Zeolites are important crystalline micro- and meso-porous materials (Schwanke et al., Reference Schwanke, Balzer, Pergher, Martínez, Kharissova and Kharisov2017). They represent a group of naturally occurring hydrous aluminosilicate minerals with a cage-like crystal structure that can be synthesized in the laboratory (Altare et al., Reference Altare, Bowman, Katz, Kinney and Sullivan2007). The structural properties of zeolites make them unique among inorganic materials. They have many applications in industry due to their high porosity, chemical stability, specific active sites, ion-exchange capacity and large specific surface area (Gottardi & Galli, Reference Gottardi and Galli1985). Despite the abundance and low cost of natural zeolites, they have been less preferred than expensive synthetic zeolites because of their variable physical–chemical properties and impure mineralogical compositions. Because the cost of these synthetic porous materials is significant, research has focused on studies that deal with the modification of natural zeolites for various potential applications. Applications in catalysis and adsorption generally require an additional modification of the zeolite, such as dealumination or ion exchange (Tsitsishvili & Andronikashvili, Reference Tsitsishvili and Andronikashvili1992).
Zeolites possess a net negative charge due to the isomorphic substitutions of Si4+ by Al3+ in the tetrahedral sites. This residual negative charge is balanced by the presence of exchangeable cations (Na+, K+, Ca2+ and Mg2+) in the cages, which allows excellent performance in terms of thermal stability, acid resistance, etc., depending on the cationic composition (Tsitsishvili & Andronikashvili, Reference Tsitsishvili and Andronikashvili1992; Bertetti & Pabalan, Reference Bertetti and Pabalan2001). Due to their variable physicochemical properties, zeolites have been exploited in large-scale industrial applications such as adsorption/separation, ion exchange, molecular sieving and catalysis (Gottardi & Galli, Reference Gottardi and Galli1985). In addition to these conventional uses, which have a significant economic impact on various sectors of industry, there are specific uses of zeolites in new emerging applications, including luminescence, electricity, magnetism, microelectronics and biomedical processes. Recent studies have revealed that cation-exchange modification of natural zeolites with transition metals such as silver, zinc, copper, etc., also provides interesting pharmaceutical properties (Hotta et al., Reference Hotta, Nakajima, Yamamoto and Aono1998; Rivera-Garza, Reference Rivera-Garza, Olguin, Garcia-Sosa, Alcántara and Rodriguez-Fuentes2000; Top & Ulku, Reference Top and Ulku2004; Akhigbe et al., Reference Akhigbe, Ouki, Saroj and Lim2014; Demirci et al., Reference Demirci, Ustaoglu, Yılmazer, Sahin and Bac2014; Milenkovic et al., Reference Milenkovic, Hrenovic, Matijasevic, Niksic and Rajic2017; Youssefa et al., Reference Youssefa, El-Naggarb, Foudac and Youssef2019; Dutta & Wang, Reference Dutta and Wang2019; Hubner et al., Reference Hubner, Donati, Quines, Tessaro and Marcilio2020).
The use of silver, which has antimicrobial properties in the form of AgNO3, dates back to ancient times. Today, the unique antibacterial activity of silver means that it is used in various applications, such as common consumer products and even in complicated medical devices. However, AgNO3 is not suitable for direct usage. Recently, research has been carried out on supporting materials for silver ions, which, in turn, will form stable structures that are resistant to atmospheric oxidation and have long-lasting antibacterial properties. Zeolites have been considered as potential candidates for delivering the properties above as cation carriers, and their porous structure allows for slow Ag release (Tosheva et al., Reference Tosheva, Belkhair, Gackowski, Malic, Al-Shanti and Verran2017; Dutta & Wang, Reference Dutta and Wang2019). The Ag-exchanged zeolite exhibits greater bactericidal activity compared to zeolites containing other transition metals such as Cu+ and Zn2+ (Top & Ulku, Reference Top and Ulku2004).
Modification of porous materials similar to zeolites with metal ions is also known and has many applications (Zhao et al., Reference Zhao, Zhou and Liu2007; Wdowin et al., Reference Wdowin, Wiatros-Motyka, Panek, Stevens, Franus and Snape2014; Čapková et al., Reference Čapková, Matoušek, Rejnek, Bendlová, Pavlík and Kormunda2016; Cisneros et al., Reference Cisneros, Gao and Corma2019; Kennedy et al., Reference Kennedy, Khanafer and Tezel2019). Most of these modifications are performed at high pressure and/or temperature and with the addition of hazardous reagents, which raise their cost and may also be harmful to the environment. The high-temperature conditions and long-lasting reduction processes often result in the aggregation of metal ions, reducing their efficiency. Recently, plasma technology, which provides energy-source savings, uses limited amounts of water and is environmentally safe, has attracted attention because of its advantages in comparison to traditional modification methods; this is important for the prevention of pollution.
