Starvation produces a series of metabolic changes that lead to a reduction in body weight, alterations in body composition and metabolic gene expression(Reference Robin, Decrock and Herzberg1, Reference Li, Zhang and Liu2). In mammals and birds, three distinct levels of energy depletion have been established(Reference Cherel, Robin and Maho3–Reference Le Maho, Vu Van Kha and Koubi10). The first phase (phase 1) is a rapid period of adaptation marked by an increase in mobilisation of fat stores and a lowering in protein utilisation. During the second phase (phase 2), which is a long period of thrift, most of the energy expenditure is derived from fats, and then fat stores are progressively exhausted, while body proteins are efficiently spared. The third phase (phase 3) is characterised by an increase in protein utilisation. In humans, the negative energy balance resulting from starvation can arise due to disease, eating or psychological disorders, or hunger strikes. Starvation and consequent re-feeding syndrome can lead to electrolyte disorders, especially hypophosphataemia, along with neurological, pulmonary, cardiac, neuromuscular and haematological complications(Reference Kraft, Btaiche and Sacks11). To avoid the re-feeding syndrome, an additional load of vitamins has been suggested to correct the vitamin deficiencies(Reference Kraft, Btaiche and Sacks11). However, little is known about B-group vitamin status during starvation.
Several B-group vitamins take part in energy metabolism. For instance, vitamin B2 functions as FAD and FMN in redox reactions including the electron transport chain and fatty acid oxidation. Nicotinamide is involved in more than 200 reactions, including the metabolism of carbohydrates, amino acids and fatty acids, and also in the electron transport chain. Vitamin B1 catalyses carbohydrate metabolism including decarboxylation of α-ketoacids and trans-ketolation as a cofactor thiamin diphosphate; vitamin B6 functions as pyridoxal 5′-phosphate in amino acid metabolism including aminotransferases, decarboxylases, racemases and dehydratases as pyridoxal 5′-phosphate; and pantothenic acid is involved in fatty acid metabolism such as oxidation and synthesis. For these reasons, in the ‘Dietary Reference Intakes for Japanese, 2010’, dietary requirements for vitamin B1, vitamin B2 and niacin are expressed per 4186 kJ (1000 kcal), and the requirement for vitamin B6 is expressed in terms of protein intake(12).
As mentioned earlier, prolonged starvation sifts the energy source from glucose to fats and then to protein, and B-group vitamins are involved in the metabolism of carbohydrates, fatty acids and amino acids. Thus, in the present study, we investigated how changes in energy metabolism altered B-group vitamin utilisation during starvation. We comprehensively determined eight kinds of B-group vitamin concentrations in rat blood, urine and tissues including the brain, heart, lung, stomach, kidney, liver, spleen, testis and skeletal muscle during 8 d of starvation.
Materials and methods
Diets
The composition of the purified diet is shown in Table 1. Vitamin-free milk casein, l-methionine and sucrose were purchased from Wako Pure Chemical Industries Limited (Osaka, Japan). Maize oil was purchased from Nisshin OilliO Group, Limited (Tokyo, Japan). Gelatinised maize starch, the mineral mixture (AIN-93G) and the vitamin mixture (AIN-93VX) were obtained from Oriental Yeast Company, Limited (Tokyo, Japan).
Table 1 Composition of the diet
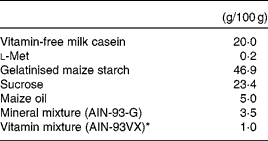
* The composition of the vitamin mixture is described by Reeves et al. (Reference Reeves, Nielsen and Fahey13).
Animals
Male rats of the Wistar strain, weighing 225–235 g, were obtained from CLEA Japan, Inc. (Tokyo, Japan). The rats were individually housed in a temperature-controlled room (22 ± 2°C and 50–60 % humidity) with a 12 h light–12 h dark cycle and were allowed to acclimate to the environment for 7 d before starting the experiment. Body mass, food consumption and water intake were recorded daily ( ± 0·1 g). We also collected 24 h urine samples every day.
