1. Introduction
Faults and folds are essential parts of any mountain belt and determine the style of the deep structure of the orogen. In addition the distinction between crystalline basement rocks and sedimentary strata are key features to further characterize this style. As early as 1949 Rodgers used the presence of crystalline rocks in the Appalachians to make a distinction between thick-skinned and thin-skinned tectonics (Rodgers, Reference Rodgers1949). Hereby, thin-skinned tectonics implies that thrust sheets are made of sedimentary strata only, whereas in thick-skinned tectonics crystalline basement is involved in thrusting as well. If collision orogens are considered it emerges quickly that basement involvement may occur in different settings. Relatively thin thrust sheets made up of mainly crystalline rocks are piled up in the core of the Alpine orogen (e.g. Heim, Reference Heim1919–22), the Caledonides of Scotland (Elliott & Johnson, Reference Elliott and Johnson1980) or Scandinavia (Heim, Schärer & Milnes, Reference Heim, Schärer and Milnes1977). On the other hand, thrust faults affecting the entire crust have been reported from the foreland of the North American and Andean cordilleras (e.g. Smithson et al. Reference Smithson, Brewer, Kaufman, Oliver and Hurich1978) or the Alice Springs orogen in Australia (Teyssier, Reference Teyssier1985). These examples correspond to truly thick-skinned tectonics. To better distinguish these cases it is suggested to restrict the term thick-skinned tectonics to the cases where the entire crust is involved, and use the term basement-involved thin-skinned tectonics for the cases where thrust sheets are made of upper crustal flakes only (Pfiffner, Reference Pfiffner, Mazzoli and Butler2006).
In thin-skinned tectonics sedimentary strata are detached from their basement along a decollement horizon made up of rocks with low shear strength. Typical examples include evaporites (salt or anhydrite) and shales. In most cases there are several thrust faults (or thrust sheets) involved with thin-skinned tectonics. While the thrust faults sole into the decollement horizon, they climb up section in the direction of transport forming ramps across mechanically strong layers such as, for example, carbonates.
The Sevier fold-and-thrust belt in Wyoming shown in Figure 1a is a classic example of thin-skinned tectonics. Decollement horizons are present in Neoproterozoic micaceous rocks (Paris and Meade thrusts), Cambrian shales for all of the thrust faults and Triassic evaporites (Prospect thrust; Royse, Reference Royse, Snoke, Steidtmann and Roberts1993). Thrusting can be dated based on Cenozoic synorogenic clastic rocks (Royse, Reference Royse, Snoke, Steidtmann and Roberts1993; DeCelles, Reference DeCelles1994, Reference DeCelles2004; Yonkee & Weil, Reference Yonkee and Weil2015). According to these authors, thrusting migrated from west to east: the Paris thrust in Early Cretaceous time, the Meade and Crawford in Late Cretaceous time, the Absaroka and Darby in Late Cretaceous to Paleocene times and the Prospect thrust in mid Paleocene to Early Eocene times. Estimates for displacements along individual thrust faults are taken from Royse (Reference Royse, Snoke, Steidtmann and Roberts1993). The thrust faults were cut by younger extensional faults from Neogene time onwards.

Figure 1. (a) Thin-skinned tectonic style in the Sevier fold-and-thrust belt (Wyoming). Redrawn from Royse (Reference Royse, Snoke, Steidtmann and Roberts1993; section D). Ages of thrust activity and displacements after Royse (Reference Royse, Snoke, Steidtmann and Roberts1993); numbers are approximate. Extensional faults are shown in blue. (b) Thick-skinned tectonic style in the Laramide structure (Wind River Range, Wyoming). After Smithson et al. (Reference Smithson, Brewer, Kaufman, Oliver and Hurich1978), modified.
The neighbouring Laramide structure shown in Figure 1b is a classic case of thick-skinned tectonics where the thrust fault putting the Wind River Range onto the Cenozoic basin fill of the Green River Basin reaches down to at least 24 km (Smithson et al. Reference Smithson, Brewer, Kaufman, Oliver and Hurich1978). It then levels off into parallelism with the crust–mantle boundary (Yeck et al. Reference Yeck, Sheehan, Anderson, Erslev, Miller and Siddoway2014). These authors suggest that the high of the Moho in the neighbouring Bighorn Mountain region is an old feature not linked to the thick-skinned Laramide shortening. As shown in Figure 1b, the Moho is not cut by the Wind River thrust. The Moho high drawn in Figure 1b is adapted from the topography reported by Yeck et al. (Reference Yeck, Sheehan, Anderson, Erslev, Miller and Siddoway2014). It is interesting to note that the Laramide shortening occurred contemporary with some of the shortening in the Sevier orogeny, although the vergence of thrusting was opposite in the two cases.
Generally speaking the Alps can be subdivided into three segments. The Western Alps (Italy and France) extend form Nice to Geneva and have structures trending more or less N–S. The Eastern Alps (Austria and Italy) extend from the Vienna Basin to the west to Chur. Here structures are trending more or less E–W. The Central Alps (Switzerland and Italy) between Chur and Geneva make the transition between the Western and Eastern Alps.
The Alps formed in response to the subduction and collision of the European and Adriatic continental margins. The collision was preceded by subduction of a Jurassic ocean (Liguria–Piemont Ocean), the amalgamation of a microcontinent (Briançon Swell) and a basin that formed on the thinned continental European margin interspersed with small oceanic pull-aparts (Valais Basin; Steinmann, Reference Steinmann1994; Steinmann & Stille, Reference Steinmann and Stille1999). The nappe systems that formed in the process reflect the crustal structure of these palaeogeographic realms. The Helvetic and Dauphinois nappe systems correspond to the shortened European margin; the Penninic nappe system evolved from the Valais Basin, the Briançon Swell and the Liguria–Piemont Ocean; and, finally, the Austroalpine and Southalpine nappe systems represent the shortened Adriatic margin. The tectonic map of Figure 2 displays the spatial arrangement of these nappe systems.

Figure 2. Schematic tectonic map of the Alps showing the main nappe systems and the distribution of crystalline basement rocks within the nappe systems. A-R – Aiguilles Rouges massif; M-B – Mont Blanc massif. Labels of traces of cross-sections correspond to figure numbers.
The Helvetic and Dauphinois nappe systems straddle the western and northern rim of the Alps; the Jura Mountains can be considered as a most frontal thrust sheet of this complex. The Southalpine and Austroalpine thrust systems are located on the southern margin of the Alps, but extend across the entire Alps in the Eastern Alps. Three windows, the Rechnitz, Tauern and Engadin windows, bear witness to the fact that the Penninic nappe system and the European margin extend all the way eastwards to the Vienna Basin beneath the Austroalpine nappe system. The zigzagging eastern limit of the Austroalpine north and south of Chur is an erosional front which reflects the easterly plunge of the nappe sheets of the Central Alps. The Dent Blanche klippe between Geneva and Milan along with small klippen not shown in Figure 2 testify to the fact that units derived from the Adriatic margin once covered the Penninic nappe system. The Penninic nappe system overlies the Helvetic and Dauphinois nappe system. East of Geneva and along the northern rim of the Central Alps, klippen of Penninic nappes demonstrate that these nappes once covered much of the Central Alps. The Penninic nappe system was derived from three palaeogeographic domains, the Valais Basin, the Briançon Swell and the Liguria–Piemont Ocean, which explains the differences in their internal structure.
The tectonic map of Figure 2 also shows the distribution of pre-Triassic crystalline basement rocks and Palaeozoic sediments within the different nappe systems. In the Helvetic and Dauphinois nappe systems crystalline basement crops out in the cores of antiforms that span the central part of the Western and Central Alps and include the heart of the Eastern Alps in the Tauern Window. Within the Penninic nappe system, crystalline basement crops out in the internal part of the Western and Central Alps. The Southalpine and Austroalpine nappe systems lack crystalline basement in the external part of the Alpine orogen.
The aim of this paper is to discuss the involvement of crystalline basement in the Alpine nappe structure. Apart from purely geometric aspects, the process of nappe formation is discussed in terms of the geodynamic evolution of the Alps. The study then sheds light on the influence of mechanical properties of the rock types within the nappes on the structure of these nappes.
2. Alpine nappe structure
In this section the structure of Alpine nappes is discussed in the light of the degree of involvement of crystalline basement rocks. This is based on a number of thrust sheets selected from the different nappe systems, which are taken to be typical for the question addressed.
2.a. Helvetic nappe system
The Helvetic nappe system comprises from top to bottom three major units: the Ultrahelvetic slices, the Helvetic nappes proper and the Infrahelvetic complex. The Ultrahelvetic slices are made of Cretaceous–Eocene sediments that were detached from their substratum and came to rest on the future Helvetic nappes. The Helvetic nappes proper were detached from their crystalline basement and transported over distances of up to 50 km and now overlie the Infrahelvetic complex and the Subalpine Molasse. The Helvetic nappes consist of mainly Mesozoic sediments, which are internally folded and in part dismembered into smaller thrust sheets. The Infrahelvetic complex is made up of crystalline basement (the Aar massif) with its autochthonous and parautochthonous Mesozoic and Cenozoic cover as well as some allochthonous units, which were stripped off the footwall and dragged along the basal thrust of the Helvetic nappes. The internal structure of the Helvetic nappe system undergoes profound changes along strike. Some of these changes occur across N–S-oriented wrench faults that were active during nappe formation and allowed the independent development of structures on either side of the fault. For details regarding these structures and their changes, the reader is referred to the compilation by Pfiffner et al. (Reference Pfiffner, Burkhard, Hänni, Kammer, Kligfield, Mancktelow, Menkveld, Ramsay and Zurbriggen2010) and Pfiffner (Reference Pfiffner2011), or the overview given in Pfiffner (Reference Pfiffner2014).
