Introduction
The Earth’s climate is undergoing change, and the consensus is that the principal cause is the increase in the atmosphere of so-called ‘greenhouse gases’ from anthropogenic activities. In particular, carbon dioxide generated by the combustion of fossil forms of carbon (Le Quéré et al. Reference Le Quéré, Raupach and Canadell2009) and also methane, often generated by agriculture (Smith et al. Reference Smith, Martino and Cai2008), are major sources of these gases in the atmosphere. These gaseous forms of carbon are also released into the atmosphere when formerly frozen sources such as Arctic permafrost regions are warmed (Schuur and Abbott Reference Schuur and Abbott2011).
Many authors in the scientific literature (e.g. Stockmann et al. Reference Stockmann, Adams and Crawford2013; Minasny et al. Reference Minasny, Malone and McBratney2017) have proposed that soil organic matter (SOM) can be used as a “managed” sink for atmospheric carbon gases and particularly carbon dioxide, through carbon sequestration. Politicians and others in public life have seen carbon sequestration as a solution to the problems that increasing emissions of these gases cause for the climate. A strong need exists, therefore, to assess the viability of the apparently useful role of SOM in halting or at least diminishing the advance of climate change.
The global stock of carbon in the soil as organic matter has been estimated as ~700 Pg to a depth of 30 cm and ~1500 Pg to a depth of 1 m (Batjes Reference Batjes1996). The SOM to 30 cm depth represents about twice the amount of C in the atmosphere and three times that in above-ground vegetation (Powlson et al. Reference Powlson, Whitmore and Goulding2011). Carbon as soil organic carbon (SOC) is particularly labile. Historically, the reservoir of C held in land has diminished drastically over decades, whereas that held in oceans, which is also labile and of a comparable size, has changed only a little (Lal Reference Lal, Hartemink and McSweeney2014). The loss of C from land is attributable to agriculture. Many (e.g. Lal Reference Lal, Hartemink and McSweeney2014) have concluded that, because C has been lost from their soils, managed agricultural soils have the capacity to replenish the losses and hence sequester C from the atmosphere.
The clay fraction of soils, comprising clay minerals (including metal oxides, oxyhydroxides, and hydroxides) is their most reactive fraction for forming associations with organic matter (Churchman Reference Churchman2010, Reference Churchman2018; Sarkar et al. Reference Sarkar, Singh, Mandal, Churchman, Bolan, Garcia, Nannipieri and Hernandez2018; Singh et al. Reference Singh, Sarkar, Sarkar, Churchman, Bolan, Mandal, Menon, Purakayastha and Beerling2018).
Several approaches have been used to investigate the importance of clay minerals in the uptake and retention, hence, potentially, the sequestration of carbon in soils (e.g. Churchman and Velde Reference Churchman and Velde2019). These include correlations of the contents of SOC with properties of soil clays, including their contents and their cation exchange capacities (CECs). Where narrow ranges of soil types have been studied, some good correlations have been obtained, but where the ranges are wider, Soil organic carbon contents and those of clays or their properties have been poorly correlated, if at all (Churchman and Velde Reference Churchman and Velde2019). In tropical Australia (Spain Reference Spain1990), such correlations were poor when soils originating from basalt were included, and a set of 167 soils taken from throughout New Zealand showed no correlation between SOC and clay fraction contents (Percival et al. Reference Percival, Parfitt and Scott2000). The lack of a relationship between SOC and clay contents or properties was explained by the important roles played by compounds of Fe (Spain Reference Spain1990; Percival et al. Reference Percival, Parfitt and Scott2000) and Al (Percival et al. Reference Percival, Parfitt and Scott2000), particularly when these were poorly crystalline (Percival et al. Reference Percival, Parfitt and Scott2000).
Another approach to the study of mineral–organic interactions in soils is the fractionation of soils, either by particle density, selective chemical dissolutions, or physical disaggregation. These studies have confirmed the important roles played by Fe (Eusterhues et al. Reference Eusterhues, Rumpel, Kleber and Kőgel-Knabner2003, Reference Eusterhues, Rumpel and Kőgel-Knabner2005) in the stabilization of SOC in many soils, and by Ca2+ in some (Oades Reference Oades, Dixon and Weed1989). In particular, leached, hence, generally acid, soils involve Fe and Al in the stabilization of C by bridging while Ca2+ is more involved in bridging with SOC in high base status soils (Oades Reference Oades, Dixon and Weed1989; Rowley et al. Reference Rowley, Grand and Verrecchia2018).
