The term ‘transition dairy cow’ was introduced in the 1990s and refers to the period from approximately 3 weeks before calving to 3 weeks after calving( Reference Grummer 1 , Reference Drackley 2 ). This is a period of profound change in the metabolic and endocrine status of cows to prepare them first for parturition and then for lactation( Reference Grummer 1 ). The increase in demand for energy and protein for accelerated fetal growth can often be met despite the 30–35 % decline in DM intake (DMI) that typically occurs during the final week before calving( Reference Hayirli, Grummer and Nordheim 3 ). However, a combination of hormonal changes and reduction in appetite during the period of increased energy demand for milk production results in a negative energy balance (NEB) in the period immediately after calving. This leads to lipolysis and the mobilisation of adipose tissue reserves( Reference Cooke, Del Rio and Caraviello 4 , Reference Rossi, Righi and Romanelli 5 ). There is a 5- to 10-fold increase in circulating concentrations of NEFA and the accumulation of TAG in the liver( Reference Bobe, Young and Beitz 6 – Reference Grummer 8 ). Although the oxidative capacity of the liver increases by 20 % during the final 3 weeks before calving( Reference Drackley, Overton and Douglas 9 ), the influx of NEFA into the liver overwhelms hepatic oxidation and the capacity of the liver to secrete esterified fatty acids (FA) (or TAG) as VLDL. The result is that TAG accumulates and the cow is likely to experience ‘fatty liver’ (FL) syndrome( Reference Bobe, Young and Beitz 6 , Reference Herdt 10 ). The latter precipitates hepatic dysfunction. The failure of the liver to use the excessive amounts of NEFA as fuel leads to a ketotic state that is associated with increased production and secretion of ketone bodies, primarily β-hydroxybutyrate (β-HB) and acetoacetate, and to a lesser extent acetone( Reference Herdt 10 , Reference Goff 11 ). About 50–60 % of transition dairy cows experience moderate to severe FL and ketosis, and this remains a major challenge for production and the health and welfare of dairy cows( Reference Duffield 12 , Reference Erb and Grohn 13 ).
An increase in plasma NEFA contributes to oxidative stress and non-specific and uncontrolled inflammatory responses in transition dairy cows. Elevated NEFA is known to be a common link between metabolic disease (FL, ketosis) and infectious disease (mastitis, Salmonellosis, metritis) around the time of calving( Reference Goff 11 , Reference Sordillo and Raphael 14 ). A compromised immune system is often a feature of the transition dairy cow( Reference Suriyasathaporn, Daemen and Noordhuizen-Stassen 15 , Reference Wathes, Cheng and Chowdhury 16 ). Of particular importance is the negative impact of inflammatory responses on the antimicrobial activity of macrophages and neutrophils. When the functions of these leucocytes are compromised, pathogens are able to establish long-term disease( Reference Sordillo and Raphael 14 ). It was suggested that strategies that reduce lipid mobilisation may also reduce the influence of inflammatory responses on animal general performance in the weeks around calving( Reference Sordillo and Raphael 14 ).
Transition cows need to be well managed to avoid inflammatory infection and metabolic disease, trauma, digestive disorders, heat and oxidative stress, and dystocia( Reference Turk, Juretić and Gereš 17 ). Subclinical inflammation can have a detrimental effect on metabolic function, as reflected by the development of ketosis and FL( Reference Bertoni, Trevisi and Calamari 18 , Reference Ametaj, Bradford and Bobe 19 ). Acute-phase proteins (APP), which provide a measure of inflammation, are mainly synthesised in the liver in the days around calving. Pro-inflammatory cytokines produced at the same time by immune cells can disrupt the hepatic synthesis of negative APP such as APOB100, a key component of VLDL( Reference Fleck 20 , Reference Gruys, Toussaint and Landman 21 ). Supplementary feeding of rumen-protected choline (RPC) was shown to reduce the negative effects of hepatic lipidosis in transition cows by increasing the expression of FA transport protein 5 and carnitine transporter SLC22A5 in the liver and promote apo B-containing lipoprotein assembly( Reference Goselink, van Baal and Widjaja 22 ). The inclusion of choline in the transition diet can therefore be partially effective in attenuating the negative effects of inflammatory-like condition on hepatic function in transition cows.
The transition dairy cow has understandably been the focus of considerable research, and general reviews are available( Reference Sordillo and Raphael 14 , Reference Chagas, Bass and Blache 23 , Reference Bradford 24 ). The present article provides an overview of the functional and morphological changes in the liver of transition dairy cows, the response of transition dairy cows to the provision of choline and the ability of choline to alleviate FL and some of its consequences.