The youngest synorogenic sediments involved in thrusting give a minimum age of Early Eocene in the more internal, Ultrahelvetic part, and Early Oligocene in the external part (Pfiffner, Reference Pfiffner, Allen and Homewood1986; Burkhard, Reference Burkhard1988; Schmid et al. Reference Schmid, Pfiffner, Froitzheim, Schönborn and Kissling1996; Burkhard & Sommaruga, Reference Burkhard, Sommaruga, Mascle, Puigdefabregas, Luterbacher and Fernandez1998; Kempf & Pfiffner, Reference Kempf and Pfiffner2004). The trailing end of the Helvetic nappes was metamorphosed to an epizonal degree, the leading edge to a diagenetic degree only (Groshong, Pfiffner & Pringle, Reference Groshong, Pfiffner and Pringle1984; Frey & Ferreiro Mählmann, Reference Frey and Ferreiro Mählmann1999). The underlying Infrahelvetic complex reached anchizonal to epizonal conditions. The metamorphic isograds cross-cut the internal structure of the nappe system, but are offset along the basal thrust of the Helvetic nappes (op. cit.). This metamorphism is dated as Oligocene to Miocene (see Frey & Ferreiro Mählmann, Reference Frey and Ferreiro Mählmann1999 and references therein). Combined with the stratigraphic data it points to a northward encroachment of the Helvetic nappe system onto the subsiding foreland (see also Pfiffner, Reference Pfiffner2014 and references therein).
The Helvetic nappes represent a classic thin-skinned style with Mesozoic sediments detached from their pre-Triassic crystalline substratum. Figure 3 shows a cross-section through central Switzerland highlighting the typical structures of the Helvetic nappe system. The cross-section is based on J.-W. Menkveld (unpub. Ph.D. thesis, Univ. Bern, 1995), Hänni & Pfiffner (Reference Hänni and Pfiffner2014) and Pfiffner & Deichmann (Reference Pfiffner and Deichmann2014). In the case of the Axen thrust shown in Figure 3, decollement occurred in Triassic evaporites, Lower Jurassic shales and Middle Jurassic shales, whereby the thrust fault climbed in a stepwise fashion to successively higher levels in the direction of transport. Within the Axen nappe south of Gross Walenstock in Figure 3, the relatively thick Lower and Middle Jurassic strata led to the development of detachment folds. These mechanically weak strata filled the cores of anticlines that developed in the mechanically strong Upper Jurassic limestones. Beneath and north of Gross Walenstock the Upper Jurassic limestones form an imbricate stack; here the mechanically weak layers are thin such that the formation of detachment folds was inhibited. The causal relationship between the thickness of detachment horizons and the formation of detachment folds and/or thrust faults is discussed in Pfiffner (Reference Pfiffner1993). This study suggests that the ratio, n, of the thickness of mechanically weak to the thickness of mechanically strong layers is of importance regarding the style of a fold-and-thrust belt. A low ratio favours harmonic folding and/or imbricate thrusting, whereas ratios greater than 0.5 lead to disharmonic folding and the formation of detachment folds.
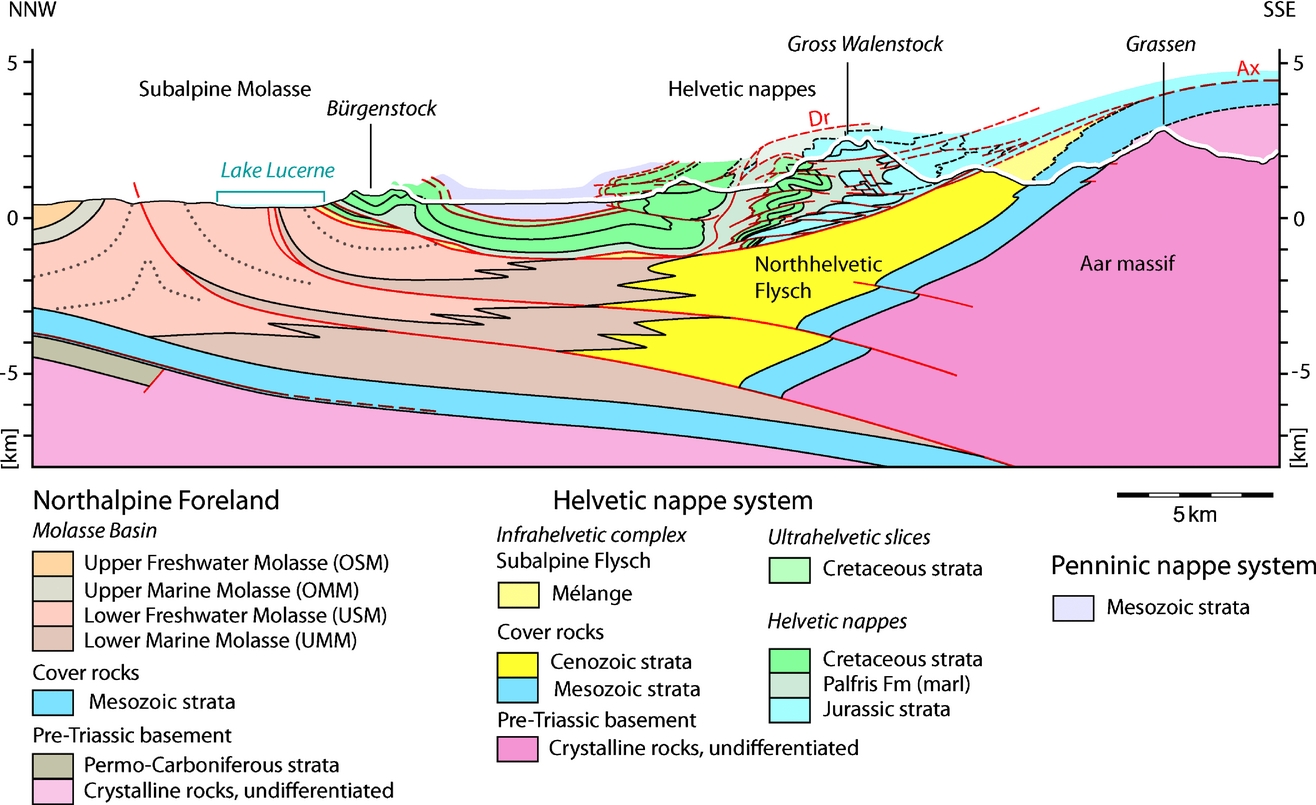
Figure 3. Cross-section of the Helvetic nappe system and the Subalpine Molasse in central Switzerland. See text for discussion. Trace of cross-section is given in Figure 2. Ax – Axen thrust; Dr – Drusberg thrust.
As evident in the cross-section of Figure 3, the Cretaceous strata extend much further to the north owing to differential movement along the Drusberg thrust, which followed the decollement horizon located in the marls of the Palfris Formation. Within the Drusberg nappe, the Cretaceous limestones above the decollement form detachment folds south of Bürgenstock and north of Gross Walenstock. The fact that the folds in the Axen and Drusberg nappe are disharmonic suggests that the decollement of the Cretaceous strata occurred at the very onset of nappe formation. The decoupling was controlled by the greater thickness of the Palfris Formation on the subsiding European margin in the south of the Helvetic realm. In the Axen nappe, Cretaceous strata are folded just north of Gross Walenstock; these folds change from detachment fold style to imbricate thrusting as the Palfris Formation tapers off towards the north.
The question that arises is which crystalline basement formed the original substratum of these Helvetic nappes. Since the underlying Aar massif retained its autochthonous cover this basement is to be sought further to the south (Trümpy, Reference Trümpy1969; Kempf & Pfiffner, Reference Kempf and Pfiffner2004). In fact, as evident on geological maps, on the southernmost Aar massif, the Tavetsch massif and the Gotthard massif the autochthonous Mesozoic cover is missing except for lenses of Triassic strata that now delimit these basement blocks.
Inspection of Figure 3 reveals that the Aar massif is updomed in the hanging wall of a thrust fault that puts the Aar massif onto the autochthonous foreland. This bulging is a late feature in the structural evolution and was responsible for the passive updoming of the Axen thrust. The thick-skinned nature of this structure will be discussed later in the framework of the entire Alpine orogen.
North of and beneath the Drusberg nappe the Molasse sediments exhibit an imbricate structure giving way to an antiformal structure north of Lake Lucerne. This shortened package of Molasse sediments that is overridden by the Helvetic nappes is called Subalpine Molasse in the literature. The clastic sequence involved was deposited in a foredeep that formed during nappe stacking in the ancestral Alps. The decollement of the thrust sheets occurred in a thick Oligocene mudstone sequence (the so-called Lower Marine Molasse, UMM). The northernmost thrust fault within the Subalpine Molasse is tentatively linked to the basal thrust fault of the Aar massif in Figure 3. The structure shown at the transition of the Northhelvetic Flysch to the Subalpine Molasse in Figure 3 is speculative. It can't be studied at outcrop, has not been drilled and the available reflection seismic lines (Pfiffner, Sahli & Stäuble, Reference Pfiffner, Sahli, Stäuble, Pfiffner, Lehner, Heitzmann, Mueller and Steck1997) are not conclusive for this particular question.
In the Western Alps, Boutoux et al. (Reference Boutoux, Bellahsen, Lacombe, Verlaguet and Mouthereau2014) reported that the updoming of the external massifs was strongly controlled by the Jurassic rift basins. Deformation within the crystalline basement involved localized shear zones that link to tight folds in the sedimentary cover above. According to these authors, Jurassic rifting weakened the crust and concentrated deformation during the Alpine orogenesis.
2.b Penninic nappe system
The Penninic nappe system, located in the very core of the Alpine chain, is by far the most complex of all. Generally speaking, three types of nappes may be distinguished based on the rock suites involved: crystalline basement nappes, sediment nappes and ophiolite nappes. As will be shown, a clear distinction is not always possible.
Crystalline basement nappes consist of polymetamorphic gneisses interspersed with amphibolites and late- to post-Variscan granitoids now preserved as orthogneisses. Sediments are rare and usually consist of Triassic quartzites and carbonates that occur as thin lenses traceable over long distances. They allow for the separation of the various crystalline basement nappes (they are referred to as ‘nappe separators’ in early Alpine literature). The sediment nappes display mostly a very complex geometry owing to a multiphase deformation (including post-nappe folding). Ophiolite nappes emanating from the ocean basins that opened in the Jurassic–Cretaceous period crop out as lenses several kilometres long and are typically associated with deep-water sediments.