Studies of incubations of soils revealed that clay minerals with the largest surface area, namely allophane and smectite, in the soils used had the strongest effect on the suppression of the decomposition of SOC (Saggar et al. Reference Saggar, Parshotam, Sparling, Feltham and Hart1996), while Rasmussen et al. (Reference Rasmussen, Matsuyama, Dahlgren, Southard and Brauer2007) found that poorly crystalline Fe oxyhydroxides strongly suppressed SOC decomposition.
These three approaches, together with others (e.g. Churchman and Velde Reference Churchman and Velde2019), have shown that clay minerals, including metal oxides, oxyhydroxides, and hydroxides, are important in the uptake and retention of SOC in soils. Even so, these studies do not provide an assessment of the strength and duration of the binding of SOM by soil minerals. Strong and long-term binding of SOM is required for the sequestration of carbon in soils.
“Sequester” is defined as “to hold on to” or “to keep separate” (Powlson et al. Reference Powlson, Whitmore and Goulding2011). In the chemical or environmental context, sequestration means “the trapping of a chemical in the atmosphere or environment and its isolation in a natural or artificial storage area” (Dictionary.com 2019). The sequestration of organic C in soils is generally taken to mean the retention of SOC for a “stipulated duration timeframe (usually 100 years)” (Stockmann et al. Reference Stockmann, Adams and Crawford2013). The most useful meaning for carbon sequestration for a decrease in greenhouse gases in the atmosphere is given by a net gain of SOC. This represents “new” sequestration of carbon.
Several recent experiments (Singh et al. Reference Singh, Sarkar, Biswas, Churchman and Bolan2016, Reference Singh, Sarkar, Biswas, Bolan and Churchman2017a, Reference Singh, Sarkar, Hussain, Ok, Bolan and Churchman2017b, Reference Singh, Sarkar, Bolan, Ok and Churchman2019; Schapel et al. Reference Schapel, Marschner and Churchman2018) were carried out to help assess or improve the effectiveness of soils for the uptake and retention of OC. Experiments were carried out in the laboratory and also in the field.
The laboratory experiments included determinations of the stabilization of OC by clay minerals as they occur in soils, in contrast to clay minerals from deposits, as determined by Saidy et al. (Reference Saidy, Smernik, Baldock, Kaiser, Sanderman and Macdonald2012). Clay minerals originating in soils can have quite different properties from “pure” clay minerals from deposits (Churchman Reference Churchman2010; Churchman et al. Reference Churchman, Hesterberg and Singh2012; Churchman and Lowe Reference Churchman, Lowe, Huang, Li and Sumner2012; Churchman and Velde Reference Churchman and Velde2019).
The field experiments were based on a common agricultural practice in much of the States of South Australia and Western Australia where sandy soils often become hydrophobic in summer in the xeric moisture conditions prevailing in these areas. A practical solution to this problem has been to add clay to the soils (McKissock et al. Reference McKissock, Gilkes and Walker1998; Cann Reference Cann2000; Churchman et al. Reference Churchman, Noble, Bailey, Chittleborough, Harper, Hartemink and McSweeney2014; Churchman and Velde Reference Churchman and Velde2019). Initially, clay-rich material was incorporated in the surface soils but, because most of the soils concerned are texture-contrast types having sandy topsoils over clay-rich subsoils, the common practice now is to obtain clay for incorporation in surface soils via mechanical deep ripping, a process known as “delving” (Betti et al. Reference Betti, Grant, Churchman and Murray2015).
The results of these various experiments have enabled an assessment of the potential of soils to take up and retain added OC, especially in relation to their clay mineral composition. Reference to the literature has also pointed to likely causes of limitations on carbon uptake and retention in soils.
In this paper, some of the important results and conclusions of these various recent experiments, particularly from Singh (Reference Singh2016), Singh et al. (Reference Singh, Sarkar, Biswas, Churchman and Bolan2016, Reference Singh, Sarkar, Biswas, Bolan and Churchman2017a), and Schapel et al. (Reference Schapel, Marschner and Churchman2018), are highlighted and assessed together with results from earlier work, especially Churchman et al. (Reference Churchman, Noble, Bailey, Chittleborough, Harper, Hartemink and McSweeney2014), as indicators of the prospects for (new) carbon sequestration in soils.
The hypothesis is that clays, as the most reactive inorganic compounds in soils, ultimately govern the prospects for sequestration of organic carbon in soils.