Metabolic function of the liver in transition dairy cows
As noted, the energy requirement in dairy cows increases at calving because of the initiation of lactation. Circulating concentrations of NEFA increase immediately after calving because of adipose tissue mobilisation to support lactation( Reference Emery, Liesman and Herdt 25 , Reference Reynolds, Aikman and Lupoli 26 ). Hepatic capacity to esterify imported NEFA to TAG increases at the time of parturition( Reference Grum, Drackley and Younker 27 , Reference Litherland, Dann and Drackley 28 ), and the concentrations of TAG in hepatic tissue are elevated by 1 d postpartum( Reference Adewuyi, Gruys and Van Eerdenburg 29 – Reference Skaar, Grummer and Dentine 32 ). Because the uptake of NEFA overwhelms the capacity for hepatic oxidation and secretion of TAG as VLDL (Fig. 1), FL syndrome is common in transition cows( Reference Bobe, Young and Beitz 6 ). Wensing et al. ( Reference Wensing, Kruip and Geelen 33 ) categorised FL by the percentage of TAG in the wet weight of the liver. Normally, TAG comprises <1 % of the wet weight of the liver, but it can increase to 1–5, 5–10 or >10 % in mild, moderate and severe FL, respectively( Reference Bobe, Young and Beitz 6 ). Excessive hepatic FA uptake and FL is often accompanied by partial FA oxidation with a consequent increase in production and release of ketone bodies into the general circulation( Reference Drackley, Overton and Douglas 9 , Reference Herdt 10 , Reference Duffield 12 ). Ruminants have an inherently low hepatic capacity to synthesise VLDL, and this could be partially attributed to the limited availability of dietary choline for incorporation into phosphatidylcholine for VLDL synthesis in the liver( Reference Grummer 31 ).

Fig. 1 Role of choline in the export of hepatic NEFA as TAG in form of VLDL. NEB, negative energy balance; CAC, citric acid cycle.
Molecular and morphological changes of the liver during fatty liver syndrome
The gross morphological and cellular features of the liver are altered in cows affected by FL. The liver becomes enlarged and swollen as a result of the accumulation of cytosolic lipid droplets in the parenchyma. The colour of the liver changes from dark red to pale yellow( Reference Bobe, Young and Beitz 6 , Reference Tharwat, Oikawa and Buczinski 34 , Reference Morrow, Hillman and Dade 35 ). The hepatocytes show a number of morphological changes that include the following: (1) enlargement; (2) mitochondrial damage; (3) compression and decreased volume of nuclei, which results in DNA migration from the core to the anode; (4) decreased volume of rough endoplasmic reticulum (ER); and (5) decreased number of organelles( Reference Tharwat, Endoh and Oikawa 36 – Reference Reid and Collins 38 ).
The prolonged exposure (36–51 h) of hepatocytes to NEFA in vitro reduced ureagenesis and gluconeogenesis( Reference Strang, Bertics and Grummer 39 ). Exposure of hepatic tissue to metabolic and inflammatory challenges such as high amounts of NEFA, lipopolysaccharides of microbial origin and TNF-α as one of the pro-inflammatory cytokines during the period of NEB can activate pathways contributing to hepatocyte apoptosis and ER stress( Reference Ringseis, Gessner and Eder 40 , Reference Gessner, Schlegel and Ringseis 41 ). Biochemical adaptation and symptoms caused by ER stress are similar to those associated with fatty liver, ketosis or inflammation( Reference Gessner, Schlegel and Ringseis 41 ). It was reported that thirteen out of fourteen genes of the ER stress-induced unfolded protein response in liver were up-regulated after parturition, and some remained up-regulated even at 14 weeks postpartum – when the cows were already in a positive energy balance( Reference Gessner, Schlegel and Ringseis 41 ). This suggested that the high metabolic demand during lactation could induce a certain degree of ER stress without the burden of high NEFA concentration or inflammation( Reference Gessner, Schlegel and Ringseis 41 ).
Changes in hepatocyte integrity and function leads to cell necrosis and cell leakage( Reference Bobe, Young and Beitz 6 ). Because of cellular damage, circulating concentrations of hepatic enzymes such as aspartate aminotransferase, γ-glutamyl transpeptidase, creatine kinase and lactate dehydrogenase are elevated in cows with FL, particularly in severe cases( Reference Bobe, Young and Beitz 6 ). Plasma concentrations of bile constituents (bilirubin, cholic acid and bile acids) increase, demonstrating that bile flow decreases in FL( Reference Bobe, Young and Beitz 6 ).