Experimental procedures
A total of twenty-five rats were randomly divided into five groups. After 1 week of acclimatisation, five rats were killed by decapitation as a control group (CONT, n 5). The other rats were deprived from food for 1 d (S1, n 5), 2 d (S2, n 5), 6 d (S6, n 5) or until they had been in phase 3 for 2 d; that is, they were starved for a total duration of 6–9 d (P3, n 5). The starving phase was determined by calculating the specific daily rate of body mass loss dM/Mdt (g/kg per d) for each animal (dM represents the loss of body mass during dt = t 1-t 0 and M is the body mass of the rat at t 0 (Reference Le Maho, Vu Van Kha and Koubi10, Reference Habold, Chevalier and Dunel-Erb14). Blood was taken from the tail vein at 09.00 hours every day, and 3-hydroxybutyric acid (3-HBA) concentration in the blood was measured with a 3-HBA Kit (Abbott Japan Company, Limited, Tokyo, Japan) to confirm the metabolic state of each animal because blood 3-HBA reflects fatty acid oxidation.
After the animals were killed, blood samples were collected into EDTA-2K tubes from the carotid artery and were centrifuged at 1700 g for 10 min at 4°C. Plasma glucose, TAG, urea N, aspartate aminotransferase and alanine aminotransferase were measured with FUJI DRI-CHEM (FUJIFILM Company, Tokyo, Japan).
The cerebrum, heart, lungs, stomach, kidneys, liver, spleen, testes and leg muscles were dissected and weighed ( ± 0·001 g). The stomach was cleared of its contents. All tissue samples were immediately homogenised in ultra-pure water at 1:10 (w/v) using a Teflon glass homogeniser and stored at − 20°C until needed. The present study was conducted according to the guidelines for the care and use of laboratory animals, and was approved by the Ethics Committee of the University of Shiga Prefecture (Shiga, Japan).
Analytical methods
Vitamin B1. Thiamin in urine was measured directly. The vitamin B1 content in the blood and tissue was determined as the sum of thiamin, thiamin monophosphate and thiamin diphosphate and was expressed as total thiamin. TCA (5 %) was added to whole blood and tissue homogenates, and the blood and homogenates were centrifuged for 5 min at 20 000 g, and the supernatant of the mixture was used for measurement. Vitamin B1 levels in the urine, blood and tissue were determined by the HPLC post-labelled fluorescence method(Reference Fukuwatari, Suzuura and Sasaki15).
Vitamin B2. Riboflavin in urine was measured directly by HPLC(Reference Ohkawa, Ohishi and Yagi16). Riboflavin, FMN and FAD in blood and tissue were converted to lumiflavin by photolysis. Briefly, the supernatant from a TCA-treated blood or tissue sample was added to an equal volume of 1 m-NaOH. The alkalised mixture was irradiated with a fluorescent lamp for 30 min, and acetic acid was added to the mixture. The neutralised mixture was filtered with a 0·45 μm microfilter and the filtrate was directly injected into the HPLC system for the measurement of lumiflavin(Reference Ohakawa, Ohishi and Yagi17). The measured lumiflavin was expressed as total vitamin B2.
Vitamin B6. 4-Pyridoxic acid, a catabolite of vitamin B6, in urine was measured directly by HPLC(Reference Gregory and Kirk18). Serum pyridoxal and pyridoxal 5′-phosphate were determined by the HPLC method(Reference Rybak and Pfeiffer19). Vitamin B6 vitamers, including phosphate esters in the tissue, were converted to free vitamin B6 vitamers such as pyridoxal and pyridoxamine using an autoclave under acidic conditions. Briefly, the homogenate was added to 0·06 m-HCl at 1:8 (v/v) and autoclaved at 121°C for 3 h, and the mixture was adjusted to pH 5·0 using 1 m-NaOH. These were measured as total vitamin B6 by the microbioassay method using Saccharomyces carlsbergensis strain 4228 ATCC 9080(20).
Vitamin B12. Urine, plasma and tissue homogenates were added to a 0·2 mm-acetate buffer (pH 4·8) with 0·0006 % potassium cyanide. These were put into a boiling water bath for 5 min to be converted to cyanocobalamin, and then 10 % metaphosphoric acid was added to be neutralised. Cyanocobalamin was determined by the microbioassay method using Lactobacillus leichmannii ATCC 7830(Reference Watanabe, Katsura and Takenaka21).