LABORATORY EXPERIMENTS AND RESULTS
Three soils were studied: a Calcic Haplosteralf, a Pellustert, and a Thaptic Haploxerand, according to Soil Taxonomy (Soil Survey Staff 1975) (Singh et al. Reference Singh, Sarkar, Hussain, Ok, Bolan and Churchman2017b). Respectively, these were dominated by kaolinite and illite (together), smectite, and allophane (Singh et al. Reference Singh, Sarkar, Biswas, Churchman and Bolan2016). The OC added to soils in these experiments was in liquid form, as dissolved organic carbon (DOC), produced by dissolving ground wheat straw in water. Experiments were performed to assess the capacities of three different soils to: (1) adsorb DOC, (2) retain adsorbed DOC in the presence of water (or “desorb DOC”) (Singh Reference Singh2016, Singh et al. Reference Singh, Sarkar, Biswas, Churchman and Bolan2016), and (3) mineralize added carbon and limit the decomposition of adsorbed DOC to carbon dioxide by the "priming effect” (Singh et al. Reference Singh, Sarkar, Biswas, Bolan and Churchman2017a).
Adsorption Experiments
Adsorption experiments were performed on clay (<2 μm) fractions extracted from the soils (at 5–20 cm depth) following prolonged shaking in Milli-Q water then gravity sedimentation. Hence, both minerals and organic matter in clay-sized material were preserved in the collected fractions. The soils, all from South Australia, were characterized by their dominant clay minerals: (a) kaolinite and illite together, (b) smectite, and (c) allophane (Singh et al. Reference Singh, Sarkar, Biswas, Churchman and Bolan2016, Reference Singh, Sarkar, Hussain, Ok, Bolan and Churchman2017b). Adsorption was carried out at pH 7 in solutions of Ca or Na nitrate, either 0.1 or 0.01 M, for 24 h, and DOC in solution was analyzed after filtering. See Singh et al. (Reference Singh, Sarkar, Biswas, Churchman and Bolan2016) for details.
The various clay minerals adsorbed DOC to various extents. All sorption isotherms (for natural clay fractions, clay fractions after OC removal, and clay fractions after iron removal following OC removal) followed the Langmuir model (Singh et al. Reference Singh, Sarkar, Biswas, Churchman and Bolan2016). Results of maximum OC adsorption by the clay fraction of each soil are summarized in Table 1 for the following conditions: untreated, after organic carbon removal, and after iron removal following organic carbon removal. These data are from adsorption in 0.01 M Ca(NO3)2, a concentration likely to represent closely typical soil solutions (Blakemore et al. Reference Blakemore, Searle and Daly1987). Analyses of total C and specific surface areas, before and after chemical treatments, as well as Fe contents of the untreated clay fractions (from Singh Reference Singh2016) are also included in Table 1. Similar trends were observed for the nitrate solutions differing in terms of Ca or Na content and with different concentrations (0.01 or 0.1 M). The sorptive capacities of all clays were increased when native OC was removed and were reduced when Fe was removed.
Table 1. Maximum amounts (mg g–1) of DOC adsorbed in 0.01 Ca (NO3)2 by the clay fractions of soils with various dominant minerals in relation to their contents of C and Fe and their specific surface areas (SSAs) calculated using the BET equation for adsorption by N2 gas (data from Singh Reference Singh2016).
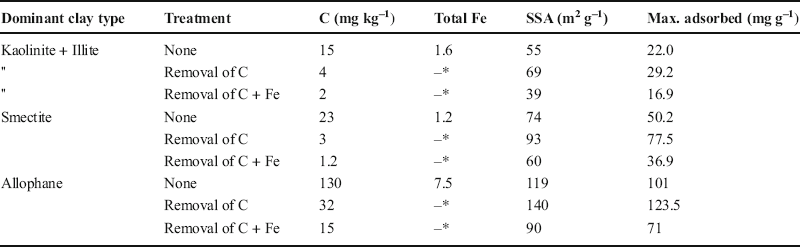
*Not determined
Desorption Experiments
Clay fraction samples taken from the adsorption experiments at various levels of equilibrium concentrations of DOC were first washed with Milli-Q water to remove entrained DOC, then equilibrated for 24 h, and supernatants were analyzed for DOC after filtering. See Singh et al. (Reference Singh, Sarkar, Biswas, Churchman and Bolan2016) for details.
The percentages of adsorbed C that were desorbed in water from each clay fraction (i.e. without treatment, after OC removal, and after iron removal following OC removal) in relation to the amounts of DOC added in the adsorption experiments are shown in Table 2. These data are from adsorption in 0.01 M Ca(NO3)2. Similar trends were observed for desorption from clay fractions following adsorption from either Ca or Na nitrate solution regardless of concentration (0.01 or 0.1 M) (Singh et al. Reference Singh, Sarkar, Biswas, Churchman and Bolan2016).
Table 2. Percent desorption of DOC in water after its adsorption in 0.01 Ca (NO3)2 at various loadings from the clay fractions of soils with various dominant minerals sequentially treated to remove native C and Fe (data from Singh Reference Singh2016).