The Penninic nappe system attained a much higher degree of metamorphism compared to the Helvetic nappe system discussed in Section 2.a above. In the Lepontine area of the Central Alps upper-amphibolite grade is reached. The isograds of this Barrovian-type metamorphism cross-cut the nappe boundaries. Peak temperatures were reached in latest Eocene time (between 35 and 30 Ma) most likely (see discussion in Frey & Ferreiro Mählmann, Reference Frey and Ferreiro Mählmann1999). It is important to note that this regional metamorphism was preceded by a high-pressure event in Late Eocene time from 45 to 35 Ma (see e.g. Rosenbaum & Lister, Reference Rosenbaum and Lister2005). It is important to note though that, considering the entire Alpine orogeny, the high-pressure metamorphism is diachronous. It is of mid Cretaceous age (~100 Ma) in the Eastern Alps and latest Cretaceous (70 Ma) to Palaeogene (50 to 35 Ma) in the Western and Central Alps (see metamorphic map by Oberhänsli et al. Reference Oberhänsli, Bousquet, Engi, Goffé, Gosso, Handy, Höck, Keller, Lardeaux, Polino, Rossi, Schuster, Schwartz and Spalla2004).
The structural evolution is characterized by multiphase thrusting and folding. Deformation phases as determined from structural analyses vary from area to area and it is often very difficult to correlate from one study area to the next. Such correlations are also hampered by the fact that deformation phases are defined by map patterns in some instances and by microstructures in other cases. Moreover, some authors put emphasis on folds (e.g. Steck et al. Reference Steck, Della Torre, Keller, Pfeifer, Hunziker and Masson2013) while others emphasize the importance of thrusting in nappe stacking (e.g. Manzotti et al. Reference Manzotti, Ballèvre, Zucali, Robyr and Engi2014). The tectonic evolution of the Penninic nappes of the Central Alps is discussed in more detail in Schmid et al. (Reference Schmid, Pfiffner, Froitzheim, Schönborn and Kissling1996) and Scheiber, Pfiffner & Schreurs (Reference Scheiber, Pfiffner and Schreurs2012) for the eastern part, and in Burkhard & Sommaruga (Reference Burkhard, Sommaruga, Mascle, Puigdefabregas, Luterbacher and Fernandez1998), Scheiber, Pfiffner & Schreurs (Reference Scheiber, Pfiffner and Schreurs2013) and Steck, Masson & Robyr (Reference Steck, Masson and Robyr2015) for the western part. A larger scale compilation of the structural syntheses and the timing of orogenic events for the Eastern, Central and Western Alps is given in Pfiffner (Reference Pfiffner2014). This compilation reveals that generally speaking the detachment of sedimentary cover nappes occurred in an early stage and was followed by thick-skinned tectonics involving the crystalline basement. For the Penninic nappe system, nappe stacking commenced in mid Eocene times and post-nappe folding lasted into Early Miocene time.
In the following, priority is given to the basement nappes within the Penninic nappe system as these relate rather directly to the topic of thin- and thick-skinned tectonics.
2.b.1. Suretta nappe
The first example, the Suretta nappe, crops out in the eastern Central Alps and is discussed using the cross-section shown in Figure 4a. The cross-section is based on the work of Scheiber, Pfiffner & Schreurs (Reference Scheiber, Pfiffner and Schreurs2012), Schreurs (Reference Schreurs1995) and Milnes & Schmutz (Reference Milnes and Schmutz1978). It shows the internal structure of this nappe, which is characterized by intensive back-folding that affected the upper part of the nappe. Remnants of autochthonous cover (Triassic quartzites and marbles) are preserved in the upper part of the nappe but extend deep into the crystalline basement and outline nearly isoclinal folds. The contact with the sediments beneath the Suretta nappe, the Schams nappe in the north, the Andossi slice in the south (see Fig. 4a), is clearly of a tectonic nature. The crystalline basement of the Suretta nappe contains a Permian intrusive complex (Rofna Porphyry complex) that is restricted to the northern part of the nappe. A major thrust fault outlined by Triassic sediments splits the frontal part of the nappe into two units (Fig. 4a).

Figure 4. Cross-sections of the Penninic basement nappes in eastern Switzerland. See text for discussion. (a) Suretta nappe. (b) Tambo nappe. (c) Adula nappe system. Traces of cross-sections are given in Figure 2.
The contact of the autochthonous cover in the upper unit of the nappe with the overlying nappes (Schams nappe and Avers nappe; see Fig. 4a) is of a thrust nature, and the thrust fault is clearly affected by the nappe-internal folds of the Suretta nappe (Milnes & Schmutz, Reference Milnes and Schmutz1978). It may hence be concluded that the younger Mesozoic cover of the Suretta nappe was removed and replaced by an allochthonous cover of more internal origin (Piemont Ocean in the case of the Avers nappe).
The Schams nappe that wraps around the Suretta nappe at the front consists of a sequence of Mesozoic sediments that was deposited on the Briançon Swell. These rocks were detached from their crystalline substratum in an early stage: the subduction phase of the Cenozoic Alpine orogeny in Middle Eocene time. As discussed by Schmid, Rück & Schreurs (Reference Schmid, Rück, Schreurs, Roure, Heitzmann and Polino1990), the crystalline substratum of the Schams nappe is to be sought in the Suretta and Tambo nappes. These basement nappes were later forced into the Schams nappe in a later phase of subduction in Late Eocene time, and led to the formation of the back-folds in the Suretta nappe.
2.b.2. Tambo nappe
The second example of Penninic basement nappes is the Tambo nappe, for which the northern part is shown in the cross-section of Figure 4b. The cross-section is based on the work of Mayerat-Demarne (Reference Mayerat-Demarne1994). On top of the Tambo nappe small remnants of Triassic quartzites is all that remains of the autochthonous cover. The Triassic marbles and cargneules (altered gypsum-bearing evaporites) of the Andossi slice located between the Tambo and Suretta nappes represent an allochthonous sequence that has been scraped off the southern part of the Tambo nappe and piled up as imbricate slices.
The Knorren mélange at the front of the Tambo nappe is a chaotic mix of lenses of boulders of metabasalts, gneisses, polymict breccias (containing gneisses), quartzites, marbles, dolostones, evaporites and calcareous sandstones embedded in a matrix of black and green slates, sandstones and marble. This mélange could have formed during Jurassic normal faulting or in the course of Alpine nappe stacking, or as a combination of the two processes (Mayerat-Demarne, Reference Mayerat-Demarne1994).
A particularly interesting feature is the intricately folded ‘sandwich’ to the north of the Knorren mélange. Here Mesozoic sediments pertaining to the Schams nappe are sandwiched between two thin basement flakes. The contact between the sediments and the basement is of a tectonic nature. But it remains so far unclear if it is related to the formation of extensional allochthons during Jurassic normal faulting, Alpine nappe stacking or both. The contact with the sediments of the Tomül nappe, however, is clearly a thrust fault. Therefore, the tight folding of the ‘sandwich’ represents post-nappe folding. It may be correlated to the back-folding phase which affected the Suretta nappe as discussed above.
2.b.3. Adula nappe complex
The third example, the Adula nappe complex, underlies the Tambo nappe and has a far more complex internal structure that is characterized by a manifold repetition of slabs of ortho- and paragneisses (Berger & Mercolli, Reference Berger and Mercolli2006; Cavargna-Sani et al. Reference Cavargna-Sani, Epard, Bussy and Ulianov2014 a; Pleuger & Podladchikov, Reference Pleuger and Podladchikov2014). As pointed out by Nagel (Reference Nagel, Siegesmund, Fügenschuh and Froitzheim2008), the gneisses of the Adula nappe complex show a mylonitic Alpine foliation in comparison to the more moderately deformed gneisses of the Simano nappe beneath and the Tambo nappe above. The cross-section shown in Figure 4c is based on a horizontal section provided by Löw (Reference Löw1987). The advantage of using a horizontal section is the relatively short projection distances of the structural features observed at outcrop. The horizontal section was then telescoped in the direction of the general ENE-directed plunge of the large-scale fold axes in order to obtain a cross-sectional geometry as one would obtain in a vertical cross-section. The plunge of the structures is corroborated by the reflection seismic data of NRP20 acquired 15 to 50 km east of the Adula outcrops (see Pfiffner & Hitz, Reference Pfiffner, Hitz, Pfiffner, Lehner, Heitzmann, Mueller and Steck1997).
The general structure of the front of the Adula nappe complex with panels of orthogneisses interlayered with polymetamorphic paragneisses was projected into the cross-section of Figure 4c using the horizontal section of Löw (Reference Löw1987), the tectonometamorphic map of Berger & Mercolli (Reference Berger and Mercolli2006) and the map by Cavargna-Sani et al. (Reference Cavargna-Sani, Epard, Bussy and Ulianov2014 a). Five major orthogneiss complexes are recognized: Zervreila, Gana-Palingera, Groven, Argio and Garenstock. Their contacts with the paragneisses are in part very complex, and owing to the intensive Alpine overprint these orthogneiss complexes cannot be interpreted in terms of intrusive bodies. Cavargna-Sani et al. (Reference Cavargna-Sani, Epard, Bussy and Ulianov2014 a) combined the Groven and Argio complexes into one complex (Rossa Orthogneiss) and consider Gana-Palingera to be part of Zervreila. They were able to date the Garenstock and combined Groven and Argio complexes as Ordovician (Garenstock to 457–446 Ma; Groven-Argio to 459–456 Ma), whereas the Zervreila complex is of Early Permian age (297–288 Ma). These authors were also able to further subdivide the paragneisses (see Fig. 4) into formations and to date these. The Salahorn Formation and Heinisch Stafel Formation, two sequences of metaclastic and magmatic rocks, were dated to be of Cambrian and Ordovician age, respectively. The Trescolmen Formation is made of metapelites containing mafic lenses and is of Palaeozoic age.