Niacin. Nicotinamide(Reference Shibata, Kawada and Iwai22) and its catabolites, N 1-methylnicotinamide (MNA)(Reference Shibata23), N 1-methyl-2-pyridone-5-carboxamide (2-Py) and N 1-methyl-4-pyridone-3-carboxamide (4-Py)(Reference Shibata, Kawada and Iwai22), in urine were measured directly by HPLC. For measuring the total nicotinamide content in blood and tissues, the whole blood and tissue homogenates were autoclaved at 121°C for 20 min to convert the coenzymes to nicotinamide. The resulting nicotinamide was then determined by the HPLC method(Reference Shibata, Kawada and Iwai22, Reference Shibata24).
Pantothenic acid. Pantothenic acid in urine was determined by HPLC(Reference Takahashi, Fukuwatari and Shibata25). To digest the bound pantothenic acid including coenzyme A and phosphopantetheine in tissue and plasma to free form, the homogenate or blood was incubated at 37°C for 24 h. Pantothenic acid in the plasma and tissue was determined by the microbioassay method using Lactobacillus plantarum ATCC 8014(Reference Skeggs and Wright26).
Folate. Folate in urine and plasma was directly determined by the microbioassay method using Lactobacillus casei ATCC 27 773(Reference Aiso and Tamura27). Folate in tissues was digested to monoglutamate forms by treatment with protease and conjugase. Briefly, 1 m-KH2PO4–K2HPO4 buffer (pH 6·1) was added to the tissue homogenate at 1:9 (v/v), and the homogenate was autoclaved at 121°C for 5 min. Proteinase MS (Kaken Pharmaceutical Company, Limited, Tokyo, Japan) was added to the homogenate at a final concentration of 2·5 mg/ml and then incubated at 37°C for 3 h. The reaction mixture was added to the conjugase solution (extract from porcine kidney acetone powder, Sigma, Porcine, Type II) at 30:1 (v/v) and incubated at 37°C for 12 h. After centrifugation at 10 000 g for 10 min, the supernatant was used for determination by the microbioassay.
Biotin. Bound biotin in tissues was converted to the free form using autoclave under acidic conditions. Briefly, 1·5 m-H2SO4 was added to the homogenate at 1:1 (v/v), and the homogenate was autoclaved for 1 h at 121°C. The suspension was centrifuged at 10 000 g for 10 min at 4°C, and the supernatant was used to measure biotin. Biotin in urine and plasma was measured directly. The biotin content in urine, plasma and tissue was determined by the microbioassay method using L. plantarum ATCC 8014(Reference Fukui, Iinuma and Oizumi28).
Statistical analysis
Values are expressed as means with their standard errors. P3 rats (starved for 6–9 d) were expressed at 8 d on the graph for convenience. To test the significance of the differences in mean values among all groups, one-way ANOVA with Tukey's post hoc test was employed. Repeated ANOVA with Bonferroni's post hoc test was used to analyse urinary excretion of B-group vitamins in P3 rats, and individual data points were compared with their data at day 0. All differences at P <0·05 were considered to be statistically significant. Prism software (version 5; obtained from GraphPad Software, Inc., San Diego, CA, USA) was used for all analyses.
Results
Changes in body mass during starvation
Changes in body mass during starvation are shown in Table 2. Starvation for the first 24 h produced a weight loss of 7 %. From the second day to the last day of starvation, the rats lost 5 % weight for each 24 h (data not shown). The specific daily rate of body mass loss (dM/Mdt) v. time in starved rats is presented in Fig. 1. The pattern of dM/dMt showed a sharp decrease during the first hours of starvation and a steady rate at days 2–6 of starvation. Since obvious rapid increase was not observed after day 7 of starvation, we showed that the last part of starvation was counted to the end of starvation in the P3 group. The dM/dMt in the P3 group clearly showed a rapid increase from 3 d before the end of starvation. These patterns were exactly consistent with previous reports(Reference Le Maho, Vu Van Kha and Koubi10, Reference Habold, Chevalier and Dunel-Erb14). Therefore, we defined the phase at first decrease as phase 1, that of steady rate as phase 2 and the third part of the curve as phase 3 according to previous reports(Reference Le Maho, Vu Van Kha and Koubi10, Reference Habold, Chevalier and Dunel-Erb14). The S6 group showed the steady rate of body mass loss and the low blood 3-HBA concentration, and these were characteristics of both phases 2 and 3. These results showed that the S1 group was representative of phase 1, the S2 was of phase 2, the S6 group was in the marginal range between phases 2 and 3, and the P3 group was in phase 3.