Alterations in immune function have been reflected by changes in the expression of hepatic serum amyloid A1 (SAA1), which is involved in neutrophil/macrophage chemotaxis. Expression of mRNA encoding pro-inflammatory cytokines and APP (TNF-α, SAA1) is positively correlated with lipid mobilisation from adipose tissue( Reference Drackley, Dann and Douglas 30 ). A similar tendency was observed in the expression of other hepatic genes affecting immune function (IL27RA, THF, IFNGR2)( Reference Drackley, Dann and Douglas 30 , Reference Loor, Dann and Everts 42 ). Inflammation also contributes to bovine FL and promotes liver TAG accumulation( Reference Bradford 24 ). It was suggested that inflammatory pathways may be responsible for decreased glucose production in cows with FL( Reference Bradford 24 ).
The expression of a network of genes that are involved in lipid, carbohydrate and N metabolism in hepatic tissue is variously altered in cows during peripartum( Reference Loor 43 ). The expression of genes contributing to FA transport (cluster of differentiation 36 (CD36) and acyl-CoA synthetase 1 (ACSL1)) increases in the first 10 d after calving( Reference Loor, Dann and Everts 42 , Reference Bionaz and Loor 44 , Reference Van Dorland, Richter and Morel 45 ). Similarly, the expression of genes involved in the esterification of hepatic FA (glycerol-3-phosphate acyl transferase, mitochondrial (GPAM), diacylglycerol O-acyltransferase, homology 1 (DGAT1) and glycerol-phosphate acyltransferase 1 (AGPAT 1)) is elevated in the first 10 d of lactation, contributing to FL development( Reference Drackley, Dann and Douglas 30 , Reference Loor, Dann and Everts 42 ). The expression of microsomal TAG transfer protein (MTTP), a factor promoting VLDL assembly in the ER, reaches the highest level in hepatic tissue around parturition( Reference Goselink, van Baal and Widjaja 22 , Reference Wetterau, Lin and Jamil 46 , Reference Loor, Dann and Guretzky 47 ). Expression of the APOB100 gene is down-regulated in dairy cows approaching parturition, which results in reduced synthesis of hepatic APOB100( Reference Bernabucci, Ronchi and Basiricò 48 ). In a recent study, no change was found in APOB100 in control cows, but the level was increased at week 6 of lactation in cows that received choline supplementation( Reference Goselink, van Baal and Widjaja 22 ). Cultured dairy cow hepatocytes showed decreased expression of MTTP, APOB100 and APOE when incubated with NEFA( Reference Li, Guan and Li 49 ). The latter suggested a direct role of mobilised FA in liver gene expression and the aetiology of FL.
Provision of choline in transition dairy cows
Choline is an important constituent of the cell membrane, found mostly in the form of the phospholipids phosphatidylcholine (PC) and lysophosphatidylcholine, which play a vital role in cellular structure and activity( Reference Lima, Filho and Greco 50 – Reference Santos and Lima 52 ). Choline is also required for the synthesis of the neurotransmitter acetylcholine, involved in the metabolism of FA in the liver and serves as a methyl donor. It is often incorrectly classified as a member of the vitamin B-complex. It does not qualify as a vitamin because (1) choline is required in greater amounts (g v. mg), (2) it does not serve as a cofactor in enzymatic reactions and (3) it can be synthesised endogenously( Reference Lima, Filho and Greco 50 , Reference Grummer 51 ). The bioavailability of choline from the diet is very low in ruminants (<30 %) because of extensive degradation by rumen micro-organisms( Reference Sharma and Erdman 53 ). However, choline can be synthesised in the liver from methionine; therefore, the level of methionine in the metabolic pool is an important factor in determining choline bioavailability in different metabolic pathways. Choline is a direct precursor to betaine in methyl metabolism( Reference Santos and Lima 52 ). As a methyl donor, choline is involved in transmethylation reactions that involve folic acid and vitamin B12 such as methionine and carnitine synthesis( Reference Lima, Filho and Greco 50 , Reference Bremer 54 ) and DNA methylation. PC synthesis is affected by methyl donors’ bioavailability, choline and methionine, in the metabolic pool. It has been suggested that PC has more contribution in VLDL synthesis in cows receiving rumen-protected methionine( Reference Osorio, Ji and Drackley 55 ). Thus, it is critical to ensure that sufficient amount of methionine is included in the diet. In addition, choline contributes to the cholinergic nervous system by contribution in acetylcholine synthesis. Henning et al. ( Reference Henning, Swendseid and Ivandic 56 ) revealed that feeding choline- and methionine-deficient diets leads to reduction of vitamins concentrations including ascorbic acid, α and γ-tocopherol and retinol, which could lead to epithelial infection, muscle degeneration, liver damage and reduced resistance to infection. The most biologically active form of vitamin E, α-tocopherol, requires hepatic VLDL to be transported via circulation for tissue utilisation, erythrocytes, adipose tissue, heart and skeletal muscles. Hence, choline has an indirect influence on general health, particularly immunity, in humans and animals( Reference Henning, Swendseid and Ivandic 56 , Reference Dutta-Roy, Gordon and Campbell 57 ).