Previous studies have focused on the use of various plasma applications in the surface modification of clays and zeolites (Djowe et al., Reference Djowe, Laminsi, Njopwouo, Acayanka and Gaigneaux2013; Yavuz & Saka, Reference Yavuz and Saka2013; Şahin et al., Reference Şahin, Kaya and Saka2015; Valesco-Maldonado et al., Reference Velasco-Maldonado, Harnandez-Montoya, Montes-Moran, Rangel Vasquez and Perez-Cruz2018; Wahono et al., Reference Wahono, Suwanto, Prasetyo, Hernawan and Jatmiko2019). On the other hand, there are few published studies on metal ion-loading onto porous materials such as zeolites that reduce these ions to metal atoms or clusters/nanoparticles using various plasma techniques. Rodríguez-Méndez et al. (Reference Rodríguez-Mendez, Lopez-Callejas, Olguín, Valencia-Alvarado, Mercado-Cabrera, Pena-Eguiluz and Munoz-Castro2017) used pulsed dielectric barrier discharge (DBD) as a simple and economical way to produce silver nano- and micro-particles from Ag-zeolite. Osonio & Vasquez (Reference Osonio and Vasquez2018) proposed 13.56 MHz radiofrequency plasma as an efficient method in the reduction of silver ions to sodium zeolite samples. As the plasma contains electrons, positive and negative ions, reactive radicals and excited and neutral species, it might lead to a rapid reduction of metal ions (Liu et al., Reference Liu, Zhao, Li, Zhang, Chang and Bu2014). The ion-exchange method is efficient at incorporating Ag+ cations within the cavities of zeolites, and plasma treatment may tune surface characteristics such as roughness and wettability for biomedical applications (Taaca et al., Reference Taaca, Nakajima, Thumanu, Janphuang, Chanlek and Vasquez2020). Among the desirable surface properties are roughness and high wettability, which improve the bimolecular affinity to polar molecules (Taaca et al., Reference Taaca, Nakajima, Thumanu, Janphuang, Chanlek and Vasquez2020). Plasma technology is green and includes fast and simple methodologies for the preparation of metal nanoparticles without the need for any chemical reagents (Valesco-Maldonado et al., Reference Velasco-Maldonado, Harnandez-Montoya, Montes-Moran, Rangel Vasquez and Perez-Cruz2018; Osonio & Vasquez, Reference Osonio and Vasquez2018; Di et al., Reference Di, Li, Zhang, Wang and Fan2019).
Based on the relative energies of the electrons, ions, radicals and neutral and excited species, the plasmas used for modification can be classified as thermal plasmas and non-thermal plasmas (or cold plasmas). In the non-thermal plasmas, the energy of electrons is greater than that of heavy particles. Therefore, they may promote chemical reactions at a lower temperature. Recently, non-thermal atmospheric-pressure plasma jets (APPJs) have been applied in various industrial processes, the surface modification of polymers, molecular modification, sterilization and biomedical devices (Araya et al., Reference Araya, Yuji, Watanabe, Kashihara and Sumida2007; Junkar et al., Reference Junkar, Cvelbar and Lehocky2011; Mozetic & Vratnica, Reference Mozetic and Vratnica2011; Kostov et al., Reference Kostov, Nishime, Castro, Toth and Hein2014; Liu et al., Reference Liu, Zhao, Li, Zhang, Chang and Bu2014; Tanışlı et al., Reference Tanışlı, Mertadam, Poyraz, Şahin and Demir2016, Reference Tanışlı, Taşal, Şahin and Arslan2017, Reference Tanışlı, Taşal, Şahin and Dikmen2018). Remarkably, the use of atmospheric-pressure plasma systems in clays and zeolites is a new approach. In these systems, it is not necessary to control the pressure of the surrounding gas atmosphere without vacuum equipment. Therefore, they are quite easy to construct.