Table 2 Body mass and organ mass in the control and starved rats
(Mean values with their standard errors, n 5)

CONT, non-starved control rats; S1, 1-day starved rats; S2, 2-day starved rats; S6, 6-day starved rats; P3, starved to phase 3 rats.
a,b,c Mean values within a row with unlike superscript letters were significantly different determined by one-way ANOVA with Tukey's multiple comparison tests (P < 0·05).
* Since the control rats were killed at the beginning of the experiment, the initial body weight was same as the final body weight.
† Phase 3 is determined by the rapid increase in dM/dMt (refer to Fig. 1).

Fig. 1 Rate of body mass loss (dM/Mdt) in starved rats. Values are means with their standard errors (n 1–20 per d in the left; n 5 per d in the right). dM/Mdt (dM represents the loss of body mass during dt = t 1 − t 0 and M is the body mass of rat at t 0) was calculated for each animal. Abscissa: left, days counted from the beginning of starvation in all rats; right, counted to the end of starvation in the P3 group.
Changes in mass of individual organs during starvation
Table 2 shows the changes in the mass of individual organs during starvation. The cerebrum, lung and stomach mass was not affected by starvation. The liver weight was gradually reduced by starvation, and that in the P3 group was 30 % of the control group. From 2 d of starvation, kidney mass decreased. Heart and spleen mass decreased from 6 d. Testes mass decreased in P3 rats. Prolonged starvation reduced the spleen and liver weight the most.
Blood/plasma parameters
Table 3 shows the blood parameters. Blood 3-HBA increased more in S1 and S2 rats than in control rats. In contrast, the urea concentration in plasma was significantly higher in S6 and P3 rats, whereas there was a non-significant increase in the S1 and S2 rats. Plasma glucose level was 60 % significantly lower in the S1, S2 and S6 rats than in the control rats. Interestingly, plasma glucose returned to the basal level in the P3 rats. Plasma TAG was dramatically decreased after 1 d of starvation and then continued to decrease gradually throughout the remainder of the starvation period. Plasma aspartate aminotransferase was not affected by starvation. Plasma alanine aminotransferase began to increase after 6 d of starvation.
Table 3 Blood parameters in the control and starved rats
(Mean values with their standard errors, n 5)

CONT, non-starved control rats; S1, 1-day starved rats; S2, 2-day starved rats; S6, 6-day starved rats; P3, starved to phase 3 rats; 3-HBA, 3-hydroxybutyrate; AST aspartate aminotransferase; ALT, alanine aminotransferase.
a,b Mean values within a row with unlike superscript letters were significantly different determined by one-way ANOVA with Tukey's multiple comparison tests (P < 0·05).
* 3-HBA was measured in whole blood, and the others in serum.
Effect of starvation on vitamin status
Table 4 shows B-group vitamin content in tissue, blood and urine in the control rats. We determined the B-group vitamin contents in nine tissues including the cerebrum, heart, lung, stomach, kidney, spleen, testis, skeletal muscle and liver, and five tissues were selected as representative variations in Fig. 2.
Table 4 Contents of B-group vitamins in each tissue, blood and urine of control rats
(Mean values with their standard errors, n 4–5)

WB, whole blood.

Fig. 2 Relative value of B-group vitamin concentrations in (A) cerebrum, (B) stomach, (C) kidney, (D) skeletal muscle, (E) liver and (F) blood of rats during starvation. * Sum of serum pyridoxal and pyridoxal-5′-phosphate is expressed as serum vitamin B6. Values are reported as means with their standard errors, n 5 per d. Values of control rats are expressed as 100 %. P3 is expressed at 8 d of starvation. Means with unlike letters were significantly different from day 0 in avitamin B1 (○), bvitamin B2 (●), cvitamin B6* (Δ), dvitamin B12 (▲), enicotinamide (□), fpantothenic acid (■), gfolate ( × ) and hbiotin (+; P < 0·05).
