparison test).
The HF diet significantly increased visceral fat compared with control animals (control = 8·48 (sem 0·63) g/kg; HF = 12·70 (sem 0·64) g/kg) while the HSu diet did not (10·99 (sem 0·86) g/kg). Caffeine consumption in control and HSu animals did not significantly modify visceral fat (Caff = 6·91 (sem 0·40) g/kg; HSuCaff = 9·91 (sem 0·97) g/kg) but the effect was significant in the HFCaff group (7·80 (sem 0·90) g/kg), a value similar to control (Fig. 3(b)). Fig. 3(c) shows that while the HF diet did not alter serum NEFA (NEFA control = 389·14 (sem 40·45) μm; NEFA HF = 436·45 (sem 36·23) μm), the HSu diet significantly increased NEFA to 940·62 (sem 89·66) μm. Chronic caffeine intake did not modify NEFA either in control or in HF animals (NEFA Caff = 406·57 (sem 29·55) μm; NEFA HFCaff = 391·67 (sem 38·23) μm); however, it significantly decreased NEFA in the HSu group to 610·24 (sem 41·06) μm. The correlations between both KITT and visceral fat and KITT and NEFA, with and without caffeine treatment, are depicted in Fig. 4. We observed a statistically significant negative correlation between KITT and visceral fat (r − 0·467; P = 0·0071) that is lost upon chronic caffeine treatment (r − 0·181; P = 0·314; Fig. 4(a)). Similarly, a statistically significant correlation was observed between KITT and serum NEFA (r − 0·444; P = 0·014) and this correlation was lost in the caffeine-treated groups (r − 0·148; P = 0·338; Fig. 4(b)).

Fig. 4 Effect of chronic caffeine intake (1 g/l) on the correlations between insulin sensitivity, visceral fat and NEFA in all the animals tested. (a) Caffeine eliminates the correlation between constant rate for glucose disappearance (KITT) and visceral fat mass. (b) Caffeine eliminates the correlation between KITT and serum NEFA. Pearson correlation coefficient was in (a): r − 0·467, P = 0·0071 without caffeine (□, - - -) and r − 0·181, P = 0·314 with caffeine (■, ––); in (b): r − 0·444, P = 0·014 without caffeine (Δ, - - -) and r − 0·148, P = 0·338 with caffeine (▲, - - -). Data represent all the individual samples obtained from control, high-fat and high-sucrose diet rats.
Effect of chronic caffeine intake on total hepatic glutathione and plasma NO/NO3− concentration
Fig. 5(a) shows that the HF and HSu diets significantly decreased the total hepatic glutathione content by 35·37 and 70·40 %, respectively, from a control value of 75·3 (sem 3·85) nmol/mg tissue. Chronic caffeine administration did not significantly modify hepatic glutathione + glutathione disulfide (GSSG) levels (Caff = 83·6 (sem 7·46) nmol/mg tissue; HFCaff = 59·1 (sem 7·55) nmol/mg tissue; HSuCaff = 35·3 (sem 3·28) nmol/mg tissue).

Fig. 5 Effect of chronic caffeine intake (1 g/l) on biomarkers of oxidative status. (a) Effect of caffeine on total hepatic glutathione in control rats and in rats submitted to high-fat (HF) and high-sucrose (HSu) diets. (b) Effect of caffeine on plasma NO/NO3− in control, HF and HSu rats. (□), Without caffeine (n 8–11 and n 8–13 for glutathione and NO, respectively); (■), with caffeine (n 8–12 and n 6–12, for glutathione and NO, respectively). Values are means, with standard errors represented by vertical bars. Mean value was significantly different from that of the control rats not given caffeine: **P < 0·01, ***P < 0·001 (two-way ANOVA with Bonferroni multiple comparison test).
Fig. 5(b) represents the effect of chronic caffeine intake on plasma NO/NO3−. The HF diet caused a significant increase in plasma NO/NO3− compared with control animals, whereas the HSu diet did not (control, 15·4 (sem 1·0) μm; HF, 24·1 (sem 2·4) μm; HSu, 12·9 (sem 1·0) μm). Caffeine intake did not modify plasma NO/NO3− in any of the groups tested (Caff, 17·3 (sem 0·7) μm; HFCaff, 22·0 (sem 1·8) μm; HSuCaff, 14·8 (sem 1·2) μm).