Dielectric-free electrodes, DBD, DBD-like and single-electrode plasma jets are well-known APPJs. The advantage of DBD systems is that there is no risk of arcing between the object and the dielectrics, regardless of the closeness to the end of the discharge tube. Depending on whether the material applied is conductive or not, there is a risk of arcing in applications using DBD-like plasma jet systems. However, in these systems, more power can easily be given to the plasma. If the arcing is prevented, their use carries many advantages (Lu et al., Reference Lu, Laroussi and Puech2012). DBD-like plasma jets have been applied to samples using various gases such as helium (He), argon (Ar), oxygen (O2) and gas mixtures (Junkar et al., Reference Junkar, Cvelbar and Lehocky2011; Lu et al., Reference Lu, Laroussi and Puech2012; Kawasaki et al., Reference Kawasaki, Hoketsu, Morisaki, Sato, Baba and Umeda2013; Chu & Lu, Reference Chu and Lu2014; Lu et al., Reference Lu, Reuter, Laroussi and Liu2019). Compared with other gases, argon ionizes easily, and therefore low levels of energy are generally sufficient to create argon plasma. Therefore, it is easier to generate non-thermal plasmas at atmospheric pressure with argon than with other gases.
This study attempts to build on the work of previous screening studies of this type (Djowe et al., Reference Djowe, Laminsi, Njopwouo, Acayanka and Gaigneaux2013; Yavuz & Saka, Reference Yavuz and Saka2013; Şahin et al., Reference Şahin, Kaya and Saka2015; Čapková et al., Reference Čapková, Matoušek, Rejnek, Bendlová, Pavlík and Kormunda2016; Fouodjouo et al., Reference Fouodjouo, Fotouo-Nkaffo, Lamins, Cassini, de Brito-Benetoli and Debacher2017; Taaca & Vasquez, Reference Taaca and Vasquez2017; Osonio & Vasquez, Reference Osonio and Vasquez2018; Valesco-Maldonado, Reference Velasco-Maldonado, Harnandez-Montoya, Montes-Moran, Rangel Vasquez and Perez-Cruz2018; Di et al., Reference Di, Li, Zhang, Wang and Fan2019; Wahono et al., Reference Wahono, Suwanto, Prasetyo, Hernawan and Jatmiko2019; Taaca et al., Reference Taaca, Nakajima, Thumanu, Janphuang, Chanlek and Vasquez2020) and focuses on understanding the influence of DBD-like Ar plasma jets on the properties of Ag-exchanged clinoptilolite using structural and spectroscopic characterization. The Ar plasma may reduce the Ag+ metal ions present in the pores and channels of Ag-clinoptilolite, as was further confirmed by ultraviolet–visible diffuse reflectance spectroscopy (UV–Vis DRS). The Ar plasma was applied to the surfaces of pellet samples prepared with Ag-clinoptilolite powder.
Materials and methods
Materials
A natural zeolitic tuff sample from a sedimentary deposit in the Gördes region (western Anatolia, Turkey) was supplied by Rota Mining Corporation. After grinding and screening, the <63 μm fraction was separated for this study.
In a previous study (Dikmen & Yorukogullari, Reference Dikmen and Yorukogullari2011), the main mineral constituent was estimated by semi-quantitative X-ray diffraction (XRD) analysis as clinoptilolite, an intermediate type of the isomorphous series heulandite–clinoptilolite end-members (Gottardi & Galli, Reference Gottardi and Galli1985). The physical properties of the natural clinoptilolite are listed in Table 1. The SiO2/Al2O3 ratio and oxide percentages (Table 2) were determined by X-ray fluorescence spectroscopy (Rigaku ZSX Primus). The Si/Al ratio of the sample is ~5.5, which is within the range 4.0–5.5, typical for clinoptilolite (Tsitsishvili & Andronikashvili, Reference Tsitsishvili and Andronikashvili1992).
Table 1. Mineralogical composition and some physical properties of natural clinoptilolite (after Dikmen & Yorukogullari, Reference Dikmen and Yorukogullari2011).
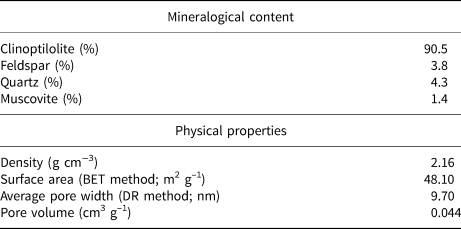
BET = Brunauer–Emmett–Teller; DR = Dubinin–Radushkevich.
Table 2. Chemical composition of natural and Ag-clinoptilolite.

LOI = loss on ignition.
Silver nitrate (AgNO3; 99.0% purity, locally sourced) was used to prepare Ag-clinoptilolite. HCl (Sigma-Aldrich) and NaCl (Carlo Erba) were of analytical grade. Deionized water was obtained from a Milli-Q purification system.