A peculiar feature contained within the paragneiss panels are the lenses of marble, as well as lenses of (partly eclogitic) amphibolites within metapelites, which are lined up forming strings of pearls in the roof of the orthogneiss complexes. The repartition of these lenses within the Adula nappe complex shown in the cross-section is based on the map by Berger & Mercolli (Reference Berger and Mercolli2006). In some instances, groups of marble and amphibolite lenses occur side by side. Cavargna-Sani et al. (Reference Cavargna-Sani, Epard, Bussy and Ulianov2014 a) dated one lens to be of Ordovician age. The carbonate lenses are in part possibly of Palaeozoic age (Cavargna-Sani et al. Reference Cavargna-Sani, Epard, Bussy and Ulianov2014 a), but most of them are likely to be of Mesozoic age (Nagel et al. Reference Nagel, De Capitani, Frey, Froitzheim, Stünitz and Schmid2002; Berger & Mercolli, Reference Berger and Mercolli2006; Cavargna-Sani, Epard & Steck, Reference Cavargna-Sani, Epard and Steck2014). Cavargna-Sani et al. (Reference Cavargna-Sani, Epard, Bussy and Ulianov2014 a) suspected that the Trescolmen Formation with its Palaeozoic mafic lenses represents a tectonic mélange related to terrane accretion or subduction. But it has to be noted that in the case of the Fanella and Trescolmen units the lenses straddle the boundary between Palaeozoic paragneisses above and post-Variscan orthogneisses below. Similarly, the Cambrian Salahorn Formation overlies the Ordovician orthogneisses of the Groven–Argio complex in the case of the Soazza unit. The contacts marked Fanella, Trescolmen and Soazza in Figure 4c extend over 10 and more kilometres towards the south, retaining the nature of putting older rocks onto younger rocks. Of particular interest is the presence of Triassic carbonate lenses along these contacts, which suggests that these contacts are thrust faults that formed in an early stage of nappe stacking (Zapport Phase; see e.g. Cavargna-Sani, Epard & Steck, Reference Cavargna-Sani, Epard and Steck2014). It may well be that parts of the mafic lenses next to the carbonate lenses are also of Mesozoic age and represent remnants of ophiolites related to the Valais Basin.
As Cavargna-Sani, Epard & Steck (Reference Cavargna-Sani, Epard and Steck2014) have shown, intensive shearing and folding characterize the internal structure of the northern Adula nappe. This post-nappe folding affected the internal structure of the northern Adula nappe profoundly. Nevertheless, an early imbrication cannot be ruled out (Pleuger & Podladchikov, Reference Pleuger and Podladchikov2014) and is also supported by the structure of the southern Adula nappe and the adjacent Southern Steep Belt where Engi, Berger & Roselle (Reference Engi, Berger and Roselle2001) reported the existence of a mélange including lenses of mantle material; these authors explained this structure as having formed in a tectonic accretion channel (TAC) along the Eocene plate boundary (‘Alpine lithospheric mélange’ of Trommsdorff, Reference Trommsdorff1990).
The Mesozoic cover sediments of the basement slivers now forming the Adula nappe complex have been detached to form a nappe pile of their own. These cover sediments now form the Grava, Terri-Lunschania, Aul, Tomül, and the Upper and Lower Vals imbricates shown in Figure 4c. This nappe pile is affected by significant post-nappe folding as indicated by isoclinally folded thrust faults (e.g. Probst, Reference Probst1980; Wiederkehr et al. Reference Wiederkehr, Bousquet, Schmid and Berger2008). The Aul and Tomül imbricates contain Mesozoic ophiolites (Tomül at the base only).
2.b.4. Bernard nappe complex
The fourth example of Penninic basement nappes discussed is the Bernard nappe complex of the western Central Alps (Fig. 5). The structure of this nappe complex has been elaborated on by generations of geologists (see Scheiber, Pfiffner & Schreurs, Reference Scheiber, Pfiffner and Schreurs2013 and references therein). The Bernard nappe complex is subdivided into a lower unit, the Siviez–Mischabel nappe, and an upper unit, the Mont Fort nappe. It is presented using two cross-sections based on the work by Scheiber, Pfiffner & Schreurs (Reference Scheiber, Pfiffner and Schreurs2013). The eastern cross-section (Fig. 5a) contains the lower unit only: the Siviez–Mischabel overlying the Mesozoic carbonates of the Zone Houillère (formerly attributed to the Pontis nappe, which was also considered part of the Bernard nappe complex). The Siviez–Mischabel nappe has a core made of pre-Triassic basement rocks (mainly Palaeozoic paragneisses), which is overlain by an autochthonous cover of Permo-Triassic and Triassic quartzites and Jurassic carbonates and shales. A thick sequence of mainly quartzites can be found beneath the crystalline basement rocks. Because of the seemingly symmetric structure with an upper and lower limb of sediments on either side of the crystalline basement, the Siviez–Mischabel nappe was interpreted to represent a large-scale recumbent fold (e.g. Argand, Reference Argand1916; Escher, Masson & Steck, Reference Escher, Masson and Steck1993; a detailed discussion is given in Scheiber, Pfiffner & Schreurs, Reference Scheiber, Pfiffner and Schreurs2013). However, as Markley, Teyssier & Caby (Reference Markley, Teyssier and Caby1999) and Scheiber, Pfiffner & Schreurs (Reference Scheiber, Pfiffner and Schreurs2013) have shown, the sedimentary sequences beneath the crystalline basement represent a stack of imbricate thrust sheets where the sediments are in an upright position (as indicated by the arrows in Fig. 5). They are interpreted as having been detached from their crystalline substratum, piled up and subsequently overridden by their former substratum. Several lenses of Permian metasediments are found within the quartzites and the basement and point to pervasive internal imbrications and shearing. The basal thrust of the Tsaté nappe is folded harmonically with the folds in the cover of the Siviez–Mischabel nappe, which proves that the emplacement of the Tsaté nappe occurred in an early stage of nappe stacking. The Tsaté nappe is an upper Penninic unit derived from the Piemont Ocean (e.g. Sartori, Gouffon & Marthaler, Reference Sartori, Gouffon and Marthaler2006), and the overlying Dent Blanche nappe consists of crystalline basement attributed to the Adriatic margin, i.e. to the Southalpine nappe system (cf. Manzotti et al. Reference Manzotti, Ballèvre, Zucali, Robyr and Engi2014).

Figure 5. Cross-sections of the Bernard nappe complex in western Switzerland. See text for discussion. (a) Eastern section (Val d'Anniviers). (b) Western section (Val d'Hérémence). Traces of cross-sections are given in Figure 2.
The cross-section shown in Figure 5b looks rather different even though it is located only about 23 km further to the WSW. Here the Bernard nappe complex is represented by an additional nappe, the Mont Fort nappe. Both, the Siviez–Mischabel and the Mont Fort nappe contain a thick sequence of Permian metasediments. It is in fact the termination of these Permian basins (graben structures) that causes the change in the internal architecture (see Scheiber, Pfiffner & Schreurs, Reference Scheiber, Pfiffner and Schreurs2013 for a more detailed discussion). The Permian metasediments are in a stratigraphically inverted position owing to the inversion of the Permian graben. Nevertheless, the crystalline basement of the Siviez–Mischabel has an autochthonous cover of Permian and Triassic quartzites, whereas the younger Mesozoic cover was detached and can now be found in the Klippen nappe in the more external part of the Alps (Ellenberger, Reference Ellenberger1952; Sartori, Reference Sartori1987; Stampfli, Reference Stampfli1993; Sartori, Gouffon & Marthaler, Reference Sartori, Gouffon and Marthaler2006). The contact with the Permian metasediments below this crystalline basement is of a thrust nature (Scheiber, Pfiffner & Schreurs, Reference Scheiber, Pfiffner and Schreurs2013). In the Mont Fort nappe, a thin synform of the Tsaté nappe pinched between the Permian metasediments points to intensive folding and shearing of the Permian. Stratigraphic younging directions suggest the presence of an anticline to the north of the synform. The contact with the crystalline basement of the upper part of the Mont Fort nappe is of a thrust nature again, but the Permian beneath has been overturned in the course of basin inversion.
All in all, the western cross-section indicates that the Mesozoic sequence of the Briançon Swell was detached along the Triassic evaporites (now present as cargneules). The presence of lateral mechanical discontinuities provided by Permian graben structures could explain the contrasting styles in the western and eastern cross-sections of Figure 5 (Scheiber, Pfiffner & Schreurs, Reference Scheiber, Pfiffner and Schreurs2013); moreover, the large-scale folds in the western section may be attributed to inversion of the Permian graben structures.
2.c. Austroalpine–Southalpine nappe systems
The Austroalpine and Southalpine nappe systems are a heterogeneous structural assembly of nappes consisting of crystalline basement, Mesozoic sediments or both. Their common feature is the palaeogeographic attribution to the Adriatic continental margin. Two examples illustrating the involvement of crystalline basement in the Alpine nappe structure will be discussed in more detail.
2.c.1 Silvretta–Sesvenna–Campo nappe complex
The Austroalpine nappe system in the Eastern Alps forms an orogenic lid covering the underlying Penninic nappe system, which crops out in three windows: the Engadin, Tauern and Rechnitz windows (see Fig. 2). The cross-section given in Figure 6 passes through the westernmost window, the Engadin Window. It illustrates the internal structure of the largest crystalline basement nappe, the Silvretta–Sesvenna–Campo nappe system, measuring about 100 km in a NW–SE direction. The shallow part of the cross-section is based on Oberhauser (Reference Oberhauser1998) in the northwest and Schmid (Reference Schmid1973) and Schmid & Haas (Reference Schmid and Haas1989) in the central part. The deep structure from the Engadin Window to the northwest is constrained by reflection seismic data (Hitz, Reference Hitz1996; Pfiffner & Hitz, Reference Pfiffner, Hitz, Pfiffner, Lehner, Heitzmann, Mueller and Steck1997).

Figure 6. Cross-section of the Austroalpine nappes of western Austria. See text for discussion. Inset shows normal faulting in the cover of the Sesvenna basement related to the Schlinig thrust at the base of the Ötztal nappe (redrawn from Schmid & Haas, Reference Schmid and Haas1989). Trace of cross-section is given in Figure 2.
To the northwest, the Silvretta basement is overthrust onto the Northern Calcareous Alps, and, according to Oberhauser (Reference Oberhauser1998), the basal thrusts of both the Silvretta basement as well as the Northern Calcareous Alps are affected by folds and thrust faults emanating from the Penninic nappe system in its footwall. The internal structure of the Penninic nappe system is not shown in the cross-section, but considering cross-sections further to the west (Schmid et al. Reference Schmid, Pfiffner, Froitzheim, Schönborn and Kissling1996; Pfiffner & Hitz, Reference Pfiffner, Hitz, Pfiffner, Lehner, Heitzmann, Mueller and Steck1997), where continuous surface outcrops and reflection seismics constrain the internal structure of the Penninic nappe system, displacements along individual thrust faults between nappes are much higher (tens of kilometres) compared to the displacements of the thrust faults that offset at the base of the Austroalpine nappe system. This situation suggests the existence of an ‘orogenic lid’ made up of Austroalpine nappes in the hanging wall of the Penninic nappes in the course of their formation.