Hormonal changes at calving and NEB during early lactation are associated with increased hepatic uptake of NEFA and a higher requirement of the liver to metabolise FA( Reference Grummer 51 ). In non-ruminants, the availability of PC is a major factor affecting the rate of VLDL export and susceptibility of the liver to accumulate TAG( Reference Cole, Vance and Vance 58 ). This is probably also true in ruminants and low bioavailability of dietary choline, and the greater requirement for VLDL export (Fig. 1) because of increased FA uptake makes the transition dairy cow particularly susceptible to FL.
Inclusion of RPC in the diet of feed-restricted dry cows or transition cows appears to facilitate TAG secretion from the liver( Reference Cooke, Del Rio and Caraviello 4 , Reference Goselink, van Baal and Widjaja 22 , Reference Zom, Van Baal and Goselink 59 , Reference Zahra, Duffield and Leslie 60 ). This suggests that choline should be considered as an important nutrient in transition dairy cows. However, there are insufficient dose–response data to make a clear case for choline requirement in transition dairy cows. Notwithstanding, the ability to reduce liver TAG has been commonly reported when supplementing with 15 g of RPC/d( Reference Zom, Van Baal and Goselink 59 , Reference Ardalan, Dehghan-Banadaky and Rezayazdi 61 – Reference Shahsavari 63 ). The effects of supplementary RPC on biochemical, reproductive and productive features of dairy cows are summarised in Tables 1, 2 and 3.
Table 1 The effect of rumen-protected choline (RPC) supplementation of dairy cows on NEFA and β-hydroxybutyrate (β-HB)

↑, Increased; ↓, decreased; −, no change.
* As choline chloride.
Table 2 The effect of rumen-protected choline supplementation of dairy cows on DM intake (DMI) and milk yield and composition
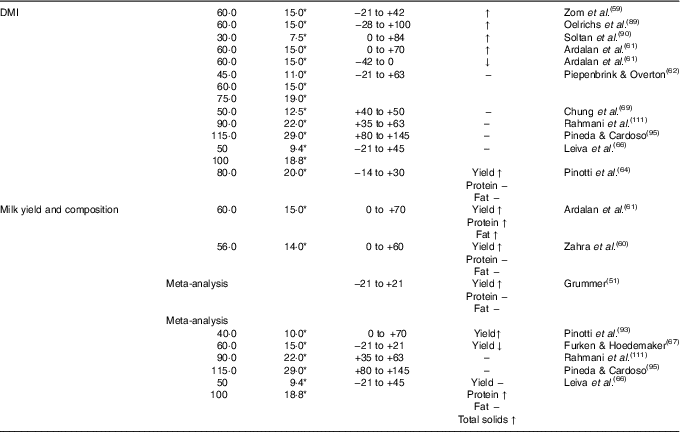
↑, Increased; ↓, decreased; −, no change.
* As choline chloride.
Table 3 The effect of rumen-protected choline supplementation of dairy cows on postpartum disorders, reproductive parameters and gene expression

↑, Increased; ↓, decreased; −, no change.
* As choline chloride.
Choline and blood metabolites
The response of blood metabolites in transition cows to RPC supplementation has been inconsistent. This could be because of (1) the period at which supplementation was started, (2) the duration of supplementation, (3) the level of supplementation or (4) the efficiency of protecting choline from rumen degradation. In some studies, RPC reduced blood NEFA( Reference Cooke, Del Rio and Caraviello 4 , Reference Pinotti, Baldi and Politis 64 , Reference Esposito, Irons and Webb 65 ), whereas no effect was observed in other studies( Reference Cooke, Del Rio and Caraviello 4 , Reference Piepenbrink and Overton 62 , Reference Shahsavari 63 , Reference Leiva, Cooke and Brandão 66 , Reference Furken and Hoedemaker 67 ). A reduction in plasma NEFA appears not to be because of reduced lipolysis, but rather attributed to a combination of (1) improved buffering and transport of intracellular NEFA, (2) improved excretion by VLDL transport and (3) improved carbohydrate metabolism( Reference Goselink, van Baal and Widjaja 22 ). Reduced blood concentrations of β-HB were observed in transition cows supplemented with RPC( Reference Shahsavari 63 , Reference Esposito, Irons and Webb 65 , Reference Furken and Hoedemaker 67 , Reference Elek, Gaál and Husvéth 68 ), but this response was not consistent in all studies( Reference Cooke, Del Rio and Caraviello 4 , Reference Zom, Van Baal and Goselink 59 , Reference Piepenbrink and Overton 62 , Reference Pinotti, Baldi and Politis 64 , Reference Leiva, Cooke and Brandão 66 , Reference Chung, Brown and Martinez 69 ). The positive response in some studies could be attributed to the positive contribution of choline in hepatic gene networks to facilitate VLDL synthesis and exportation( Reference Goselink, van Baal and Widjaja 22 ).