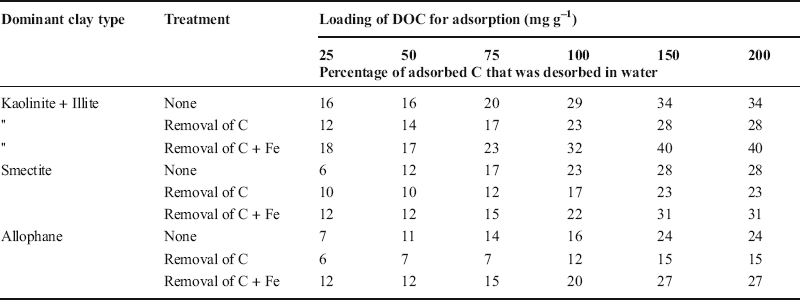
More than 30% of the C adsorbed in Ca nitrate and more than 50% of that adsorbed in Na nitrate was released on simple desorption in water (Singh et al. Reference Singh, Sarkar, Biswas, Churchman and Bolan2016). The proportion of adsorbed C that was desorbed was greatest for the kaolinite-illite clay, less for the smectite clay, and least for the allophanic clay, but even in this last case, ~20% of that adsorbed in Ca nitrate and ~30% of that adsorbed in Na nitrate was lost on desorption in water. In each case, the proportion of adsorbed C that was lost on desorption increased when DOC adsorption had been performed on clays from which Fe oxide was removed and diminished when adsorption had occurred on clays from which native C was removed.
Effects of Priming
Samples of the whole soils that had been sieved to <2 mm to remove coarse particles were equilibrated with 14C-labeled malic acid and also unamended in sterile Milli-Q water. CO2 from respiration was collected at different time periods in a solution of NaOH and the unconsumed alkali was determined by titration with HCl. See Singh et al. (Reference Singh, Sarkar, Biswas, Bolan and Churchman2017a) for details of the experiments and calculations.
The results showed that more C was lost (as CO2) from all soils after priming with malic acid (Singh et al. Reference Singh, Sarkar, Biswas, Bolan and Churchman2017a). However, the extent of loss on priming depended on the mineralogy of the soils, with more C lost from the smectite soil than from the soil dominated by illite-kaolinite and the allophanic soil. These two latter soils had similar losses on priming. Decomposition of native C increased by 108% in the smectitic soil upon priming and by 37% in the kaolinitic-illitic soil and 42% in the allophanic soil (Singh et al. Reference Singh, Sarkar, Biswas, Bolan and Churchman2017a).
FIELD EXPERIMENTS AND RESULTS
Soils in South Australia that were classified as Luvisols in the World Reference Base (Michéli and Spaargaren Reference Michéli, Spaargaren, Huang, Li and Sumner2012) were studied at four sites where clay amendments had been carried out. All soils were texture-contrast types. Farming systems in all sites were rotations of cereal and grazed pasture, using minimum or no tillage and stubble retention. At each site, the soil had been amended by both incorporation of clay-rich soils from subsoils within pits dug nearby and also by deep incorporation in situ using mechanical means. Trials were sampled at depth intervals to 50 cm after at least 3 years following treatments. See Schapel et al. (Reference Schapel, Marschner and Churchman2018) for detailed descriptions of the sites and processes used for clay amendment.
Soils were sampled during the non-growing phase as cores from various depths. They did not contain gravel so were ground to pass a <2 mm sieve. Homogenized samples were analyzed for OC by Walkley-Black wet digestion and bulk densities were determined gravimetrically for known volumes of soil. See Schapel et al. (Reference Schapel, Marschner and Churchman2018) for details of the methods.
Schapel et al. (Reference Schapel, Marschner and Churchman2018) found that the stock (or reservoir) of OC was significantly and positively correlated (R2 = 0.47) with the stock (or reservoir) of clay when these values were adjusted to an equivalent soil mass (ESM) from bulk density determinations.
The results showed that OC stock (or reservoir) in the surface (0–30 cm) of 14 clay-amended soils increased on average by 5.5 Mg C ha–1 (range –1.6 to 14.2 Mg C ha–1) from unamended sands, with the increases brought about largely by increases in clay content. Clay and OC were concentrated at the surface in clay-spread treatments and at depth in delved treatments (Schapel et al. Reference Schapel, Marschner and Churchman2018).