Effect of chronic caffeine intake on serum catecholamines
The effect of chronic caffeine intake on serum catecholamines (adrenaline plus noradrenaline) in control, HSu and HF rats is represented in Fig. 6(a). The HSu and HF diets significantly increased plasma catecholamines by 211·9 and 124·1 %, respectively, from a control value of 50·03 (sem 6·91) μm. Chronic caffeine intake did not modify plasma catecholamines in control animals; however, when administered together with the HSu and HF diets, caffeine prevented the increase in circulating catecholamines, suggesting that the metabolic and haemodynamic effects of chronic caffeine intake are mediated by a decrease in sympathetic activation. Moreover, KITT was negatively correlated with plasma catecholamines both in the absence (r − 0·399; P = 0·0484) and presence (r − 0·419; P = 0·009) of caffeine treatment (Fig. 6(b)).

Fig. 6 Effect of chronic caffeine intake (1 g/l) on plasma catecholamine concentration in control, high-sucrose (HSu) and high-fat (HF) diet rats. (a) Effect of caffeine on catecholamine (adrenaline plus noradrenaline) plasma levels. (□), Without caffeine (n 10–16); (■), with caffeine (n 12–16). Values are means, with standard errors represented by vertical bars. Mean value was significantly different from that of the control rats not given caffeine: **P < 0·01, ***P < 0·001 (two-way ANOVA with Bonferroni multiple comparison test). Mean value was significantly different from that of the rats within the same diet group not given caffeine: †† P < 0·01, ††† P < 0·001 (two-way ANOVA with Bonferroni multiple comparison test). (b) Caffeine treatment does not change the correlation between constant rate for glucose disappearance (KITT) and circulating catecholamine (adrenaline plus noradrenaline) plasma levels. Pearson correlation coefficient was r − 0·399, P = 0·0484 without caffeine (○) and r − 0·419, P = 0·009 with caffeine (●). Data represent all the individual samples obtained from control, HF and HSu rats.
Effect of carvedilol on insulin sensitivity and mean arterial pressure
There were no significant changes in individual daily liquid intake per kg in any of the groups tested. Food intake was also not significantly different (data not shown). In the HFMC group there was a decrease in KITT from a control value of 4·90 (sem 0·26) % glucose/min to 3·50 (sem 0·23) % glucose/min. The HSuMC diet decreased KITT to 3·35 (sem 0·19) % glucose/min. CVD did not modify insulin sensitivity in control animals (KITT CVD = 4·68 (sem 0·30) % glucose/min) but it completely reversed the insulin resistance induced by the diets (KITT HFCVD = 4·64 (sem 0·27) % glucose/min; KITT HSuCVD = 5·20 (sem 0·12) % glucose/min) (Fig. 7(a)). CVD significantly decreased fasting glycaemia in the HSuMC group from 1165 (sem 45·6) to 919 (sem 27·5) mg/l. Both the HFMC and HSuMC diets caused a significant increase in plasma insulin levels in comparison with the MC group and this effect was reversed by CVD administration (MC = 2·45 (sem 0·26) μg/l; HFMC = 4·88 (sem 0·30) μg/l; HSuMC = 4·36 (sem 0·37) μg/l; HFCVD = 2·42 (sem 0·42) μg/l; HSuCVD = 1·76 (sem 0·79) μg/l). We also observed that CVD completely restored MAP to control values in HF and HSu rats, as expected due to its anti-hypertensive action (MC = 95·6 (sem 3·79) mmHg; CVD = 97·2 (sem 3·35) mmHg; HFMC = 113·0 (sem 4·07) mmHg; HFCVD = 99·4 (sem 4·59) mmHg; HSuMC = 113·6 (sem 3·19) mmHg; HSuCVD = 99·0 (sem 5·17) mmHg).