Choline and metabolic hormones
The somatotropic axis comprising growth hormone (GH), the GH receptor and insulin-like growth factor-I (IGF-I) has a central role in regulating nutrient partitioning at different stages of lactation( Reference Radcliff, McCormack and Crooker 70 ). NEB in transition cows disrupts this axis causing a shift from an anabolic state to a catabolic state. It was reported that at the time of parturition GH concentrations are increased, IGF-I concentrations are reduced and GH receptors are decreased by about 50 %( Reference Radcliff, McCormack and Crooker 70 – Reference Lucy 72 ). Metabolic pathways in the liver shift towards gluconeogenesis in preparation for high-glucose demand by the mammary gland( Reference Reynolds, Aikman and Lupoli 26 , Reference Lomander 73 – Reference Akers 75 ). It is likely that GH exerts a suppressive effect on insulin, leading to reduced synthesis and secretion of insulin by the pancreas( Reference Ponsart, Freret and Seegers 76 – Reference Humblot, Grimard and Freret 80 ). There is evidence that the effects of insulin in suppressing hepatic gluconeogenesis and promoting the synthesis of IGF-I are attenuated during early lactation( Reference Boisclair, Wesolowski and Kim 81 ). Cows maintained under severe NEB had reduced expression of hepatic IGF-I, GH receptors and IGF-binding proteins( Reference Fenwick, Fitzpatrick and Kenny 82 ). In a recent in vitro study, higher IGF-I positively affected the expression of hepatic genes involved in hepatic VLDL assembly and secretion in bovine hepatocytes, namely APOB100, MTTP and the LDL receptors( Reference Li, Guan and Li 49 ). The provision of 120 g of RPC/cow per d (30 g choline chloride/cow per d) to transition cows from 21 d before calving to 42 d after calving did not influence IGF-I concentration( Reference Shahsavari 63 ). A similar finding was observed when multiparous Holstein cows received 50 and 100 g of RPC/cow per d (9·4 and 18·8 g of choline chloride/cow per d, respectively) in the last 21 d of pregnancy and in the first 45 d of lactation( Reference Leiva, Cooke and Brandão 66 ). The latter study reported greater concentrations of insulin and haptoglobin in RPC-supplemented cows. A positive association between haptoglobin and insulin can partially explain the higher insulin concentration found in RPC-supplemented cows( Reference Steiger, Senn and Altreuther 83 , Reference Andersson, Flodström and Sandler 84 ). In contrast, plasma concentration of insulin did not show major differences in multiparous Holstein cows that received 120 g of RPC (30 g of choline chloride/cow per d) during the period between 3 weeks before and 3 weeks after calving( Reference Shahsavari 63 ).
Choline and DM intake
The decline in DMI during the last 3 weeks of pregnancy can reach 32 %, with 89 % of the decline occurring during the final week( Reference Hayirli, Grummer and Nordheim 3 ). There is evidence to suggest that a significant proportion of this decline can be avoided by feeding low-energy diets( Reference Janovick and Drackley 85 ). However, such diets can only be fed before lactation because of the lower demands for nutrients, particularly energy. Colostrum production immediately before calving initiates increasing energy demand, which rises more sharply in the weeks after calving following lactation( Reference Hayirli, Grummer and Nordheim 3 , Reference Grummer 31 , Reference Goff and Horst 86 , Reference Bell 87 ). Hormonal changes around calving noted above and NEB trigger lipolysis, leading to increased concentration of NEFA in circulation( Reference Vazquez-Anon, Bertics and Luck 88 ). Compared with the non-lactation period, the concentration of hepatic TAG can be elevated 3-fold by calving and can continue to increase thereafter( Reference Goff and Horst 86 ).