In the central part, the autochthonous sedimentary cover of the Sesvenna basement is preserved (Schmid, Reference Schmid1973), folded and partly scraped off by the emplacement of the Ötztal nappe along the Schlinig thrust in a westerly direction in Cretaceous time (Schmid & Haas, Reference Schmid and Haas1989). Scraping off of the sediments in the footwall of the thrust from the Sesvenna basement involved low-angle normal faults associated with a westerly motion of the upper part of the sedimentary cover. The inset in Figure 6 is redrawn from Schmid & Haas (Reference Schmid and Haas1989) and displays the structure in the footwall of the Schlinig thrust. According to these authors, the cover rocks of the Sesvenna basement were horizontally stretched by low-angle normal faults during the emplacement of the Ötztal basement. Towards the east, the Schlinig thrust widens into a thick ductile shear zone (Schmid & Haas, Reference Schmid and Haas1989) indicating that the displacement along the thrust faults within the basement was dissipated by these shear zones. This contrasts with the sharp thrust contacts in the Penninic basement nappes discussed in the previous Section 2.b.
If the Ötztal nappe clearly overlies the Sesvenna nappe, the contacts between the Sesvenna and Campo basement are more difficult to interpret in terms of thrust sheets. As discussed by Schmid (Reference Schmid1973), the various tectonic units are not sheets but rather blocks, which moved in different directions, and namely, the detached sedimentary cover sequences were intricately imbricated in a manner not shown in the cross-section of Figure 6. The ‘double nature’ of faults – strike-slip and thrust faults – is particularly highlighted by the Gallo and Braulio faults shown in Figure 6. The Gallo fault, nearly vertical in the cross-section of Figure 6, becomes a more shallow E-dipping thrust fault a few kilometres to the northeast (Schmid, Reference Schmid1973). The Braulio fault, NW-dipping in the cross-section of Figure 6, undergoes strike changes going west and east and rejoins the Gallo fault at some stage (Schmid, Reference Schmid1973).
The Campo basement and its Triassic cover in the Ortler nappe in the south rise towards the southeast. Just northwest of the Insubric fault the Campo basement forms an antiform with a steep southern limb and disappears in the subsurface. This antiform formed in response to strike-slip and reverse faulting along the Insubric fault.
2.c.2 Southalpine nappe complex
In the Southalpine nappe complex S-vergent thrusting created a thick stack of basement nappes. The sedimentary cover of these nappes remained largely attached to the crystalline substratum. The cross-section shown in Figure 7 is based on reflection seismic data of NRP20, redrawn and modified from Schumacher (Reference Schumacher, Pfiffner, Lehner, Heitzmann, Mueller and Steck1997). For all the units thrust faults formed a ramp from the crystalline basement up across the Mesozoic in their rear (northern) parts such that in the vicinity of the Insubric fault we observe a stack of basement pieces, whereas to the south, beneath the Po Basin we observe a stack consisting of Mesozoic cover rocks.
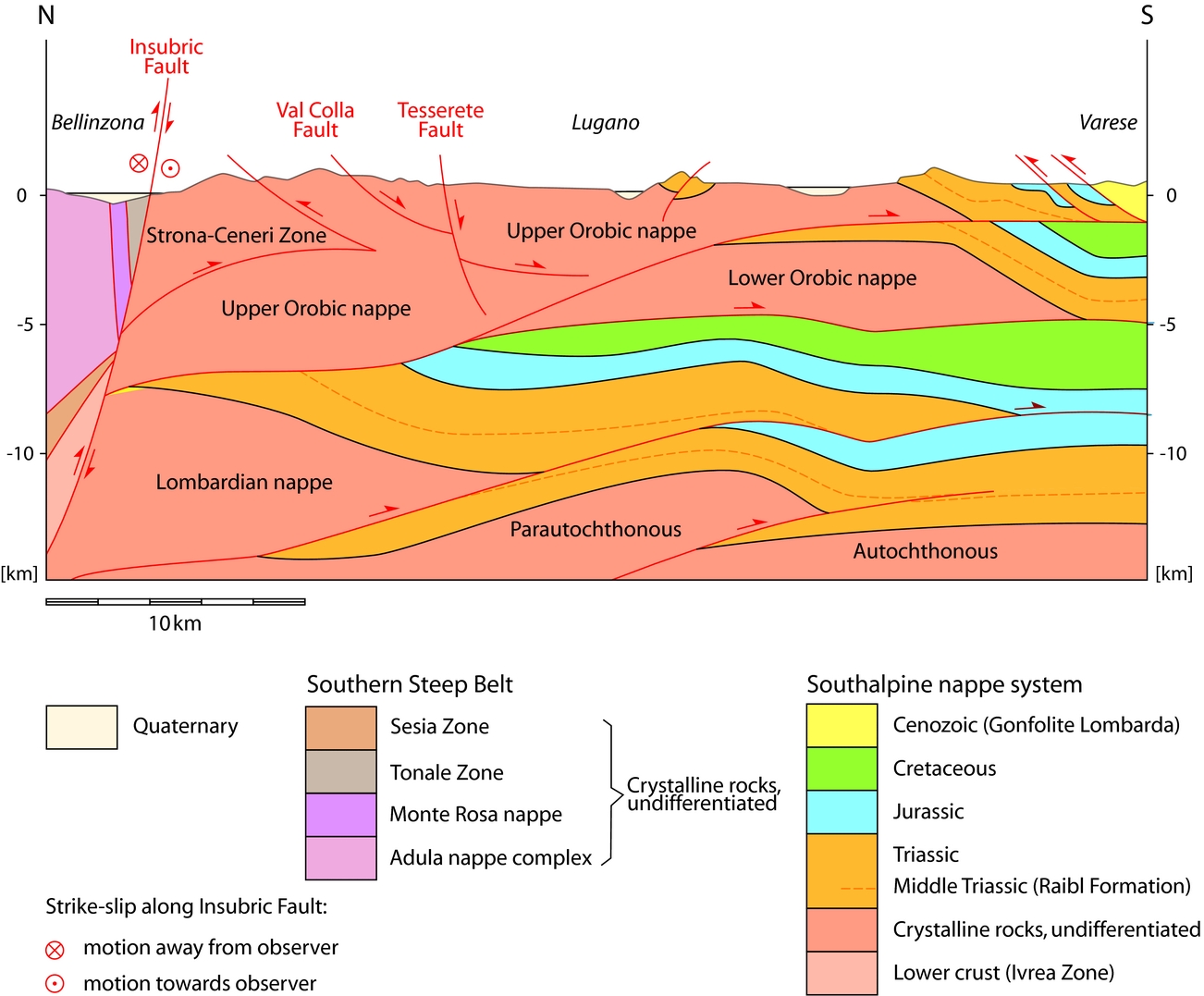
Figure 7. Cross-section through the Southalpine Orobic nappes in southern Switzerland (modified from Schumacher, Reference Schumacher, Pfiffner, Lehner, Heitzmann, Mueller and Steck1997). See text for discussion. Trace of cross-section is given in Figure 2.
In case of the Strona–Ceneri Zone (see Fig. 7) two thrust faults of opposing vergence indicate that this basement was jammed into the basement of the Upper Orobic nappe. Similarly, at the southern front of the Upper Orobic nappe the Mesozoic carbonates were wedged into the sediments underlying the Po Basin as evidenced by the N-vergent thrust faults.
3. Crustal structure
Apart from the detached upper crustal thrust sheets discussed in the preceding Section 2, new geophysical data give insight into how the lower part of the crust was affected by the Alpine collision event. Figure 8 shows two crustal sections across the Western and Central Alps. The lithologic contacts and thrust faults shown in the upper crust are based on geological surface data, structural considerations and interpretation of the reflection seismic lines of ECORS/CROP (Schmid & Kissling, Reference Schmid and Kissling2000) and NRP20 (Schmid et al. Reference Schmid, Pfiffner, Froitzheim, Schönborn and Kissling1996; Pfiffner & Hitz, Reference Pfiffner, Hitz, Pfiffner, Lehner, Heitzmann, Mueller and Steck1997; Pfiffner, Reference Pfiffner2014).

Figure 8. Cross-sections showing crustal structure of the Alps. See text for discussion. (a) Western Alps. (b) Central Alps. Traces of cross-sections are given in Figure 2.
The crustal structure shown in Figure 8 is based on controlled-source seismology (CSS) combined with local earthquake tomography (LET) (Diehl et al. Reference Diehl, Husen, Kissling and Deichmann2009; Wagner, Kissling & Husen, Reference Wagner, Kissling and Husen2012). To highlight the crustal structure, the P-wave velocity contour of 6.5 km s−1 and the crust–mantle boundary (Moho) was chosen. The vP = 6.5 km s−1 velocity contour is taken as a proxy for the interface between upper and lower crust, lower crust having P-wave velocities ≥ 6.5 km s−1 based on CSS data (Ansorge, Blundell & Mueller, Reference Ansorge, Blundell, Mueller, Blundell, Freeman and Mueller1992). It has to be mentioned though that the resolution of the LET data is not homogeneous throughout a cross-section (Diehl et al. Reference Diehl, Husen, Kissling and Deichmann2009). Also, there are some discrepancies between CSS and LET data regarding the position of the interface between the upper and lower crust (Conrad Discontinuity) derived from CSS data (Ansorge, Blundell & Mueller, Reference Ansorge, Blundell, Mueller, Blundell, Freeman and Mueller1992) and the vP = 6.5 km s−1 velocity contour. This leaves some room for interpretation for the position of the Conrad Discontinuity. In the cross-sections of Figure 8, the vP = 6.5 km s−1 contour was taken to represent this discontinuity, a first-order approximation also adapted by Rosenberg & Kissling (Reference Rosenberg and Kissling2013). Velocity contours for vP < 6.5 km s−1 derived from LET data show a complex pattern within the upper crust which cannot be correlated with geological structures as derived from reflection seismics and are thus not considered in this study. Similarly, contours at the vP > 7 km s−1 located in the lithospheric mantle also exhibit a complex pattern that cannot be interpreted and thus are also not considered for this study. However, the vP = 7 km s−1 contour derived from LET data follows closely the Moho determined from reprocessed CSS data as discussed by Diehl et al. (Reference Diehl, Husen, Kissling and Deichmann2009) and Wagner, Kissling & Husen (Reference Wagner, Kissling and Husen2012).