Experimental
Preparation of Ag-clinoptilolite
The Ag-exchanged form of clinoptilolite was prepared by the ion-exchange method according to Osonio & Vasquez (Reference Osonio and Vasquez2018). First, the clinoptilolite samples were mixed in 1 M HCl solution with a magnetic stirrer for 24 h at room temperature (25 ± 1°C) at a solid-to-liquid ratio of 1:100, washed with deionized water until Cl–-free (AgNO3 test) and dried overnight at 100°C. After drying, the clinoptilolite was stirred in 0.1 M NaCl solution at room temperature for 24 h and washed repeatedly with deionized water until Cl–-free (AgNO3 test). Subsequently, the Na-clinoptilolite was dried overnight at 100°C and was dispersed in 0.1 M AgNO3 solution to obtain Ag-clinoptilolite by Ag+-for-Na+ exchange. The ion-exchange process was performed with a solid-to-liquid phase ratio of 1:100 at room temperature for 24 h by mechanical stirring in darkness to avoid reduction of Ag+ to Ag0. Subsequently, the Ag-clinoptilolite was washed with deionized water until free of salts, dried at 50°C overnight and stored in darkness. The Ag-clinoptilolite powder was pressed into a 13 mm-diameter, 1 mm-thick pellet by applying 5 t of pressure using a uniaxial hydraulic press. The pellets were kept in a dark vial at room temperature for plasma treatment.
Plasma jet treatment of Ag-clinoptilolite
A plasma jet generated by a DBD-like system was used for the plasma treatments. The DBD-like plasma jet was produced in a horizontal quartz tube using a high-voltage AC power supply (Fig. 1). The length and outer and inner diameters of the quartz tube were 15, 0.52 and 0.41 cm, respectively. The quartz tube contains the 17.0 cm-long electrical tungsten electrode and the grounded electrode made of disc-shaped aluminium with a diameter of 3.6 cm and a thickness of 0.42 cm. Argon gas was sent through the quartz tube to obtain plasma. The Ar gas flow rate was fixed at 4 L min–1 and was controlled with an M + W Instruments GmbH Mass-Stream D6300 series flowmeter. The applied voltage and frequency of the power supply were 10 kV and 20 kHz, respectively.

Fig. 1. (a) Experimental setup and (b) image of a DBD-like plasma jet.
Material characterization
After completion of the plasma treatment for 30 and 60 min, the morphological, composition and structural changes of the samples were analysed using various techniques. The reduction of Ag+ to Ag0 after exposure to the Ar plasma was monitored via colour change in the pellets, because the shade of the pellets may be associated with the reduced Ag metallic forms. Fine powder samples were taken using a spatula from the dark grey shaded areas of the pellets for all analyses.
The UV–Vis DRS spectra were recorded on an UV–Vis Shimadzu 2600 spectrophotometer with a diffuse reflectance attachment. The background spectrum was recorded at room temperature with a blank glass plate and then the samples were placed onto the glass to record the UV–Vis DRS spectra over the range of 220–400 nm.
The Fourier-transform infrared (FTIR) spectra were recorded on a Perkin Elmer 100 FTIR spectrometer in the range 4000–400 cm–1, with a resolution of 4 cm–1 over an average of 100 scans. Approximately 1.5 mg samples were diluted in analytical-grade KBr (~200 mg) and pressed into discs before analysis. The XRD traces were obtained with a BRUKER D8 Advance diffractometer using Cu-Kα radiation (λ = 1.54059 Å) in the 7.0–70.0°2θ range, with a scanning step of 0.02° and a counting time of 4 s per step. The Cu-tube was operated at 40 kV and 40 mA. The changes in microstructure and the formation of Ag clusters were observed with a ZEISS Ultra Plus field emission scanning electron microscope (FE-SEM) after the plasma treatment.
Results and discussion
The chemical compositions of the Ag-clinoptilolite and natural clinoptilolite are shown in Table 2. The natural clinoptilolite contains Ca2+, Mg2+ and K+ as major extra-framework cations. In the structure, pores form negatively charged channels and cavities due to the imbalance caused by the substitution of Si4+ by Al3+. This negative charge is compensated by alkali or alkaline earth extra-framework cations (i.e. Na+, K+, Ca2+; Gottardi & Galli, Reference Gottardi and Galli1985; Petrov, Reference Petrov, Ming and Mumpton1995; Hubner et al., Reference Hubner, Donati, Quines, Tessaro and Marcilio2020). Following the Ag+ exchange, a significant portion of the original exchangeable cations in the natural clinoptilolite were replaced by Ag+ (Table 2), in agreement with previous work (Lihareva et al., Reference Lihareva, Dimova, Petrov and Tsvetanova2010; Unaldı et al., Reference Unaldı, Mızrak and Kadir2013; Kennedy et al., Reference Kennedy, Khanafer and Tezel2019). In addition, a small amount of Fe3+ was observed in both samples and did not affect Ag+ exchange. In addition, the Si/Al ratio of the Ag-clinoptilolite was greater than the Si/Al ratio of the original clinoptilolite, demonstrating that extraction of aluminium occurred during purification with HCl (Table 2).