Fig. 7 Effect of carvedilol (CVD, 0·2 g/l) and its vehicle, methylcellulose (MC, 0·5 %), on insulin sensitivity and plasma catecholamines in control, high-fat (HF) and high-sucrose (HSu) diet rats. (a) The effect of CVD on insulin sensitivity was determined by the insulin tolerance test and is expressed as constant rate for glucose disappearance (KITT). (□), MC (n 10–17); (■), CVD (n 7–12). Values are means, with standard errors represented by vertical bars. Mean value was significantly different from that of the control rats given MC: *P < 0·05, **P < 0·01 (two-way ANOVA with Bonferroni multiple comparison test). Mean value was significantly different from that of the rats within the same diet group given MC: †P < 0·05, †††P < 0·001 (two-way ANOVA with Bonferroni multiple comparison test). (b) Effect of CVD on catecholamine (adrenaline plus noradrenaline) plasma levels. (□), MC (n 10–36); (■), CVD (n 5–7). Values are means, with standard errors represented by vertical bars. * Mean value was significantly different from that of the control rats given MC (P < 0·05; two-way ANOVA with Bonferroni multiple comparison test).
Effect of carvedilol on serum catecholamines
The HSuMC and HFMC groups showed a significant increase in plasma catecholamines of 112·4 and 97·9 %, respectively, from a control value of 58·53 (sem 8·88) μm in the MC group. In contrast with caffeine, CVD treatment did not modify plasma catecholamines in any of the groups tested (CVD, 59·2 (sem 17·80) μm; HFCVD, 108·6 (sem 33·14) μm; HSuCVD, 88·8 (sem 32·26) μm; Fig. 7(b)). CVD administration did not significantly modify weight gain, visceral fat, NEFA, hepatic glutathione or NO/NO3− in any of the disease models tested (data not shown).
Discussion
We demonstrated in the present study that chronic caffeine intake prevents the development of insulin resistance and hypertension in high-fat- and high-sucrose-fed rats and induces a pronounced decrease in circulating adrenaline and noradrenaline. Also, the effect of CVD, an antagonist of β1, β2 and α1 adrenoceptors, on insulin sensitivity mimicked the effect of caffeine, supporting the hypothesis that caffeine's beneficial effects are mediated by the inhibition of the sympathetic nervous system.
Chronic caffeine treatment did not significantly change fasting glycaemia both in the control and HF groups, which were normoglycaemic without caffeine treatment(Reference Kim, Ellmerer and Van Citters33), but it totally reversed the hyperglycaemia induced by the HSu diet. Also, in contrast with the acute effects of caffeine on insulin secretion described in the literature(Reference Greenberg, Boozer and Geliebter12), the present results show that plasma insulin levels significantly decreased in the HFCaff group and trend towards a decrease in the HSuCaff group compared with the HF and HSu groups, respectively. In the HFCaff group the reduction of fasting insulinaemia, accompanied by an increase in KITT, indicates that chronic caffeine not only increased insulin sensitivity but also normalised insulin secretion in the HF animals. We do not know at this point if the decrease in plasma insulin levels is a direct effect of caffeine on the pancreas, or simply a negative-feedback mechanism induced by improved peripheral insulin sensitivity at the β-cell. However, previous studies in human subjects have described that caffeinated coffee consumption leads to a reduction of plasma C-peptide concentrations in healthy and in obese women, suggesting that coffee components decrease insulin secretion(Reference Wu, Willett and Hankinson16) and in vitro studies also showed that adenosine regulates insulin secretion by the β-cell(Reference Ismail, El Denshary and Montague34).
In contrast with the HFCaff animals, in the HSuCaff group caffeine restored insulin sensitivity and normoglycaemia but the animals remained hyperinsulinaemic compared with controls. This suggests that in the caffeine-treated sucrose-fed model, insulin action improved sufficiently to maintain glucose homeostasis but not enough to normalise endogenous insulin secretion.