In the Western Alps (Fig. 8a), the Conrad Discontinuity between the upper and lower European crust has a wavy shape, whereas the underlying Moho is more linear except for the southernmost segment. This contrast indicates that the lower European crust has been substantially deformed. A direct linkage between the shape of the Conrad Discontinuity and the structure displayed by the top surface of the crystalline basement is not possible, suggesting an independent deformation between lower and upper crust. Considering the resolution of the LET data it remains open if the antiform south of Belledonne is of a thrust nature or a pure fold. On the Adriatic side, the mantle lithosphere rises to a shallow level and the overlying Adriatic lower crust almost reaches the Earth's surface (in fact it is exposed in the Ivrea Zone 15 km to the NE of the cross-section). The entire shape of the lower Adriatic crust clearly shows a thick-skinned tectonic style.
In the Central Alps (Fig. 8b), the European Moho dips more steeply to the southeast beneath the Adriatic Moho. The gap between the two Mohos is real, but the continuation of the European Moho beneath the gap is not supported by any data (and thus dashed in Fig. 8b). Interestingly there are two upper crustal units (Aar and Gotthard massifs beneath and south of Chur, respectively) that should likely have lower crustal equivalents, which are expected in the Moho gap and its extension to the southeast at depths in excess of 50 km. It could be argued that this lower crust is metamorphosed and that the resulting higher density and vP velocities would thus render it undistinguishable from mantle lithosphere by geophysical methods. The depth of the thrust fault on which the Aar massif was detached from its lower crust was determined by balancing the bed length of the top of basement and the area of structural relief (Pfiffner & Hitz, Reference Pfiffner, Hitz, Pfiffner, Lehner, Heitzmann, Mueller and Steck1997). This estimate yields a depth of 12 km beneath the basement top, which makes it plausible to connect the detachment to the plate boundary at depth as shown in Figure 8b.
Contrary to the European crust, the Adriatic crust shows an intricate pattern of deformation, with the Conrad Discontinuity forming two succinct highs beneath Chiavenna and Bergamo. Again it remains speculative if these highs represent fold or thrust structures, and a structural linkage between these two and the shape of the top of the crystalline basement is not possible.
An alternative interpretation of this cross-section is given in Rosenberg & Kissling (Reference Rosenberg and Kissling2013). The main difference between the two interpretations lies in the structure of the lower crust of the European and Adriatic plates. Rosenberg & Kissling (Reference Rosenberg and Kissling2013) preferred a smooth top for the lower crust, following the vP = 6.5 km s−1 contour rather loosely, whereas in Figure 8b the top of the lower crust follows this contour strictly. As a consequence, the tip of the Adriatic lower crust in Figure 8b is shown as being thickened, its volume balancing the volume of the upper crust that is thickened by thrust faults. Rosenberg & Kissling (Reference Rosenberg and Kissling2013) showed the European lower crust as being thickened beneath the tip of the Adriatic lower crust. In both interpretations there is a disharmony between upper and lower crust, suggesting a decoupling at the middle crustal level.
Both cross-sections, Figure 8a, b, suggest that the Adriatic lower crust has been indented into the European crust, thereby uplifting the Penninic basement units. The nature of the crustal structure clearly indicates a thick-skinned tectonic style, where the lower and upper crust deformed somewhat independently. A link from shallow upper crustal structures (for example thrust faults) to a deeper lower crustal level cannot be established. This suggests that thrust faults involving crystalline basement level off in the middle crust and pass into broad shear zones.
4. Kinematic succession of nappe formation
The various Alpine nappes discussed in Section 2 and the crustal structure described in Section 3 formed separately in time, location and depth. In order to display the kinematic evolution of these structures, a set of restored cross-sections through the Central Alps will be presented. The cross-sections rely on an original set of restored sections presented by Schmid et al. (Reference Schmid, Pfiffner, Froitzheim, Schönborn and Kissling1996) and Schmid, Pfiffner & Schreurs (Reference Schmid, Pfiffner, Schreurs, Pfiffner, Lehner, Heitzmann, Mueller and Steck1997); these works also give a detailed discussion on the dating of the various deformation phases involved in creating the Alpine nappe stack. A summary of the structural evolution of the Alps in these cross-sections accompanied by an orogenic time table is presented in Pfiffner (Reference Pfiffner2014).
Apart from structural data on folding and thrusting within the Alpine nappe pile, a retrodeformation also needs petrologic data, which indicate at which pressure and temperature conditions these structures formed. As examples, p–T–t paths obtained for the Adula and Tambo nappes (both discussed in Section 2) are shown in Figure 9. They are based on Marquer et al. (Reference Marquer, Baudin, Peucat and Persoz1994), Frey & Ferreiro Mählmann (Reference Frey and Ferreiro Mählmann1999) and Nagel et al. (Reference Nagel, De Capitani, Frey, Froitzheim, Stünitz and Schmid2002). In both cases, the timing of the p–T loops is relatively well constrained. Comparison of the two graphs (a) and (b) reveals that although tectonic burial for both nappes starts at around 50 Ma, the Adula nappe was buried much deeper compared to the Tambo nappe. In addition, within the Adula nappe the southern part was buried much deeper than the northern part. Following Petrini & Podladchikov (Reference Petrini and Podladchikov2000) and Mancktelow (Reference Mancktelow2008), the pressures determined from metamorphic mineral assemblages were converted to depths by allowing a contribution of tectonic overpressure of the order of 50% to these pressures. As discussed by Pleuger & Podladchikov (Reference Pleuger and Podladchikov2014), overpressures are likely to have varied in time and space. The 50% used in the reconstruction is thus a first-order approximation only.
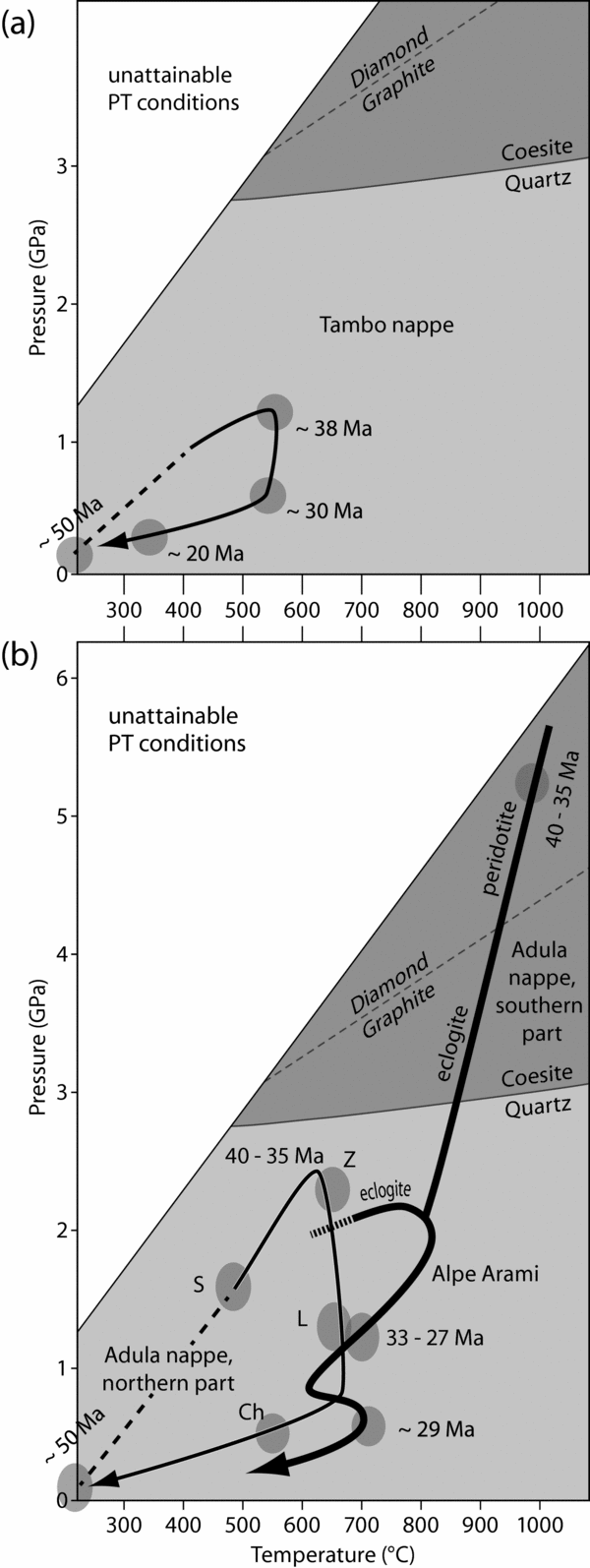
Figure 9. p–T–t paths determined for Penninic basement nappes of the Central Alps. (a) Tambo nappe. (b) Adula nappe. S, Z, L and Ch refer to deformation phases, where Z (Zapport) corresponds to a phase of nappe stacking.
The retrodeformed (restored) cross-sections constructed for time slices within the Cenozoic are shown in Figure 10. These sections are based on the original sections shown in Schmid et al. (Reference Schmid, Pfiffner, Froitzheim, Schönborn and Kissling1996) and Schmid, Pfiffner & Schreurs (Reference Schmid, Pfiffner, Schreurs, Pfiffner, Lehner, Heitzmann, Mueller and Steck1997) but have been substantially modified. They cover a total of at least c. 700 km of convergence between the European and Adriatic margins. The convergence numbers indicated are taken from Schmid et al. (Reference Schmid, Pfiffner, Froitzheim, Schönborn and Kissling1996) and Schmid, Pfiffner & Schreurs (Reference Schmid, Pfiffner, Schreurs, Pfiffner, Lehner, Heitzmann, Mueller and Steck1997).