The changes in the physical appearance of the pellets after plasma treatment for 30 and 60 min are shown in Fig. 2. The original colour of the pellet was close to white, and this turned to shades of dark grey after plasma exposure in accordance with previous studies on the fabrication of silver nanocomposites via plasma-assisted reduction or other synthesis methods (Shameli et al., Reference Shameli, Ahmad, Yunus, Rustaiyan, Ibrahim, Zargar and Abdollahi2010; Osonio & Vasquez, Reference Osonio and Vasquez2018).

Fig. 2. Photograph of an untreated Ag-clinoptilolite pellet compared to the Ag-clinoptilolite pellets treated with plasma for 30 and 60 min.
The UV–Vis DRS spectra for Ag-clinoptilolite subjected to various plasma treatment times and the spectrum of the untreated Ag-clinoptilolite are shown in Fig. 3. The spectrum of Ag-clinoptilolite shows a peak at 303 nm, in agreement with previous studies. The UV-Vis DRS spectra of zeolitic-rich tuffs containing primarily clinoptilolite and its silver-modified forms are used to determine the states of silver in the extra-framework sites (Concepción-Rosabal et al., Reference Concepción-Rosabal, Rodriguez-Fuentes, Bogdanchikova, Boschc, Avalosb and Lara2005; Rodríguez-Iznaga et al., Reference Rodríguez-Iznaga, Petranovskii, Castillón-Barraza and Concepción-Rosabal2011; Chmielewská et al., Reference Chmielewská2014; Suligoj et al., Reference Suligoj, Pavlovic, Arcon, Rajic and Tusar2020). After the DBD-like plasma jet application, new absorption peaks centred at 325 and 370 nm appeared. The peak centred at 370 nm showed a cluster of metallic Ag on the external surface of the clinoptilolite framework. The peak at 325 nm is related to Ag clusters, as shown in previous work. Hence, eight-atom Ag clusters in mordenite absorb at 295 and 325 nm (Bogdanchikova et al., Reference Bogdanchikovaa, Petranovskiia, Machorro, Sugic, Soto and Fuentes1999), while the UV–Vis DRS spectra of Ag-clinoptilolite reduced to Ag clusters at various temperatures showed absorbance peaks at 325 and 290 nm, related to Ag0 and Ag+ clusters, respectively (Concepción-Rosabal et al., Reference Concepción-Rosabal, Rodriguez-Fuentes, Bogdanchikova, Boschc, Avalosb and Lara2005). Silver clusters with <10 atoms related to these peaks are larger than the diameter of clinoptilolite channels (Chmielewská et al., Reference Chmielewská, Jesenák and Gáplovská2003). Thus, it is suggested that the DBD-like plasma jet application yields small Ag clusters, in agreement with previous work (Chmielewská et al., Reference Chmielewská, Jesenák and Gáplovská2003; Zaarour et al., Reference Zaarour, El Roz, Dong, Retoux and Aad2014; Skіba & Vorobyova, Reference Skіba and Vorobyova2018; Gonçalves et al., Reference Gonçalves, Barauna, Cunha-Filho, Chiavone-Filho, Vitoriano, Júnior and Mota-Lima2019; Taaca et al., Reference Taaca, Nakajima, Thumanu, Janphuang, Chanlek and Vasquez2020).

Fig. 3. UV–Vis DRS absorption spectra of (a) untreated plasma Ag-clinoptilolite, (b) Ag-clinoptilolite treated with plasma for 30 min and (c) Ag-clinoptilolite treated with plasma for 60 min. Cln = clinoptilolite.
Recently, a mechanism related to the plasma reduction of metal ions introduced into porous structures has been proposed, which suggests a direct combination of the electrons generated in the plasma and the ions of the salt (i.e. AgNO3). In this stage, the metal ions perform as ‘electron sinks’ that capture electrons upon exposure to the plasma. The neutral atom (Ag0) forms relatively stable Ag clusters with other Ag+ ions. Then, the Ag clusters combine with electrons to form Ag nanoparticles. Another mechanism suggests a plasma-induced dissociation of water molecules physisorbed on the zeolitic structure, which generates hydrated electrons and hydrogen radicals that easily react with the metal ions in the structure (Chmielewská et al., Reference Chmielewská, Jesenák and Gáplovská2003; Osonio & Vasquez, Reference Osonio and Vasquez2018; Gonçalves et al., Reference Gonçalves, Barauna, Cunha-Filho, Chiavone-Filho, Vitoriano, Júnior and Mota-Lima2019).