Discussion
Organic carbon in solution is adsorbed by soils to various extents, and especially by their most reactive clay fractions and depending on their clay mineral composition. The uptake of OC correlates with the specific surface area of the soil clay fractions (Table 1). Uptake of OC was increased by the presence of iron oxides, as also demonstrated by Kahle et al. (Reference Kahle, Kleber and Jahn2003, Reference Kahle, Kleber and Jahn2004). Some organic matter in natural soils is held particularly strongly, and, notwithstanding that a small fraction was even resistant to peroxidation, the oxidation of OC enables further uptake. Other workers, e.g. Kaiser and Guggenberger (Reference Kaiser and Guggenberger2003) and Mikutta et al. (Reference Mikutta, Kleber and Jahn2005), have also found that some OC is held particularly strongly in soils. The strongly held portion may be located within small pores (McCarthy et al. Reference McCarthy, Ilavsky, Jastrow, Mayer, Perfect and Zhuang2008).
Desorption shows that considerable organic matter was easily lost in water (Table 2). OC was held more strongly, i.e. less was released on desorption, after removal of native OC had occurred prior to its uptake. OC was held less strongly when Fe oxides were removed prior to its uptake. Nonetheless, much adsorbed OC was quite labile. Kahle et al. (Reference Kahle, Kleber and Jahn2004), Mikutta et al. (Reference Mikutta, Mikutta, Kalbitz, Scheel, Kaiser and Jahn2007), and Saidy et al. (Reference Saidy, Smernik, Baldock, Kaiser and Sanderman2013) found that OC adsorbed on pure clay minerals included a substantial portion that was lost easily on desorption.
The results of the priming experiments showed that, for all the soils, addition of extra OC led to an enhanced release of CO2 due to stimulation of microbial activity, hence respiration. Singh et al. (Reference Singh, Sarkar, Biswas, Bolan and Churchman2017a) attributed the greater effect of priming in the smectitic soil than in the allophanic soil or the soil dominated by kaolinite and illite to the higher microbial activity which they measured for the smectitic soil. Even so, priming, showing breakdown of SOC, occurred even in the allophanic soil, where OC was held more strongly against desorption than in the other two soils studied. This confirms the results of Finley et al. (Reference Finley, Dijkstra, Rasmussen, Schwartz, Mau, Liu, van Gestel and Hungate2018), who found a high rate of decomposition of native OC in soils comprising the short-range order minerals, allophane and ferrihydrite.
Field experiments (Schapel et al. Reference Schapel, Marschner and Churchman2018) demonstrated that both direct incorporation of added exogenous clay and ‘delving’ of in situ clay resulted in an increase in SOC content. Increases in SOC were also observed in Western Australia 8 years after additions of subsoil kaolinitic clay to sandy topsoils by delving (Hall et al. Reference Hall, Jones, Crabtree and Daniels2010). The top 10 cm of soil contained only 1% clay, which increased to 6% clay on delving. The increase in SOC was 0.2%. In South Australia, Bailey and Hughes (Reference Bailey, Hughes, Burkitt and Sparrow2012) found that delving soils in 11 sites increased the mean SOC contents of their A2 horizons from 0.3% to 0.7%, Other clayey material can also enable increases in SOC, and wastes from bauxite processing (85% silt, 11% clay, and 4% sand) were added to sandy soils in Western Australia (Harper et al. Reference Harper, Sochacki and Bell2012; Churchman et al. Reference Churchman, Noble, Bailey, Chittleborough, Harper, Hartemink and McSweeney2014). Over a 19–20 year period, addition of this waste led to increases in SOC in the top 30 cm of soil of between 0.1 to 0.65%, resulting in a significant (P<0.01) increase of 11.6 Mg C ha–1 across the sites with a strong (r 2 = 0.93, P<0.001) curvilinear correlation between clay content and SOC for the 0–5 cm layer (Churchman et al. Reference Churchman, Noble, Bailey, Chittleborough, Harper, Hartemink and McSweeney2014). This amounts to an annual uptake of 1.9 Mg CO2-eq. ha–1 year -1.
Implications for Carbon Sequestration in Soils
Carbon is turned over – and lost from the soil system – through the biological processes needed for soil functioning in agriculture (e.g. Janzen Reference Janzen2006). Sequestration of C through long-term storage and the use of soil for growing plants appear to have conflicting aims. For example, Bolan et al. (Reference Bolan, Kunhikrishnan, Choppala, Thangarajan and Chung2012) concluded that amending soils with biochar, which is more stable than composts, could enhance soil carbon sequestration (long-term carbon storage) but this carbon might not be useful for an immediate improvement of soil health, such as for supplying food to soil microorganisms for keeping the nutrient cycling operational. Following Janzen (Reference Janzen2006), the most efficient way of extracting extra carbon as CO2 from the atmosphere may be by using it more rapidly for increasing plant production, hence flow-through of SOM.