Zom et al. ( Reference Zom, Van Baal and Goselink 59 ) reported that supplying 60 g of RPC/cow per d (15 g of choline chloride) from week 3 before calving to week 6 after calving increased DMI from 14·4 to 16·0 kg/d and net energy intake from 98·2 to 109·1 MJ/d. This was in agreement with an earlier study in which feeding 60 g of RPC/cow per d (15 g of choline chloride) to lactating cows from day 28 prepartum to day 100 in milk increased DMI as a percentage of body weight over the entire study period( Reference Oelrichs, Lucy and Kerley 89 ). In another study, DMI was increased by 8·4 % in cows supplemented with 30 g of RPC/cow per d (7·5 g of choline chloride) in the first 12 weeks of lactation( Reference Soltan, Mujalliz and Mandour 90 ). Ardalan et al.( Reference Ardalan, Dehghan-Banadaky and Rezayazdi 61 ) reported that feeding 60 g of RPC/cow per d (15 g choline chloride) from the time of calving until week 10 of lactation increased DMI (22·1 v. 18·9 kg/d), whereas feeding the same amount of choline before parturition was not associated with increased intake. An interaction between body condition score (BCS) and DMI was reported by Zahra et al. ( Reference Zahra, Duffield and Leslie 60 ). Cows with BCS of≥4 (scale 1 to 5) at 3 weeks before calving ate 1·1 kg of DM/d more from week 3 before calving to week 4 after calving when supplemented with RPC. Cows with lower BCS did not show the same response. Other studies did not demonstrate an effect of RPC on postpartum DMI( Reference Zahra, Duffield and Leslie 60 , Reference Piepenbrink and Overton 62 , Reference Leiva, Cooke and Brandão 66 , Reference Chung, Brown and Martinez 69 ). A meta-analysis of thirteen studies showed that feeding RPC does not affect feed intake before calving but increases DMI by 0·8 kg/d in early lactation( Reference Grummer 51 ). Feeding RPC did not affect changes in body condition in transition cows, but this may have been because of increases in milk yield that accompanied increased feed intake( Reference Zom, Van Baal and Goselink 59 , Reference Elek, Newbold and Gaal 91 ). It can be concluded that choline can indirectly affect DMI in transition cows by restricting the detrimental effects of fatty liver syndrome (FLS) and ketosis on general health( Reference Esposito, Irons and Webb 65 ). Positive responses can be associated with fewer incidences of postpartum disorders such as mastitis and retained fetal membrane (RFM) in transition cows( Reference Esposito, Irons and Webb 65 , Reference Lima, Sa Filho and Greco 92 ).
Choline and milk yield
Pinotti et al. ( Reference Pinotti, Baldi and Politis 64 ) reported that RPC supplementation of Italian Holstein multiparous cows during the period from 14 d before calving to 30 d after calving at a rate of 20 g of choline chloride/cow per d increased milk yield without affecting the concentrations of fat or protein. Supplementing dairy cows fed alfalfa hay- and maize silage-based total mixed ration (TMR) with 60 g of RPC/d (providing 15 g choline chloride) during the first 10 weeks of lactation increased the 40g/kg fat-corrected milk (4 % FCM) yield by 3·28 kg/d and the yield of both fat and protein( Reference Ardalan, Dehghan-Banadaky and Rezayazdi 61 ). Multiparous Holstein cows that received 50 and 100 g of RPC/cow per d (9·4 and 18·8 g of choline chloride/cow per d) for 21 d before calving and 45 d after calving, respectively, had higher milk protein, total solids and fat concentration( Reference Leiva, Cooke and Brandão 66 ). In contrast, no treatment effect was observed on fat-corrected milk or on solid-corrected milk yield( Reference Leiva, Cooke and Brandão 66 ). Primiparous and multiparous Holsteins fed a maize silage-, haylage- and grass hay-based TMR produced more milk (1·2 kg/d) during the first 60 d of lactation when supplemented with 56 g of RPC/d (14 g of choline chloride)( Reference Zahra, Duffield and Leslie 60 ). Cows with BCS≥4 (scale 1–5) showed greater increases in milk yield than cows with lower BCS( Reference Zahra, Duffield and Leslie 60 ). The analysis of data from 11 studies revealed a positive milk yield response when RPC was supplemented at >40 g of RPC/d (>10 g of choline chloride)( Reference Pinotti, Polidori and Campagnoli 93 ). A meta-analysis of thirteen trials indicated that RPC supplementation during the transition period increased milk yield by an average of 3·9 kg/d( Reference Grummer 51 ); however, milk protein and fat percentage were not affected. Another meta-analysis that included studies beyond the transition period found that milk fat content was not influenced by RPC( Reference Sales, Homolka and Koukolová 94 ). It was suggested that the increase in protein yield is possibly because of the provision of methyl groups and methionine sparing( Reference Sales, Homolka and Koukolová 94 ). A recent study showed that inclusion of RPC in the diet of mid-late lactation dairy cows can alter the milk fat profile by increasing the proportion of n-3 content( Reference Pineda and Cardoso 95 ); however, milk yield, 3·5 % fat-corrected milk and energy-corrected milk were not affected. On the basis of the above overview, it can be speculated that dietary choline can directly and indirectly promote milk production in dairy cows. The direct effect may reflect a higher level of available methionine for milk synthesis and lower hepatic TAG, leading to enhanced gluconeogenesis in the liver( Reference Goselink, van Baal and Widjaja 22 ). The indirect effect is based on a positive production response due to general health improvement in transition cows.