Cerebrum ( Fig. 2(A)) and spleen. With the exception of biotin, all vitamin concentrations were unchanged by starvation. Biotin concentration was initially elevated to 150 % in the S1 rats, and then returned to basal level. B-group vitamin concentrations in the spleen showed a similar pattern that starvation did not affect their concentrations except for vitamin B2. Vitamin B2 concentration in the testis was elevated to 130 % after 6 d of starvation.
Heart ( Fig. 2(B)). Vitamin B1 and folate concentrations significantly decreased to approximately 60 % after 6 d of starvation. Pantothenic acid concentration was significantly lower in the S2 and S6 rats than in the control rats. Biotin and vitamin B6 concentrations significantly increased to 160 and 250 % in the S1 and S6 rats, respectively. The other B-group vitamin concentrations were unchanged.
Kidney ( Fig. 2(C)) and testis. Pantothenic acid concentration dramatically decreased to 50 % of control during starvation. Folate concentration significantly decreased to 40 % in the S6 and P3 rats. Vitamin B2 concentration significantly decreased to 70 % in the P3 rats. The reduction in pantothenic acid concentration from day 1 of starvation was also observed in the stomach and testis, and their maximal reduction was 60 %. In the testis, vitamin B2 concentration also significantly reduced to 50 % during starvation, and other vitamin concentrations were not changed.
Skeletal muscle ( Fig. 2(D)) and stomach. Concentrations of vitamin B1, vitamin B2 and vitamin B6 significantly decreased to 50 % from 2 d of starvation, but only vitamin B2 concentration returned to control levels in the P3 rats. Vitamin B12 concentration significantly decreased to 40 % only in the S2 rats. Pantothenic acid concentration significantly decreased in the P3 rats. Folate concentration significantly decreased to 50 % in the S6 and P3 rats. Pantothenic acid concentration was same as control until 6 d of starvation, and then dramatically decreased to 30 % in the P3 rats. Similar pattern was observed in the stomach that starvation reduced several B-group vitamin concentrations. In brief, pantothenic acid and biotin concentrations reduced to 30 and 60 % from day 1 of starvation, respectively. Vitamin B6 concentration significantly decreased to 50 % from day 2, and vitamin B2 did to 40 % from day 6 of starvation.
Liver ( Fig. 2(E)). Vitamin B6 concentration was significantly higher in the S1, S6 and P3 rats, and the relative value in the P3 rats was 160 % of the control animals. Nicotinamide concentration increased in the S2, S6 and P3 rats. Vitamin B1 and pantothenic acid concentrations were higher in the P3 rats, and their values were 150 and 250 %, respectively. Other B-group vitamins concentrations were unchanged.
Blood ( Fig. 2(F)). Whole blood vitamin B1, serum vitamin B6 and plasma pantothenic acid concentrations decreased in the S6 and P3 rats. Plasma folate and biotin concentrations decreased in the P3 rats. The relative values of vitamin B1 and vitamin B6 in the P3 rats were 60 % of control, those of folate and biotin were 50 %, and those of pantothenic acid were 30 %.
Urinary contents of B-group vitamins ( Fig. 3). Vitamin B1 excretion acutely decreased to 10 % after 1 d of starvation. Urinary excretion of riboflavin, pyridoxal metabolite 4-pyridoxic acid and vitamin B12 gradually decreased during 4 d of starvation. Subsequently these values were stable, at approximately 20, 20 and 50 % of each control value. Urinary folate was initially unchanged in the S1 rats and then decreased to 40 % of the baseline value. Urinary pantothenic acid was increased to 170 % in 3rd and 4th days of starvation, and then returned to the control level. Although biotin excretion increased to 460 % during the first 3 d of starvation, it subsequently returned to the basal level.