Increased adsorption which occurred when native C was first removed from all soils (Singh et al. Reference Singh, Sarkar, Biswas, Churchman and Bolan2016) shows that some C had occupied the most reactive sites. The results from desorption (Singh et al. Reference Singh, Sarkar, Biswas, Churchman and Bolan2016) show that further C occupied less reactive sites in all soils and was easily removed. The effect of priming (Singh et al. Reference Singh, Sarkar, Biswas, Bolan and Churchman2017a) shows that simple addition of new C to soils may result in the loss of C that is already present. Overall, these results show that simply adding new C to soils that already contain C does not result in additional C that is held strongly, i.e. sequestered.
However, the addition of clays to soils can enable the net uptake of C. Furthermore, the rate of incubation of wheat residues added to a sandy soil was retarded by the addition of the clay fractions used in the studies of sorption of DOC (Singh et al. Reference Singh, Sarkar, Bolan, Ok and Churchman2019). The addition of clay produces new surfaces for holding C.
According to Churchman and Velde (Reference Churchman and Velde2019, p. 152), “SOC can be associated with almost any secondary (and also altered primary) minerals, but shows a preference for poorly crystalline oxides and also silicates of Fe and Al.” If these are rare, SOC binds to phyllosilicates according to their relative surface reactivities, with smectites being the most reactive of these. pH also plays a role in governing reactivities of minerals for SOC. Low pH favors Fe and Al compounds and higher pH favors phyllosilicates. The type, hence strength, of these associations also varies with pH. Associations between SOC and minerals tend to be inner-sphere, hence stronger, at low pH and outer-sphere at high pH (Kleber et al. Reference Kleber, Eusterhues, Keiluweit, Mikutta, Mikutta and Nico2015).
Various functional groups in organic matter can be bound to minerals in layers. Proteins and molecules containing carboxyl groups occur commonly adjacent to mineral surfaces while molecules containing hydrophobic groups tend to occur in outer layers (e.g. Kleber et al. Reference Kleber, Sollins and Sutton2007). The layering of organic molecules around the clay minerals is onion-like. Functional groups with a strong attraction to minerals are likely to form long-lasting associations with them early in soil development. Proteins provide an example in which they are attracted to the negative charges that characterize many clay minerals, especially 2:1 (Si:Al) phyllosilicates via electrostatic bonds through –NR 3 +groups (where R is H or CH x with x = 1–3). The same applies to carboxyl and O/N alkyl groups, which form covalent bonds with Fe oxides that may be free or else associated with phyllosilicates (Schöning et al. Reference Schöning, Knicker and Kőgel-Knabner2005).
Organic matter most intimately held on mineral surfaces would be held for longer times than that beyond the surfaces. Even so, associations of organic matter with minerals in soils often occur in 3-dimensional entities known as microaggregates. These are distinguished from macroaggregates by size, although the demarcation between the two may be arbitrary and empirical (Totsche et al. Reference Totsche, Amelung and Gerzabeck2018). In the literature, microaggregates are defined as being, variously, in the <250 μm (Totsche et al. Reference Totsche, Amelung and Gerzabeck2018), 53–250 μm (Beare et al. Reference Beare, Hendrix and Coleman1994; Six et al. Reference Six, Elliott and Paustian1999; Denef et al. Reference Denef, Six, Merckx and Paustian2004), and/or 2–50 μm size ranges (Tisdall and Oades Reference Tisdall and Oades1982; Paradelo et al. Reference Paradelo, van Oort, Barré, Billiou and Chenu2016), while Liefeld and Kőgel-Knabner (Reference Liefeld and Kögel-Knabner2003) measured their mean weight diameters in a range of soils as being 11.8–15.6 μm. Using transmission electron microscopy of thin slices of soil, Chenu and Plante (Reference Chenu and Plante2006) and Churchman et al. (Reference Churchman, Foster, DAcqui, Janik, Skjemstad, Merry and Weissmann2010) found many microaggregates involving mineral and organic associations in the size range of 1–5 μm.
In any case, microaggregates provide the basis of the structure of soils, stabilizing them against disruption by agricultural practices and erosion. In particular, SOC is stabilized in microaggregates. In microaggregates, organic matter often occurs within “shells” of the minerals (Chenu and Plante Reference Chenu and Plante2006; McCarthy et al. Reference McCarthy, Ilavsky, Jastrow, Mayer, Perfect and Zhuang2008; Churchman et al. Reference Churchman, Foster, DAcqui, Janik, Skjemstad, Merry and Weissmann2010).
Organic matter turnover in microaggregates has been estimated variously in at least four different studies as from ~100–1000 years (Churchman and Velde Reference Churchman and Velde2019). Studies of chronosequences (Totsche et al. Reference Totsche, Amelung and Gerzabeck2018) have shown that the accretion of newly formed soil components such as microbial residues or hydrous Fe oxides into microaggregates appears to take place within ~200 y. Turnover time is slow even in larger, silt-size aggregates (Virto et al. Reference Virto, Moni, Swanston and Chenu2010).