Choline and postpartum disorders
FL and ketosis are common problems in high-yield dairy herds shifting from transition to lactation stage( Reference Herdt 10 , Reference Grummer 31 ). Feeding 60 g of RPC/cow per d (15 g of choline chloride) between 25 d before and 80 d after calving reduced the incidence of clinical ketosis and morbidity, and also reduced the incidence of mastitis( Reference Lima, Sa Filho and Greco 92 ). The occurrence of RFM was lower for cows that received 15 g of choline/d as RPC for the last 21 d of gestation, but cows fed RPC had an increased incidence of fever and tended to have more puerperal metritis( Reference Lima, Sa Filho and Greco 92 ). The positive responses could be attributed to fewer incidences of metabolic challenges around calving associated with general health improvement.
Choline and reproductive measures
As previously discussed, the disruption of metabolic homoeostasis in early lactation is associated with alterations in the profile of metabolic hormones and metabolites( Reference Bobe, Young and Beitz 6 , Reference Grummer 31 , Reference Lucy 72 ). These changes have negative consequences for ovarian follicular function, the resumption of ovulation and fertility in cows( Reference Bobe, Young and Beitz 6 , Reference Włodarek, Jaśkowski and Nowak 96 ). For example, IGF-I in concert with insulin has a critical role in ovarian responsiveness to follicle-stimulating hormone (FSH) and luteinising hormone (LH). IGF-I can act synergistically with FSH and LH to enhance ovarian development, steroidogenesis and embryonic development( Reference Radcliff, McCormack and Crooker 70 , Reference Lucy 97 ). Reduced plasma concentrations of IGF-I in early lactation can lead to decreased ovarian follicular growth and lower fertility( Reference Lucy 72 , Reference Zulu, Nakao and Sawamukai 98 ).
Variable reproductive responses to choline supplementation have been observed in transition cows. Oelrichs et al. ( Reference Oelrichs, Lucy and Kerley 89 ) reported that conception and pregnancy rates increased by feeding RPC during the transition period, whereas in another study there were no differences in conception rate, pregnancy loss and percentage of cows cycling( Reference Lima, Sa Filho and Greco 92 ). A recent study showed that feeding 60 g of RPC/cow per d (15 g of choline chloride) from 21 d prepartum to 21 d postpartum was associated with less endometritis, lower number of stillbirths and more cyclic cows in week 5 postpartum( Reference Furken and Hoedemaker 99 ). Feeding 240 g of RPC/cow per d (60 g of choline chloride) from parturition to day 42 in milk was associated with increased ovarian follicle growth and earlier resumption of ovulation( Reference Shahsavari 63 ). Feeding 30 g of choline chloride/cow per d (120 g of RPC) from day 21 before calving to day 42 after calving increased the proportion of pregnant cows by day 100 postpartum (28 v. 40 %) after the first artificial insemination on day 70 after calving( Reference Shahsavari 63 ). In the same study, the level of leptin was significantly higher in cows supplemented with RPC. This outcome can probably be attributed to the improvement of the link between metabolic homoeostasis and hypothalamic–pituitary–ovarian axis in transition cows. Leptin has an important role in this axis by binding to its specific receptors, kisspeptin, in the hypothalamus and consequently stimulating gonadotropin-releasing hormone release( Reference Delavaud, Ferlay and Faulconnier 100 , Reference Brann, Wade and Dhandapani 101 ). In addition, leptin receptors have been detected in the ovaries of cattle( Reference Spicer 102 ), and leptin has positive effects on oocyte quality and embryo development in cattle( Reference Clempson, Pollott and Brickell 103 ).