The FTIR spectra of the untreated and plasma-treated Ag-exchanged clinoptilolite were compared with that of the original clinoptilolite (Fig. 4a–d). The spectrum of the natural clinoptilolite sample is in accord with the clinoptilolite FTIR spectra reported in the literature (Breck, Reference Breck1974; Pechar & Rykl, Reference Pechar and Rykl1981; Tsitsishvili & Andronikashvili, Reference Tsitsishvili and Andronikashvili1992; Franus & Dudek, Reference Franus and Dudek1999; Mozgawa, Reference Mozgawa2000; Auerbach et al., Reference Auerbach, Carrado and Dutta2003; Korkuna et al., Reference Korkuna, Leboda, Skubiszewska-Zieba, Vrublevska, Gunko and Ryczkowski2006; Doula, Reference Doula2007; Elaiopoulos et al., Reference Elaiopoulos, Perraki and Grigoropoulou2010; Unaldı et al., Reference Unaldı, Mızrak and Kadir2013; Nikita & Chervonny, Reference Nikita and Chervonny2016; Valesco-Maldonado et al., Reference Velasco-Maldonado, Harnandez-Montoya, Montes-Moran, Rangel Vasquez and Perez-Cruz2018). The broad bands at 3640 and 3450 cm–1 are attributed to asymmetric and symmetric O–H stretching and the band at 1635 cm–1 is attributed to O–H bending of physisorbed water. The prominent band centred at ~1060 cm–1 corresponds to asymmetric T–O stretching vibrations (T = tetrahedral species Al, Si). The intense band in the 1040–1070 cm–1 region is due to O–Si(Al)–O bond vibrations in tetrahedra (TO4) or aluminium- and silicon-oxygen bridges (Pechar & Rykl, Reference Pechar and Rykl1981). More specifically, an idealized clinoptilolite sample would give a band at 1060 cm–1 (Mozgawa, Reference Mozgawa2000; Nikita & Chervonny, Reference Nikita and Chervonny2016). This band is associated with the Al and Si content in the crystalline framework of natural zeolites, and its position varies with the Si/Al ratio. More specifically, an increasing number of tetrahedral Al atoms (i.e. a lower Si/Al ratio) might shift the vibration mode to a lower frequency (Breck, Reference Breck1974; Pechar & Rykl, Reference Pechar and Rykl1981; Rodriguez-Fuentes et al., Reference Rodriguez-Fuentes, Ruiz-Salvador, Mir, Picazo, Quintana and Delgado1998; Elaiopoulos et al., Reference Elaiopoulos, Perraki and Grigoropoulou2008). The bands at 790 and 465 cm–1 are assigned to the external symmetric O–T–O stretching modes and the bending of the bonds inside the TO4 units, respectively (Rodriguez-Fuentes et al., Reference Rodriguez-Fuentes, Ruiz-Salvador, Mir, Picazo, Quintana and Delgado1998; Doula, Reference Doula2007). In the region of 700–500 cm–1, the bands at 667 and 600 cm–1 are due to four- or six-membered ring vibrations of TO4 units and stretching of the inter tetrahedral bonds typical of the ordered crystal structure, respectively. The very weak band at 525 cm−1 is attributed to ‘pore opening’ (Mozgawa, Reference Mozgawa2000; Doula, Reference Doula2007).

Fig. 4. FTIR spectra of (a) clinoptilolite, (b) untreated plasma Ag-clinoptilolite, (c) Ag-clinoptilolite treated with plasma for 30 min and (d) Ag-clinoptilolite treated with plasma for 60 min.