Fig. 3 Relative value of urinary B-group vitamin contents in P3 rats during starvation. Those of vitamin B1, vitamin B2, vitamin B6, vitamin B12, nicotinamide and folate are shown in (A), and pantothenic acid and biotin in (B). Thiamin is expressed as vitamin B1, riboflavin as vitamin B2, 4-pyridoxic acid as vitamin B6, and sum of nicotinamide and its catabolites as nicotinamide. Values are reported as means with their standard errors, n 5 per d. Values of control rats are expressed as 100 %. P3 is expressed at 8 d of starvation. Means with unlike letters were significantly different from day 0 in avitamin B1 (○), bvitamin B2 (●), cvitamin B6* (Δ), dvitamin B12 (▲), enicotinamide (□), fpantothenic acid (■), gfolate ( × ) and hbiotin (+; P < 0·05).
Urinary contents of nicotinamide and its catabolites ( Fig. 4). Nicotinamide excretion increased after 1 d of starvation and then returned to the basal level. 2-Py and 4-Py decreased after an initial increase on day 1. In contrast, MNA excretion increased during the starvation period. Urinary excretion of the sum of nicotinamide and its catabolites increased 1·4-fold after 1 d of starvation and then decreased by less than half of the food sufficient state.

Fig. 4 Relative value of urinary nicotinamide (a) and its catabolites MNA (b), 2-Py (c) and 4-Py (d) contents in P3 rats during starvation. Values are reported as means with their standard errors, n 5 per d. * Mean values were significantly different from day 0 determined by one-way ANOVA with Tukey's multiple comparison tests (P < 0·05). MNA, N 1-methylnicotinamide; 2-Py, N 1-methyl-2-pyridone-5-carboxamide; 4-Py, N 1-methyl-4-pyridone-3-carboxamide.
Discussion
The effects of metabolic changes, which are designated as the changes in the main energy sources such as glucose, lipids and protein, during starvation on the tissue and urine vitamin concentrations are currently poorly understood(Reference Kraft, Btaiche and Sacks11). Elucidation of the effects will lead to a suitable supplementation for preventing the refeeding syndrome. Therefore, we investigated the effects of short- and long-term starvation on the vitamin concentrations in organs, muscle, blood and urine in rats.
Vitamin concentrations in organs and muscle showed different patterns for each vitamin. For noticeable characteristics, biotin concentration, which means the value in terms of g tissue, was increased in most organs of the S1 rats. A part of the reason is a reduced organ mass at S1. It was unclear why the biotin concentrations in organs remained at the same level regardless of organ mass during starvation. Vitamin B1 is the vitamin that has the most rapid turnover(Reference Fukuwatari, Yoshida and Takahashi30), but the levels in the kidney were maintained. This may point to the necessity of vitamin B1 in kidneys of starving rats. In terms of the metabolic state, vitamin B1 was expected to decrease in the early days of starvation, because glucid is the main energy source in this period(Reference Cherel, Robin and Maho3). However, vitamin B1 concentrations in tissues and blood were stable in the S1 rats. This is due to the sharp decrease in liver weight and in the urinary excretion of vitamin B1. Along with the shifts in the main energy source from glucid to fat, vitamin demands appear to change. Next to vitamin B1, pantothenic acid requirement may be the highest because it is involved in the metabolism of fatty acids(Reference Wittwer, Beck and Peterson31), and also, biotin requirement may be higher because the gluconeogenesis is more active at the deficient state of glucose(Reference Bannister32). However, the present results were contrary to our expectations. Pantothenic acid concentrations in the heart, stomach, kidneys and testes were decreased in the S1 rats, and a similar phenomenon was observed in biotin concentrations. The urinary excretion of pantothenic acid and biotin was significantly increased by starvation. A similar phenomenon was already reported by Fukuwatari et al. (Reference Fukuwatari, Yoshida and Takahashi30). Shibata and co-workers reported(Reference Fukuwatari and Shibata33–Reference Tsuji, Fukuwatari and Sasaki38) that the urinary excretion of water-soluble vitamins reflects recent intake of the vitamins over the last few days, and in addition, the decreased urinary excretion of vitamins means the elevated demand for vitamins, whereas the increased urinary excretion of vitamins means the reduced demand for vitamins when their intake of vitamins is almost the same(Reference Fukuwatari and Shibata33). The increased urinary excretion suggests that the requirement of pantothenic acid and biotin was reduced by starvation, that is, lower concentrations of pantothenic acid and biotin in the body might prefer to live for a long time during starvation. A possible inferable reason for the increase in urinary pantothenic acid and biotin might be a mechanism to prevent the stored fat in the body from overspending or to decrease the amount of acetyl-coenzyme A, which modifies several functional proteins such as histone(Reference Alcarraz-Vizán, Boren and Lee39) and some enzymes(Reference Zhao, Xu and Jiang40), and in addition, to decrease holoenzymes of carboxylases(Reference Samols, Thornton and Murtif41, Reference Knowles42). Acetylation generally activated some enzymes in fatty acid oxidation(Reference Zhao, Xu and Jiang40). The physiologically active form of biotin is covalently attached at the active site of a class of important metabolic enzymes in gluconeogenesis, lipogenesis and amino acid metabolism(Reference Samols, Thornton and Murtif41, Reference Knowles42). Accordingly, decreased acetylation and biotin-dependent enzymes lead to reduced fatty acid oxidation and to save fat in the body.