In truth, gases such as carbon dioxide are not removed directly from the atmosphere by soils or SOM. Generally, they are taken up by plants for photosynthesis leading to their growth and release into the soils. This occurs during the growth of plants, e.g. through root exudates or after their death, by their decomposition. The extra OC added to soils when clay was added to overcome non-wetting (Churchman et al. Reference Churchman, Noble, Bailey, Chittleborough, Harper, Hartemink and McSweeney2014; Schapel et al. Reference Schapel, Marschner and Churchman2018) came about because addition of clays encouraged plant growth, mainly by holding water on their large hydrophilic surfaces for later release to plant roots. In other experiments, additions of clays to light-textured (sandy) soils have been shown to increase plant production. In the laboratory, Churchman and co-workers (unpub. results 2001) found that addition of 12.5% by weight of foundry waste containing 35% bentonite to a non-wetting sandy soil from South Australia not only overcame its hydrophobicity but trebled dry matter production. In the field, addition of similar foundry waste to a sandy soil in Thailand (without non-wetting problems) also led to increases of up to three times in the dry matter yield of maize (Soda et al. Reference Soda, Noble, Suzuki, Simmons, Sindhusen and Bhuthornharaj2006). Pot trials adding 40 t ha–1 to a degraded Oxisol and a sandy Ultisol from northern Australia showed increases in the biomass production of forage sorghum of three and eight times, respectively (Noble et al. Reference Noble, Gillman, Nath and Srivastava2001). Carbon contents were not measured in these various experiments, but the increased plant growth in each case would have led to substantial increase in SOC. Wherever excess clay is available locally, increased retention, if not new sequestration, of C can occur in sandy soils where sparse plant growth is the norm.
Limited sources of clays occur as industrial wastes, with, for example, ~ one million tons of “bleaching earths,” which are acid-activated bentonites, being released as wastes from cooking oil manufacture world-wide each year (Crossley Reference Crossley2001), as well as from some foundries. However, huge areas of sandy soils are found world-wide (>900 million hectares, according to FAO/UNESCO 1995) and their augmentation by clays from these sources offers prospects for only limited overall sequestration of carbon.
The prospects for the (new) sequestration of C are constrained by (1) the availability of reactive surfaces, and (2) the possible formation of microaggregates. Most surfaces in soils are mineral rather than organic (Mayer and Xing Reference Mayer and Xing2001), with microorganisms and organic matter occupying only a very small fraction (<<1%, according to Kleber et al. Reference Kleber, Eusterhues, Keiluweit, Mikutta, Mikutta and Nico2015) of soil surfaces. Selective spots, variously described as “organo-mineral clusters with rough surfaces” (Vogel et al. Reference Vogel, Mueller and Hőschen2014, p. 5), appear to occur for the uptake of OC in soils. Etch pits, micropores, and cracks on mineral surfaces are likely to constitute such spots (Churchman and Velde Reference Churchman and Velde2019), which are probably related to those for microorganisms, and include the rhizospheres of plants (Hinsinger et al. Reference Hinsinger, Bengouh, Vetterlin and Young2009). Hence, the opportunities for uptake and especially for strong uptake, i.e. sequestration, of C are quite limited.
Carbon sequestration takes place in microaggregates but takes a long time, so that the formation of microaggregates does not provide an immediate solution to the removal of newly released greenhouse gases from the atmosphere. Nonetheless, formation of microaggregates takes place within macroaggregates (Oades Reference Oades1984; Golchin et al. Reference Golchin, Oades, Skjemstad and Clarke1994; Six et al. Reference Six, Elliott and Paustian1999; Balesdent et al. Reference Balesdent, Chenu and Balabane2000) and macroaggregate formation is favored by vigorous plant growth (Six et al. Reference Six, Bossuyt, Degryze and Denef2004). Although strong plant growth may not sequester C in the long term, its promotion could remove some C from the atmosphere. The removal of C by isolation in larger macroaggregates is transitory (Balesdent Reference Balesdent1996; Puget et al. Reference Puget, Chenu and Balesdent2000; Six et al. Reference Six, Conant, Paul and Paustian2002), but continues as long as the strong plant growth is maintained. The process “buys time” while possible new technologies may become available, as Minasny et al. (Reference Minasny, Malone and McBratney2017) claimed for the “4 per mille” initiative for increasing soil C worldwide. The C isolated in macroaggregates may be transferred into microaggregates and, hence, truly sequestered, but only in the very long-term.