Choline and gene expression
RPC increased the expression of FA transport protein 5 (FATP5) and carnitine transporter SLC22A5 in hepatic tissue( Reference Goselink, van Baal and Widjaja 22 ). These proteins are involved in FA uptake and intercellular transport in the liver( Reference Goselink, van Baal and Widjaja 22 ). The response to RPC might be attributable to the role of choline in carnitine synthesis by the provision of methyl groups( Reference Bremer 54 ) or by improving hepatic intake of carnitine( Reference Carter and Frenkel 104 ). Increasing carnitine availability in hepatocytes can reduce TAG accumulation in transition cows by stimulating FA oxidation( Reference Schlegel, Keller and Hirche 105 , Reference Carlson, McFadden and D’Angelo 106 ). Similar results were obtained when feed intake in mid-lactating cows was restricted to induce a NEB( Reference Akbar, Bionaz and Carlson 107 ). Abomasal infusion of l-carnitine tended to prevent or decrease TAG accumulation but had no apparent effect on the expression of genes involved in gluconeogenesis (pyruvate carboxykinase, pyruvate carboxylase and pyruvate dehydrogenase kinase, isozyme 4, PDK4), inflammation (serum amyloid A3; SAA3) and signalling (adiponectin receptor 2; ADIPOR2). It was concluded that DMI restriction, but not the infusion of l-carnitine, influenced expression of the above genes( Reference Akbar, Bionaz and Carlson 107 ). It could be suggested that carnitine does not directly affect the expression of genes associated with FA oxidation.
Feeding RPC to transition cows increased expression of the PPARα/δ gene, which is involved in regulatory loops of FA oxidation and transport( Reference Goselink, van Baal and Widjaja 22 , Reference Loor, Everts and Bionaz 108 ). Feeding RPC also increased mRNA for GLUT2, a hepatic protein that facilitates glucose release from the liver into blood( Reference Zhao and Keating 109 ). This would improve carbohydrate metabolism in the liver.
In a nutrition-induced ketosis study, the expression of glucogenic genes pyruvate carboxylase and fructose 1,6-bisphosphatase 1 was up-regulated, whereas phosphoenolpyruvate carboxykinase 1, which is also an important glucogenic gene, was down-regulated( Reference Loor, Everts and Bionaz 108 ). The level of pyruvate carboxylase mRNA was positively correlated with total fat in the liver and plasma concentrations of NEFA and β-HB( Reference Hammon, Stürmer and Schneider 110 ). An increase in the expression of pyruvate carboxylase and PDK4 was also reported in mid-lactating cows subjected to NEB( Reference Akbar, Bionaz and Carlson 107 ). The increase in the expression of pyruvate carboxylase and PDK4 indicates a hepatic response to increased demand for glucose for the lactating mammary gland during NEB. The increase in expression of the pyruvate carboxylase gene was reduced in cows receiving RPC before and after calving, suggesting that RPC improves energy status and carbohydrate metabolism in the liver and reduces the need for pyruvate carboxylase( Reference Goselink, van Baal and Widjaja 22 ). RPC supplementation during the transition period affected the expression of two key genes involved in VLDL synthesis (MTTP and APOB100)( Reference Goselink, van Baal and Widjaja 22 ). MTTP promotes VLDL synthesis in the ER( Reference Wetterau, Lin and Jamil 46 ) and reduces the accumulation of TAG in hepatic tissue. As mentioned earlier, APOB100 is a key protein required for VLDL synthesis( Reference Bernabucci, Ronchi and Basiricò 48 ). RPC had no effect on mRNA abundance for carnitine palmitoyl transferase 1A( Reference Goselink, van Baal and Widjaja 22 ). This suggested that the transport of long-chain FA into mitochondria is not affected by the provision of choline in transition cows( Reference Loor, Dann and Everts 42 , Reference Van Dorland, Richter and Morel 45 ).
Conclusion
Choline is an important nutrient and vital for the neurotransmitter acetylcholine, cell membrane structure and function, and hepatic VLDL synthesis. Lactating cows experiencing NEB during early lactation develop moderate to severe FLS because of an inability of the liver to cope with a high influx of NEFA. Under these conditions, supplementary choline can complement dietary choline to meet the increasing demand for the synthesis of PC, involved in the export of TAG from the liver. Given the extensive ruminal degradation of natural dietary choline, and the tremendous influx of FA into the liver during the transition period, it is reasonable to assume that choline is deficient in transition dairy cows. In support of this assumption, supplementation with RPC reduces TAG accumulation in the liver in transition dairy cows. The benefits of RPC supplementation in transition cows have been observed at the animal level (increased milk yield), organ level (reduce hepatic TAG) and cellular level (increased expression of MTTP and ApoB100 mRNA). Further research is required to determine optimal dietary levels of RPC for transition dairy cows and to elucidate the mechanism(s) of action of choline more precisely.
Acknowledgements
The authors gratefully acknowledge support from the Nancy Roma Paech Bequest to Professor Michael J. D’Occhio for the endowed chair at The Sydney of University. Arash Shahsavari received postgraduate financial support from The University of Queensland (position number 3022886).
All authors contributed to the literature search, manuscript writing and revisions of the article.
The authors declare that there are no conflicts of interest.