In the HF rats both visceral fat and weight gain increased significantly and caffeine reversed these effects, in agreement with the literature(Reference Greenberg, Boozer and Geliebter12). Weight loss and visceral fat mass decrease induced by long-term caffeine consumption may have been due to increased lipolysis accompanied by increased NEFA oxidation, since circulating NEFA were not elevated compared with control animals. We observed no changes in weight gain or visceral fat mass in the HSu rat treated with caffeine. These results indicate that increased adipose tissue mass is not contributing to insulin resistance in this animal model, which is in accordance with previous results by Kanazawa's group(Reference Kanazawa, Xue and Kageyama35).
In contrast to the HF diet, the HSu diet increased circulating NEFA, indicating augmented lipolysis or decreased NEFA oxidation in the sucrose-fed rats as previously described by others(Reference Delarue and Magnan36). These results may also be due to an increased de novo lipogenesis in adipocytes and liver in this model which, combined with insulin resistance, may lead to enhanced lipolysis and systemic NEFA(Reference Lewis, Carpentier and Adeli37). We have observed that chronic caffeine intake reversed the increase in NEFA induced by the HSu diet. This effect may be related to an augmented activity of 5′-AMP activated protein kinase (AMPK) induced by caffeine that promotes an enhancement in NEFA uptake and oxidation in skeletal muscle(Reference Egawa, Hamada and Kameda38, Reference Raney and Turcotte39). Recently, it has also been shown that caffeine can increase GLUT-4 mRNA in skeletal muscle cells and insulin-independent glucose transport mediated by AMPK(Reference Egawa, Hamada and Kameda38), which may explain the return to normal glycaemia observed in the caffeine-treated rats. Despite the beneficial effects of caffeine on visceral fat in the HF-fed rat and in circulating NEFA in the HSu rat, the correlation analyses show that insulin action loses its dependence of these variables after caffeine treatment.
The insulin-sensitising effect of chronic caffeine treatment may also be directly related to antagonism of adenosine receptors on insulin target tissues. The effect of adenosine on glucose homeostasis is currently a hot-topic area with controversial results: while positive effects of A1 receptor partial agonists are well documented on adipose tissue glucose uptake(Reference Dhalla, Chisholm and Reaven40), studies using adenosine receptor antagonists have shown increases(Reference Espinal, Challiss and Newsholme41), decreases(Reference Han, Hansen and Nolte42) and no effect(Reference Vergauwen, Hespel and Richter43) of these drugs on skeletal muscle glucose uptake. Also, A2B receptor antagonists have been used to increase plasma insulin levels in vivo (Reference Rusing, Muller and Verspohl44) and more recently to reduce insulin resistance(Reference Figler, Wang and Srinivasan45). Clearly, more studies are required to clarify the role of adenosine receptors on glucose homeostasis.
In the present study we also demonstrated that chronic caffeine intake prevents the development of hypertension in HF- and HSu-fed animals. Recently, it has been shown that endothelium-dependent vasodilatation after long-term caffeine administration is enhanced in young healthy men(Reference Umemura, Ueda and Nishioka46), a mechanism by which caffeine treatment could lower blood pressure. However, we did not find any effect of chronic caffeine consumption on NO production, suggesting that NO is involved neither in the prevention of hypertension nor in insulin resistance induced by caffeine. It has also been suggested that caffeine may prevent oxidative damage(Reference Scharf, Prustomersky and Huber47, Reference Varma and Hegde48) which could improve endothelium-dependent vasodilation and insulin action. However, in our experimental setting we observed no changes in total hepatic glutathione, both in HF- and HSu-treated animals. Hepatic glutathione stores are significantly depleted after exposure to oxidative stress(Reference Mytilineou, Kramer and Yabut49) and if administration of caffeine produced significant antioxidant effects, we would expect intracellular hepatic glutathione levels to rise, which did not happen.