Figure 10. Restored cross-section through the Central Alps of eastern Switzerland. See text for discussion. (a) Piemont ocean subducted, entrance of Briançon microcontinent. (b) Briançon cover detached. Valais basement (Adula) subducted, cover detached. (c) Stacking and expulsion of basement nappes (Suretta, Tambo, Adula). (d) Back-folding and thrusting along Insubric fault, Helvetic cover detached. (e) Further back-folding and thrusting along Insubric fault, indentation of Adriatic plate, basement of Gotthard and Aar massifs thrust and updomed. Su –Suretta; Ta – Tambo; AsS – Adula south; AsN – Adula north; Si – Simano; Lu – Lucomagno.

On a lithospheric scale the cross-sections reveal the rollback of the European plate from Paleocene time up into Oligocene time. The hinge of the plate is located south of the Briançon microcontinent at 65 Ma (Fig. 10a), in the Valais Basin at 50 Ma, south of Simano at 40 Ma and south of Lucomagno at 32 Ma. The rollback velocity may be estimated at 20 mm a−1 in Paleocene time and 10 mm a−1 in Eocene time. These numbers compare to the convergence rates determined by Schmid et al. (Reference Schmid, Pfiffner, Froitzheim, Schönborn and Kissling1996) and the progradation rates of the foreland bulge reported by Kempf & Pfiffner (Reference Kempf and Pfiffner2004). From Oligocene time on, important dextral strike-slip occurred along the Insubric fault. This brought a different piece of the leading edge of the Adriatic plate into the cross-section considered here.
Figure 10a shows the situation at 65 Ma. At this stage the Piemont Ocean had been subducted and the Briançon microcontinent enters the subduction zone. The 200 km of convergence accomplished so far is an absolute minimum estimate. The Valais Basin that had formed in Jurassic and mainly Cretaceous times (Steinmann, Reference Steinmann1994) had a basement consisting of thinned continental crust and new oceanic crust (Steinmann & Stille, Reference Steinmann and Stille1999). This substratum was to become the future Adula nappe.
By 50 Ma, after an additional convergence of about 200 km, the Briançon microcontinent was subducted, but its Mesozoic sedimentary cover had already been detached (Avers Phase of Milnes & Schmutz, Reference Milnes and Schmutz1978) and remained at a shallow level, marking the onset of thin-skinned tectonics. The basement of the Valais Basin was also subducted; its Mesozoic cover had been detached and remained at a more shallow level. The youngest sediments deposited in the Valais Basin are Early Eocene in age and were of a flysch character (Prättigau Flysch; Nänny, Reference Nänny1948); they were accumulated in the trench where active thrust faults broke the surface. The southern part of the continental basement, Adula S in Figure 10b, had reached a depth of around 100 km and was eclogitized, whereas the northern part, Adula N in Figure 10b, lagged behind, which explains the lesser degree of metamorphism this part acquired (cf. Fig. 9b).
Figure 10c depicts the subduction geometry at 40 Ma, after another 150 km of convergence. By this time the European continental margin entered the contact zone and triggered the onset of continent–continent collision (Schmid et al. Reference Schmid, Pfiffner, Froitzheim, Schönborn and Kissling1996; Schmid, Pfiffner & Schreurs, Reference Schmid, Pfiffner, Schreurs, Pfiffner, Lehner, Heitzmann, Mueller and Steck1997). At this time rapid decompression set in in the Tambo and Adula nappes (cf. Fig. 9a, b). This is explained by a detachment and rapid upward motion of the upper crust of the Briançon microcontinent. The upper crust was thereby shortened and stacked (Ferrera Phase of Milnes & Schmutz, Reference Milnes and Schmutz1978) to form the Tambo and Suretta nappes (Ta and Su in Figure 10c; see Scheiber, Pfiffner & Schreurs, Reference Scheiber, Pfiffner and Schreurs2012). These nappes were subsequently jammed into their original but detached sedimentary cover (Schams nappe), and overprinted its earlier formed thin-skinned style (Niemet-Beverin Phase of Schreurs, Reference Schreurs1995). In the case of the Adula nappe, the various pieces of continental and oceanic crust were stacked (Zapport phase, Z, in Fig. 9b) and expelled upwards. Buoyant rise may have contributed to this but the exact nature is still a matter of debate (see discussion in Nagel et al. Reference Nagel, De Capitani, Frey, Froitzheim, Stünitz and Schmid2002). Engi, Berger & Roselle (Reference Engi, Berger and Roselle2001) described the formation of this nappe stack within the subduction zone and proposed the term ‘tectonic accretion channel’ to explain its nature. In any case, this stack is also an example of basement-involved thin-skinned tectonics, and its emplacement into the higher units also deformed the earlier formed structures within the detached cover sediments.
By 32 Ma and another 50 km of convergence the geometry of the plate boundary had profoundly changed (Fig. 10d). Slab break-off of the leading edge of the European plate (not shown in Fig. 10) and the further advance of the European continental crust caused a buoyancy effect, which triggered vertical escape of the Penninic nappe stack. This was accomplished by the formation of a major S-vergent back-fold and steep back-thrusting along the Insubric fault. The latter was simultaneously active by dextral strike-slip. Melts that formed as a consequence of upwelling following slab break-off (von Blanckenburg & Davis, Reference Von Blanckenburg and Davis1995) were rising along the steep zone bordering the Insubric fault and intruded the Adula, Tambo and Suretta nappes (Bregaglia intrusion).
In the north, the sedimentary covers of the Lucomagno and Gotthard basement blocks were detached at about this time and moved northwards as Helvetic nappes (Calanda Phase of Pfiffner, Reference Pfiffner, Allen and Homewood1986) much in the style of thin-skinned tectonics. Still further north, the Northhelvetic Flysch Basin was being filled on top of the future Aar massif.
As shown in Figure 10e, in Miocene time back-folding and back-thrusting north of and along the Insubric fault continued and now also included Simano and Lucomagno (Si and Lu in Fig. 10e). Erosion accompanied (and facilitated) back-folding and back-thrusting such that by 19 Ma the Bregaglia intrusion was exhumed and shed pebbles into the Po Basin (Giger & Hurford, Reference Giger and Hurford1989).
In the north, the Helvetic nappes had travelled further north in a thin-skinned style and the Gotthard massif started to overthrust the Aar massif (basement-involved thin-skinned tectonics). The latter started updoming thereby deforming the now inactive basal thrust of the overlying Helvetic nappes (Pfiffner, Reference Pfiffner, Allen and Homewood1986). Molasse sedimentation prevailed in the Northalpine foreland basin.
Sedimentation also continued in the Po Basin to the south. The margin of the Adriatic plate now present in the cross-section considered here was shortened in a thick-skinned manner. As discussed in Schmid & Kissling (Reference Schmid and Kissling2000), the Adriatic plate underwent an anticlockwise rotation concurrent with the dextral strike-slip along the Insubric fault. As shown in Figure 10e, it is interpreted that this rotation indented the leading edge of the Adriatic plate into the European plate and that the Adriatic plate overrode the subducted European slab.
The overridden European slab likely had remnants of lower and maybe even upper continental crust that were still attached to the mantle lithosphere. Considering the depth range, one would expect that these crustal lenses were eclogitized in the process and thus not detectable by geophysical methods. It is these units that might be expected in the Moho gap and its southern extension discussed in conjunction with Figure 8b.
The kinematic sequence of nappe formation in this transect clearly shows that as the European margin entered the subduction zone, first the sedimentary cover was detached. This cover remained at a shallow depth whereas the crystalline basement was subducted to greater depths. Once there, the basement units were detached from their substratum and stacked into a nappe pile, which then moved upwards aided by buoyancy and erosion at the surface. The upward movement deformed the units at shallower depth and triggered post-nappe folding. The mechanism of thin-skinned frontal accretion followed by thick-skinned basal accretion has been analysed by numerical modelling by Selzer, Buiter & Pfiffner (Reference Selzer, Buiter and Pfiffner2008). The model study suggested that erosion enhances the formation of thick-skinned-type basement nappes at depth, while sedimentation at the front of the orogen favours the formation of thin-skinned-type nappes.
5. Mechanical implications
Structural analyses clearly show that, generally speaking, in the Alps the sedimentary cover was detached in an early stage of nappe formation and was followed by the stacking of basement nappes. Considering the Alpine cover nappes, detachment occurred mainly along Triassic evaporites, which are present in many instances. Such a detachment is possible at very low temperatures of less than 150°C, as was the case, for example, in the Jura Mountains.
In the case of crystalline basement nappes, the presence of Permo-Carboniferous and Mesozoic basins embedded in gneissic and granitic basement played some role during nappe formation. Such basins are associated with steep synsedimentary normal faults bounding them. It seems that the lateral heterogeneity present at the margins of these basins controlled the initiation of thrust faults. Examples of this behaviour include the Glarus Verrucano in the Glarus nappe (Pfiffner, Reference Pfiffner2014) and the Rofna Porphyry complex in the Suretta nappe (Scheiber, Pfiffner & Schreurs, Reference Scheiber, Pfiffner and Schreurs2012), both of which are Permian structures. Bellahsen et al. (Reference Bellahsen, Jolivet, Lacombe, Bellanger, Boutoux, Garcia, Mouthereau, Le Pourhiet and Gumiaux2012) discussed the localization of basement deformation by Mesozoic syn-rift basins that led to thick-skinned tectonics in the Western Alps. Their conclusion is that the Jurassic normal faults were not directly reactivated but caused shear zones tens or hundreds of metres thick that extend into the basement and led to fold-like structures at the cover–basement interface.
In the absence of such syn-rift basins, basement nappes are typically 1 to 5 km thick and tens of kilometres long in the direction of transport, suggesting that detachment occurred within the upper crust and along a horizontal or shallow-dipping horizon. The determination of the depth of detachment requires the retrodeformation of the Alpine overprint. For the Suretta nappe (Fig. 4a) Scheiber, Pfiffner & Schreurs (Reference Scheiber, Pfiffner and Schreurs2012) reported a depth of 5 km for the detachment horizon at the time of its initiation. In the Tambo nappe (Fig. 4b) the estimate for this depth is 8 km (Mayerat-Demarne, Reference Mayerat-Demarne1994), and in the Bernhard nappe complex (Scheiber, Pfiffner & Schreurs, Reference Scheiber, Pfiffner and Schreurs2013) about 10 km.