After the Ag+-exchange process, the FTIR bands occur at similar wavenumbers (Fig. 4b). The Ag+ cations did not affect significantly the spectrum of clinoptilolite in the range of vibrations of the framework (800–400 cm–1) and T–O bonds (1200–900 cm–1). Hence, the clinoptilolite lattice is largely unaffected by the Ag+ cations due to the low concentration of AgNO3. Kennedy et al. (Reference Kennedy, Khanafer and Tezel2019) and Unaldı et al. (Reference Unaldı, Mızrak and Kadir2013) reported two bands at 253 and 206 cm–1 corresponding to Ag–O stretching vibrations (i.e. outside the spectral range of the present study). Therefore, it cannot be verified whether Ag complexes have been formed following Ag+ cation exchange. Exposure to Ag-clinoptilolite through plasma discharge induced functional changes in the hydroxyl stretching region (Fig. 4c,d). The narrowing and slight shifting of the Ag-clinoptilolite band at 3640 cm–1 (Fig. 4c) may be attributed to the interaction between the hydroxyl group of adsorbed water on clinoptilolite and the Ag metallic forms. The increase in the absorbance intensity of the –OH stretching band might have contributed to the significant increase in the polar surface free energy of the plasma-modified Ag-exchanged zeolite (Taaca & Vasquez, Reference Taaca and Vasquez2017). The interaction is more visible in the framework region, indicating that the structure of the clinoptilolite might have been affected by the plasma treatment. More specifically, the band intensity of the internal tetrahedral bonds (600 cm–1) and the external symmetric O–T–O stretching vibrations (790 cm–1) increased. A similar observation was reported after treatment of an Ag-zeolite (AgZ) composite at a radiofrequency of 13.56 MHz (Osonio & Vasquez, Reference Osonio and Vasquez2018). Non-thermal plasma modification of smectites affected the framework region (530 and 473 cm–1) to a lesser degree compared to the hydroxyl stretching region (4000–2600 cm–1) (Djowe et al., Reference Djowe, Laminsi, Njopwouo, Acayanka and Gaigneaux2013). Finally, the sample exposed to plasma for 60 min showed a new band at ~1500 cm–1. This may be due to excited species produced by argon plasma treatment in this time interval.
The XRD traces of the clinoptilolite, Ag-clinoptilolite and Ar plasma-treated Ag-clinoptilolite for 30 and 60 min are shown in Fig. 5. The presence of clinoptilolite was confirmed by characteristic diffraction lines at 9.88°2θ (d 020 = 8.955 Å), 11.19°2θ (d 200 = 7.899 Å), 17.37°2θ (d 111 = 5.099 Å), 22.43°2θ (d 400 = 3.959 Å), 26.06°2θ (d 222 = 3.416 Å), 30.04°2θ (d 151 = 2.971 Å) and 32.75°2θ) (d 261 = 2.731 Å). After ion exchange with 0.1 M AgNO3, the overall XRD trace was substantially preserved; however, significant variations in the peak intensity were observed compared to the natural clinoptilolite. The significant decrease in intensity of the (020), (200) and (400) diffraction lines after ion exchange is due to the nature, location and amount of extra-framework species (Petrov, Reference Petrov, Ming and Mumpton1995; Concepción-Rosabal et al., Reference Concepción-Rosabal, Rodriguez-Fuentes, Bogdanchikova, Boschc, Avalosb and Lara2005; Dimova et al., Reference Dimova, Petrov, Kadiyski, Lihareva, Stoyanova-Ivanova and Mikli2011; Hubner et al., Reference Hubner, Donati, Quines, Tessaro and Marcilio2020). The 020 diffraction line at 9.88°2θ almost disappeared (Fig. 5) due to modifications in the crystal form. Previous research has reported similar XRD results for clinoptilolite exchanged with transition metal cations (Dimova et al., Reference Dimova, Petrov, Kadiyski, Lihareva, Stoyanova-Ivanova and Mikli2011; Unaldı et al., Reference Unaldı, Mızrak and Kadir2013; Kennedy et al., Reference Kennedy, Khanafer and Tezel2019). The intensity of the (020) peak of Ag-exchanged clinoptilolite varies probably because it is strongly related to the extra-framework species located in the mirror plane perpendicular to the b-axis of the monoclinic structure of clinoptilolite (Hubner et al., Reference Hubner, Donati, Quines, Tessaro and Marcilio2020).

Fig. 5. XRD traces of clinoptilolite (black) and untreated Ag-clinoptilolite (red) compared to the Ag-clinoptilolite treated with plasma for 30 min (blue) and 60 min (pink).
Before plasma treatment, no obvious diffraction peaks belonging to metallic silver were detected in the XRD traces. After the plasma treatment, new peaks corresponding to Ag+ metal ions in the Ag-clinoptilolite sample appeared at 26.42°2θ, 28.03°2θ and 38.20°2θ, corresponding to the (111), (210) and (111) planes of a cubic face-centred lattice of silver, confirming that a silver crystalline structure might have been produced by applying a plasma jet. In addition, most of the clinoptilolite peaks shifted slightly to higher angles after plasma treatment, especially after 30 min of treatment. Moreover, the characteristic XRD peaks of Ag-clinoptilolite exhibit broadening after exposure to Ar plasma (Fig. 5). Similar changes induced by plasma treatment of the structure of the natural zeolite, Ag-zeolite, bentonite clay, etc., have been reported in previous studies (Djowe et al., Reference Djowe, Laminsi, Njopwouo, Acayanka and Gaigneaux2013; Čapková et al., Reference Čapková, Matoušek, Rejnek, Bendlová, Pavlík and Kormunda2016; Osonio & Vasquez, Reference Osonio and Vasquez2018; Valesco-Maldonado et al., Reference Velasco-Maldonado, Harnandez-Montoya, Montes-Moran, Rangel Vasquez and Perez-Cruz2018).