Chronic caffeine treatment caused a decrease in circulating catecholamines, suggesting that decreased sympathetic nervous system activation, either at the adrenal medulla or at the sympathetic nerves innervating blood vessels, is involved in the protective effects of caffeine. Adenosine receptor agonists inhibit sympathetic neurotransmission and catecholamine release in several tissues, including the hippocampus(Reference Jackisch, Fehr and Hertting50) and adrenal chromaffin cells(Reference Tseng, Chan and Lo51), when administered acutely. Adenosine receptor antagonists, such as caffeine, increase plasma renin activity, adrenaline and noradrenaline(Reference Robertson, Frolich and Carr52) after acute administration; however, in accordance with the present results, it has been shown that the increase in adrenaline levels induced by a single dose of caffeine is lost with sustained consumption(Reference Debrah, Haigh and Sherwin53).
The present results also show that the adrenergic antagonist, CVD, mimicked the effects of caffeine. As observed with caffeine treatment, CVD totally reversed the hyperglycaemia induced by the HSuMC diet. Plasma insulin levels significantly decreased after CVD treatment in the HFCVD and HSuCVD groups, in contrast to the hypoinsulinaemic effect of caffeine that was observed in the HFCaff but not in the HSuCaff group. Others have also reported that CVD, among other third-generation β-blockers, lack adverse metabolic effects and may actually produce favourable effects on insulin sensitivity(Reference Carella, Antonucci and Conte54, Reference Bhatt, Makwana and Santani55). The mechanisms behind these findings are not yet fully understood but the role of the α1 and β2 adrenoceptor blocking effects of CVD appears to be crucial(Reference Kveiborg, Christiansen and Major-Petersen56). While caffeine significantly decreased weight gain and visceral fat mass in HF rats and circulating NEFA in HSu rats, CVD treatment did not change any of these factors in the experimental groups, although the effect of both drugs on insulin sensitivity was very similar. These results are consistent with the hypothesis that the insulin-sensitising properties of both caffeine and CVD are not directly related with changes in adipose tissue homeostasis. Finally, CVD has also been reported to increase endogenous NO production(Reference Corsetti, Pasini and Assanelli57, Reference Ofluoglu, Pasaoglu and Pasaoglu58), affecting endothelium-dependent vasodilation, a mechanism by which this drug could increase insulin sensitivity(Reference Guarino and Macedo59, Reference Guarino, Correia and Raposo60). We did not find any effect of CVD on NO production in our experimental conditions, suggesting that NO is involved neither in the prevention of hypertension nor in insulin sensitisation induced by CVD.
In conclusion, chronic caffeine administration prevents the development of insulin resistance and hypertension in high-fat- and high-sucrose-fed animals due to a decrease in circulating catecholamines. Treatment with an adrenergic antagonist mimics the protective effects of caffeine against diet-induced insulin resistance by blockade of adrenergic receptors without changes in serum catecholamine concentration. The exact biological mechanism underlying the decrease in circulating catecholamines induced by caffeine remains to be clarified and may involve alterations in the expression of adenosine receptors as well as in the expression of other neurotransmitter receptors and ionic channels(Reference Shi and Daly61, Reference Stonehouse, Adachi and Walcott62).
In conclusion, we have shown that long-term caffeine administration has a protective effect on the development of diet-induced insulin resistance and hypertension in rats. Our work strengthens the conclusions of recent epidemiological studies concerning the protective role of coffee consumption in type 2 diabetes and the metabolic syndrome and helps to support the need for a paradigm shift on advised lifestyle changes, like coffee withdrawal, in insulin-resistant and hypertensive patients.
Acknowledgements
We are very grateful to Elena Gonzalez Muñoz for catecholamine quantification and to Inês Faustino, Ana Carvalho and Lúcia Mirrado for technical support and ‘L'Oréal-Unesco Awards for Women in Science 2009 – Portugal’.
The present study was financially supported by CEDOC/FCT, Portuguese Society of Diabetology (SPD)/Lilly Portugal, BFU2007-61848 and JCyL-GR242, and by L'Oréal-Unesco Awards for Women in Science 2009 – Portugal.
S. V. C. and M. P. G. designed and conducted the study and also wrote the manuscript. T. N. da S. participated in data collection and analysis, M. M. C. and E. C. M. contributed in data interpretation. Catecholamine quantification was performed in the laboratory of C. G.
There are no conflicts of interest.