In the case of the external basement massifs, the reflection seismic data of NRP20 (Pfiffner, Sahli & Stäuble, Reference Pfiffner, Sahli, Stäuble, Pfiffner, Lehner, Heitzmann, Mueller and Steck1997) indicate that in the Central Alps, detachment faults reach down to depths of more than 10 km beneath the top-basement contact; however, a levelling off of the faults at depth cannot be derived from these data. In the Western Alps, Bellahsen et al. (Reference Bellahsen, Mouthereau, Boutoux, Bellanger, Lacombe, Jolivet and Rolland2014) proposed that the thrust faults at the front of the external massifs reach down to 15 or 20 km depth; but here too, an interpretation of the geometry at greater depth is not presented. Lacombe & Mouthereau (Reference Lacombe and Mouthereau2002) proposed that a thermally weakened middle crust could be responsible for the detachment and caused the decoupling of lower and upper crust. Considering the relatively small displacements along individual thrust faults related to the external basement massifs (around 5 km, in a few instances 10 km), it is well possible that these faults die out at greater depth owing to differential stretching in the footwall and hanging wall parallel to the thrust faults compensating for the displacement (Pfiffner, Reference Pfiffner1985). For example, a displacement of 10 km can die out after 1 km distance along the fault if a differential stretch of 10% is assumed. Differential stretches may be envisaged if a fault gives way to a broad shear zone in the ductile regime.
Regarding the depth of the initiation of detachments, it is interesting to consider experimental data on rock strengths. Figure 11 shows a compilation of experimental data of plastic strengths for typical rock types involved in nappe formation after Suppe (Reference Suppe1984). The data are normalized to strain rates of 10−13 s−1, which correspond to typical strain rates determined in orogens (Pfiffner & Ramsay, Reference Pfiffner and Ramsay1982). Brittle strength curves are given for hydrostatic pressure and for overpressure after Suppe (Reference Suppe2014). In addition, the strength reduction caused by the breakdown of feldspar as a load-bearing phase to phyllonite based on O'Hara (Reference O'Hara2007) is also shown.

Figure 11. Experimentally determined strengths of typical rocks encountered in continental crust. Arrows indicate potential paths taken by an uppermost crustal block made of crystalline basement and cover sediments. Strength curve of anhydrite is reached long before strength curve of quartzite. Phyllonite production by breakdown of feldspar may occur at temperatures comparable to or lower than ductile deformation of quartz. ops – overpressured brittle strength.
If a small volume of crystalline basement topped by sediments (represented by dark grey rectangles in Fig. 11) is considered, which is then buried as would be typical for orogenic processes, temperature and differential stress would increase with time. The path this volume of rocks would take could follow any of the arrows shown in Figure 11, depending on the local conditions of stress and the reigning geothermal gradient. Except for the unlikely horizontal path corresponding to increase in differential stress without temperature rise, the rock volume under consideration would hit the strength curve of anhydrite first, and in a much later stage reach the strength curves of limestone or quartz. Thus, it is to be expected that evaporites (anhydrite) trigger detachment in an early stage of burial by orogenesis. The temperatures required for strength reduction of quartz or the breakdown of feldspar to produce phyllonites are higher and thus reached much later at greater depths in a subduction regime. This explains why in Alpine-type nappes, thin-skinned tectonics precede basement-involved thin-skinned and thick-skinned tectonics.
The fact that basement nappes are thin slabs of regular thickness may reflect that the detachment followed a pre-existing zone of weakness or, alternatively, that strength reduction owing to higher temperatures occurred at a certain depth beneath the basement–cover contact. In the case of the Tambo nappe, the rocks at the detachment horizon were at more than 400°C (see Fig. 9a) and thus could have allowed a weak zone to develop 8 km beneath the basement–cover contact, which then eventually developed into a detachment horizon. This implies that the palaeogeotherms remained more or less parallel to the basement–cover contact. Unfortunately, the rocks at the very base of the Tambo nappe are not exposed, rendering the study of a potential strain gradient impossible. Baudin, Marquer & Persoz (Reference Baudin, Marquer and Persoz1993) reported a heterogeneous distribution of ductile deformation within the Tambo nappe with shearing being more pronounced at the top of the nappe.
In contrast, the Suretta nappe displays a quartzofeldspathic mylonite up to 100 m thick at its base (Scheiber, Pfiffner & Schreurs, Reference Scheiber, Pfiffner and Schreurs2012). The presence of ribbon quartz and ribbon feldspar point to elevated temperatures and, associated, low strength of the porphyritic protolith. As discussed by Scheiber, Pfiffner & Schreurs (Reference Scheiber, Pfiffner and Schreurs2012), the basal detachment of the Suretta nappe was also controlled by the presence and shape of the Rofna Porphyry complex: the basal detachment followed the base of the intrusive complex, the frontal ramp its lateral contact.
Carry et al. (Reference Carry, Gueydan, Brun and Marquer2009) modelled the effect of pressure and temperature increase in a continental margin as it is being subducted. In their model, the pressure increase with depth during subduction is followed by temperature increases owing to heating from the overlying hot lithosphere. As a consequence the strength of the margin first increases and then decreases as temperatures rise. The strength gradients in the model suggest that stacking of thin crustal slices becomes possible once the subducting slab has reached a certain depth, which itself depends on subduction dip angle, crustal rheology, heat flux and subduction velocity (op. cit.).
A different mechanism for weakening crystalline rocks is the transformation of the load-bearing feldspar by mechanically weaker phyllosilicates (Jefferies et al. 2005; O'Hara, Reference O'Hara2007). In this mechanism low-temperature cataclasis provides the permeability for the passage of fluids, which in turn aid the breakdown of feldspar and the formation of phyllonite. The breakdown of feldspar and the associated strength reduction of the rocks can occur at temperatures between 200 and 400°C (O'Hara, Reference O'Hara2007), and thus prior to the conditions for the weakening of quartz (see Fig. 11). Such a scenario for strength reduction has been documented for the Ecrins massif in the Western Alps by Bellanger et al. (Reference Bellanger, Bellahsen, Jolivet, Baudin, Augier and Boutoux2014) and is also plausible in the external basement massifs (Aar, Aiguilles Rouges, Mont Blanc, Belledonne) where the degree of metamorphism attained and the temperatures operative during thrusting are modest in comparison to the subducted basement nappes of the Penninic nappe system. Lacombe & Mouthereau (Reference Lacombe and Mouthereau2002) suspected that thermal weakening within the middle crust could represent a detachment horizon in foreland thrust belts and contribute to the formation of antiforms like the external basement massifs in the Alps. Nevertheless, as pointed out by Bellahsen et al. (Reference Bellahsen, Mouthereau, Boutoux, Bellanger, Lacombe, Jolivet and Rolland2014), the internal structures of these external massifs vary along strike, a change that these authors attributed to the impact of Mesozoic rifting. They attributed the higher shortening observed in the Central Alps (Aar, Mont Blanc – Aiguilles Rouges) compared to the Western Alps (Belledonne) to a more strongly thinned crust in the Central Alps that was more deeply buried during collision, heated up, and thereby weakened and more strongly deformed. In contrast, the buoyant thicker crust of the Belledonne massif in the Western Alps remained at a shallower level, thus cooler and consequently experienced less pervasive deformation.
Comparing the external massifs and the Penninic basement nappes it becomes clear that the latter were buried much deeper and the crust consequently weakened. In the case of the Tambo, Suretta and Bernhard nappe complex, nappes derived from the Briançon microcontinent, the upper crust was detached from the lower crust and expelled from depth while the lower crust was subducted (Schmid et al. Reference Schmid, Pfiffner, Froitzheim, Schönborn and Kissling1996). In the case of the Adula nappe, Mesozoic rifting led to the formation of extensional allochthons which were sheared off their lower crust. The stacking of these allochthons occurred at temperatures in excess of 400°C (Fig. 9b). Thrusting thus affected a weak crust and may have followed shallow-dipping parts of Jurassic normal faults. Such a scenario is also envisaged in the case of the Austroalpine nappes, where Mohn et al. (Reference Mohn, Manatschal, Masini and Müntener2011) discussed the importance of rift-related structures on the formation of Alpine nappe structures, namely the partial reactivation of low-angle Jurassic faults. These hyper-extended situations result in the formation of very thin basement nappes owing to their Mesozoic history. Stacking of these basement nappes results in an overall geometry resembling thin-skinned cover nappes in size.
6. Conclusions
In the Helvetic–Dauphinois and Penninic nappe systems of the Alps, detachment of cover sediments by thin-skinned tectonics occurred early in the orogenesis. It was followed by basement-involved thin-skinned tectonics in the Penninic nappe system. In the course of this, the earlier formed thrust faults were intricately folded (‘post-nappe folding’). Thick-skinned tectonics involving the entire upper crust took place in the latest phase of collision in the Helvetic–Dauphinois nappe system.
The presence of evaporite formations was responsible for the detachment of cover nappes in many cases. Fluid overpressures may have played a role in shaly or marly formations.
Basement-involved thin-skinned tectonics can be attributed to originally thinned continental crust that was subducted to greater depths. In these nappes, strength reduction by rising high temperatures led to ductile deformation that controlled detachment of thin upper crustal pieces. Pre-existing mechanical discontinuities (e.g. Permo-Carboniferous magmatic complexes or basins) acted as a focus for the development of detachment and fault ramps.
The thick-skinned tectonic style in the external basement massifs, which occurred late in the collision stage, involves thrusts reaching 12 km down into the middle crust. A detachment at this level could have been controlled by the breakdown of feldspar and formation of phyllonites. Reflection seismics, however, do not show a phyllonite layer of sufficient thickness to be detectable seismically. Alternatively, a gradual decrease of the displacement along these thrust faults accommodated by differential stretching of the hanging wall and/or footwall rocks may be responsible for the disappearance of these thrust faults.
The crustal-scale disharmony between upper and lower crust requires some ductile or semi-ductile deformation at the middle crustal level. The wavy nature of the top of the lower crust cannot be correlated with the structure of the upper crust related to thick-skinned tectonics.
Acknowledgements
I would like to thank Alfons Berger for the many discussions on the structure of the Adula nappe and Edi Kissling for his advice on how to interpret the earthquake tomography data. The detailed comments by C. Rosenberg, O. Lacombe and an anonymous reviewer greatly improved the quality of the manuscript.