The effect of the plasma treatment on the morphological characteristics of Ag-clinoptilolite was examined by SEM (Fig. 6a–d). The Ag-clinoptilolite showed differences in surface morphology compared to clinoptilolite as a result of mechanical processes such as grinding and mixing. The surface of Ag-clinoptilolite (Fig. 6c,d) is more irregular than the surface of the original clinoptilolite (Fig. 6a,b). The average length of the tabular clinoptilolite crystals was ~2.0 μm, and the thickness was between 0.5 and 2.0 μm. After plasma treatment for 60 min, the cubic and thin platy forms present in the Ag-clinoptilolite might have been caused by the change in the surface charge of the particles, possibly due to the plasma treatment. The energy-dispersive X-ray (EDX) spectra provided semi-quantitative analysis of the Ag+ and other cations in the samples (Fig. 7a,b). The peaks at 3.0 and 3.2 keV (Fig. 7b) are related to metallic silver binding energies, which is in accord with the literature (Rivera-Garza et al., Reference Rivera-Garza, Olguin, Garcia-Sosa, Alcántara and Rodriguez-Fuentes2000; Shameli et al., Reference Shameli, Ahmad, Yunus, Rustaiyan, Ibrahim, Zargar and Abdollahi2010; Copcia et al., Reference Copcia, Luchian, Dunca, Bilba and Hristodor2011; Boschetto et al., Reference Boschetto, Lerin, Cansian, Pergher and Di Luccio2012; Osonio & Vasquez, Reference Osonio and Vasquez2018). Other peaks in the EDX spectra correspond to the binding energies of Ca, K, Na, Si and Al.

Fig. 6. SEM images of (a) clinoptilolite, (b) untreated Ag-clinoptilolite, (c) Ag-clinoptilolite treated with plasma for 30 min and (d) Ag-clinoptilolite treated with plasma for 60 min.

Fig. 7. EDX spectra of (a) clinoptilolite and (b) Ag-clinoptilolite treated with plasma for 60 min.
Summary and conclusions
A natural clinoptilolite from Turkey rich in Ca2+, Mg2+ and K+ with an Al/Si ratio of 5.5 was successfully cation-exchanged with Ag. The Ag+ cations were incorporated within the cavities and/or channels of clinoptilolite. The effects of a DBD-like plasma jet at atmospheric pressure on the structural, composition and morphological properties of Ag-clinoptilolite were assessed using UV–Vis DRS, FTIR, XRD and SEM-EDX analysis. The plasma treatment for 30 and 60 min using Ar as the forming gas successfully reduced Ag+ to its metallic form while maintaining the structure of clinoptilolite. The UV–Vis DRS results showed two absorption peaks at 325 and 370 nm related to Ag clusters. The absorption intensity of both peaks decreased with decreasing plasma treatment time. Before Ar plasma treatment, no obvious diffraction peaks belonging to a metallic silver were detected in the XRD traces, suggesting that silver ions were introduced in the Ag-clinoptilolite cages during the plasma treatment. The FTIR spectra show that exposure of Ag-clinoptilolite to the plasma discharge induced functional changes in the hydroxyl stretching region. The Ag-clinoptilolite exposed to plasma for 60 min showed a new band at ~1500 cm–1, possibly due to excited species produced during the treatment, as was supported by SEM observations. The cubic and thin platy particles appeared on the Ag-clinoptilolite sample treated with plasma for 60 min due to the change in the surface charge of the particles, possibly due to exposure to gaseous discharges. The EDX spectra confirmed the formation of Ag clusters; however, as the oxygen content was still high, silver oxide might also be present. Based on the present investigation, the suggested method of plasma treatment may be effective, saving both energy and time.
Acknowledgements
The authors thank Prof Dr Güneş S. Kürkçüoğlu (Eskişehir Osmangazi University) for her support in the use of the FTIR spectrophotometer. The authors also thank Orhan Çetin of the technical personnel of the Ceramic Research Center of ESTU for the X-ray fluorescence analysis.