Vitamin B6 concentrations, expected to be the last vitamin decreased in tissues by starvation(Reference Cherel, Robin and Maho3), decreased in the stomach, skeletal muscle and serum of the S2 rats. Vitamin B6 in the lung and serum decreased in the S6 rats. The differences in the pattern of vitamin B6 decline may be due to the fat content of each tissue. Nicotinamide concentrations in tissues and blood were unchanged by starvation, despite this vitamin being involved in energy metabolism. Since nicotinamide is biosynthesised from tryptophan(Reference Gholson, Ueda and Ogasawara43), nicotinamide concentrations in organs and blood were maintained. Urinary excretion of the sum of nicotinamide and its catabolites was high after 1 d of starvation and subsequently decreased. These results are in agreement with those reported by a previous study(Reference Fukuwatari, Yoshida and Takahashi30). The proportion of nicotinamide, MNA, 2-Py and 4-Py in urine is controlled by enzymes involved in the metabolism of tryptophan to niacin. Starvation or food restriction induces a decline in MNA oxidase activity(Reference Shibata, Kondo and Miki44) and an elevation in nicotinamide methyltransferase(Reference Shibata, Kondo and Miki44). This may explain why levels of 2-Py and 4-Py in urine decreased while those of MNA increased. MNA is an inhibitor of nicotinamide methyltransferase(Reference Aksoy, Szumlanski and Weinshilboum45). Therefore, an accumulation of MNA inhibits the activity of nicotinamide methyltransferase and leads to an increase in free form of nicotinamide, which inhibits the activities of histone deacetylase(Reference Gallo, Smith and Smith46) and poly(ADP-ribose) synthetase(Reference Banasik, Komura and Shimoyama47). This control might be suitable for living long during starvation.
To our knowledge, the present study presents the first data on vitamin status during the three phases of starvation. The changes in B-group vitamin concentrations in tissues and blood did not always correspond to metabolic states. The changes in vitamin content can be divided into three groups. First, vitamin B1, vitamin B2, nicotinamide and biotin levels declined gradually. Second, vitamin B6 and vitamin B12 levels rapidly decreased after 1 d of starvation and then remained at a steady level. Finally, pantothenic acid and folate initially decreased in the S1 rats, then returned to near basal levels in the next day of starvation, then subsequently decreased again. This might mean that pantothenic acid and folate were mobilised to other tissues. We are unsure why such complicated changes occur. It is clear that further investigation, such as separate measurement of the free forms of the vitamins and of coenzymes, into the changes in the vitamin requirements of starving rats would be useful for the prevention of vitamin deficiency during starvation or for consequent refeeding.
Acknowledgements
The present study was supported by a Research Grant for Comprehensive Research on Cardiovascular and Lifestyle Related Diseases from the Ministry of Health, Labour and Welfare of Japan. The title of the project was ‘Studies on Construction of Evidence to revise the Dietary Reference Intakes for Japanese – Elucidation of balance between macronutrient and micronutrient’ (Principal Investigator, K. S.). A. M. and T. F. were responsible for designing the study strategies, retrieving the references and writing the manuscript. M. S. participated in retrieving references and critical reading. K. S. directed and designed the study. The authors have no conflict of interests to report.