Soil management has often been proposed as the key to increasing the sequestration of C (Lal Reference Lal2004, Reference Lal, Hartemink and McSweeney2014; Minasny et al. Reference Minasny, Malone and McBratney2017). West and Six (Reference West and Six2007) postulated that step-wise increases in the capacity of soils to sequester C with distinct changes occur in soil management. The introduction of no-till farming (NT) is a common management strategy, for example, but considerable evidence suggests that NT may only change the distribution of C within the soil profile (Luo et al. Reference Luo, Wang and Sun2010), generally toward the surface of profiles.
Even so, the experiments reported here, as well as those cited in discussion, involve surface soils. Studies of organic matter in subsoils, e.g. Lorenz and Lal (Reference Lorenz and Lal2005), Chabbi et al. (Reference Chabbi, Kögel-Knabner and Rumpel2009), and Salomé et al. (Reference Salomé, Nunan, Pouteau, Lerch and Chenu2010), have found several characteristics of organic matter in subsoils that suggest that its functioning and stabilization differs from that in topsoils. For example, (some) subsoil SOC may be very old (several thousands of years old) (Chabbi et al. Reference Chabbi, Kögel-Knabner and Rumpel2009) and strongly bound to minerals (Chabbi et al. Reference Chabbi, Kögel-Knabner and Rumpel2009), including in structural units (Salomé et al. Reference Salomé, Nunan, Pouteau, Lerch and Chenu2010). Subsoil SOC appears to have high spatial heterogeneity (Chabbi et al. Reference Chabbi, Kögel-Knabner and Rumpel2009; Salomé et al. Reference Salomé, Nunan, Pouteau, Lerch and Chenu2010) and may not be subject to a priming effect (Salomé et al. Reference Salomé, Nunan, Pouteau, Lerch and Chenu2010). Sequestration of C in subsoils may be brought about by the use of plants/cultivars with deep and thick root systems (Lorenz and Lal Reference Lorenz and Lal2005). Nonetheless, new C sequestration in subsoils is also subject to consideration of the degree of saturation of mineral surfaces. If fully saturated, they are not good candidates for net sequestration. Certainly the subsoils in the texture-contrast soils studied by Schapel et al. (Reference Schapel, Marschner and Churchman2018) and also others discussed by Churchman et al. (Reference Churchman, Noble, Bailey, Chittleborough, Harper, Hartemink and McSweeney2014) proved to be useful for net uptake of C; hence, good candidates for C sequestration.
Soils have a limited capacity for OC (Hassink Reference Hassink1997; Stewart et al. Reference Stewart, Plante, Paustian, Conant and Six2008). The limits for C sequestration, rather than for overall, may be partly transitory, uptake of C should reflect the capacity of reactive mineral surfaces for carbon. Such limits are likely to be somewhat less than the total capacity of soil for C. Following analyses of several results from field trials of the addition of C to soils, Stewart et al. (Reference Stewart, Paustian, Conant, Plante and Six2007) proposed an “effective stabilization capacity” which defines the “maximum sequestration possible with increasing C input level under a particular management scenario.” Discussion in the present work confirms the validity of the Stewart et al. (Reference Stewart, Paustian, Conant, Plante and Six2007) “effective stabilization capacity” concept, but through the use of laboratory experiments and field experiments in which clays were added to soils. If, or when, the adsorption capacity of its reactive mineral surfaces is reached, little or no prospect exists for new sequestration of C from the atmosphere into a soil.
Conclusions
The results of recent laboratory experiments on the interaction of DOC with various southern Australian soil clays and of field experiments on the effects on carbon of additions of clays to a southern Australian soil, together with the literature, have been interpreted in the context of prospects for the sequestration of carbon in soils. The amount of organic carbon (OC) that can be adsorbed by soils depends on their clay minerals, including Fe oxides. However, much of the OC that can be adsorbed by soils can also be lost easily, and substantial OC in soils can be lost when more is added (by priming). Nonetheless, some (native) OC is held strongly in soils. OC close to mineral surfaces or within microaggregates is held most strongly. Even so, only very limited areas of mineral surfaces allow the uptake of OC, and when reactive surfaces are occupied, new C will not be held strongly. The addition of clay provides new surfaces for the uptake and retention of C.
Overall, one can conclude that net new sequestration of C in soils cannot be achieved by simply adding C to unamended (top) soils, although net C sequestration may occur in subsoils. On the other hand, increasing the isolation of OC within macroaggregates by enhancing plant growth, although transitory, may provide a more effective method of decreasing atmospheric C than by its sequestration.
CONFLICTS OF INTEREST
On behalf of all authors, the corresponding author states that there is no conflict of interest.