1. Introduction
Black shales are organic-rich sediments that commonly formed coevally in space and time through Earth history and have been associated with perturbations in the carbon cycle, climate change and global extinction events (Schlanger & Jenkyns, Reference Schlanger and Jenkyns1976; Arthur & Sagemann, Reference Arthur and Sagemann1994; Sagemann et al. Reference Sagemann, Murphy, Werne, Ver Straeten, Hollander and Lyons2003; Jenkyns, Reference Jenkyns2010). They are characterized by rapid and efficient accumulation of organic matter in marine sediments, either by increased primary production or efficient preservation. Black shales commonly contain phosphorus-rich rocks referred to as phosphorites, which are defined by P2O5 contents of 18 wt.% or higher (Föllmi, Reference Föllmi1996). Phosphorites and black shales require similar formation conditions and are therefore commonly encountered together in the rock record. Phosphogenesis, which describes the precipitation of authigenic phosphorus minerals in marine sediments, occurs within a specific environmental spectrum where ocean circulation, sedimentation and the preservation of organic matter during early diagenesis allow for phosphorus to accumulate sufficiently in sedimentary pore waters (Glenn et al. Reference Glenn, Föllmi, Riggs, Baturin, Grimm, Trappe, Abed, Galli-Oliver, Garrison, Ilyin, Jehl, Rohrlich, Sadaqah, Schildowski, Sheldon and Siegmund1994; Föllmi, Reference Föllmi1996; Benitez-Nelson, Reference Benitez-Nelson2000; März et al. Reference März, Poulton, Beckmann, Küster, Wagner and Kasten2008; Küster-Heins et al. Reference Küster-Heins, Steinmetz, De Lange and Zabel2010). In the modern ocean, such conditions exist in suboxic to anoxic marine sediments typified by a constant supply of organic matter, as observed in coastal upwelling zones, continental margin sediments or restricted marine basins (Filipelli & Delaney, Reference Filipelli and Delaney1996; Schenau et al. Reference Schenau, Slomp and De Lange2000; Filipelli, Reference Filippelli2011; Lomnitz et al. Reference Lomnitz, Sommer, Dale, Löscher, Noffke, Wallmann and Hensen2016). Because phosphorus is highly mobile and is cycled efficiently during early diagenesis, persistent anoxic conditions are required to allow for phosphorus minerals to precipitate (Ruttenberg & Berner, Reference Ruttenberg and Berner1993; Ingall & Jahnke, Reference Ingall and Jahnke1994; Föllmi, Reference Föllmi1996; Kraal et al. Reference Kraal, Slomp, Reed, Reichart and Poulton2012). Phosphogenesis typically requires multiple phosphorus sources including the anaerobic microbial degradation of organic matter, the reductive dissolution of iron oxides releasing adsorbed phosphate, as well as the degradation and dissolution of bone material and fish debris (Jensen et al. Reference Jensen, Mortensen, Andersen, Rasmussen and Jensen1995; Schenau et al. Reference Schenau, Slomp and De Lange2000; Smith et al. Reference Smith, Glover, Treude, Higgs and Amon2015). Since phosphorus minerals such as carbonate fluorapatite are common in organic-rich black shales, these deposits are regarded as type sections for phosphogenesis that hold important clues on the driving processes enabling the formation of sedimentary phosphate minerals (Filippelli, Reference Filippelli2011).
Phosphatic stromatolites have been found in Proterozoic and Phanerozoic carbonate, phosphorite and black shale lithologies (Krajewski et al. Reference Krajewski, Lesniak, Lacka and Zawidzki2000; Caird et al. Reference Caird, Pufahl, Hiatt, Abram, Rocha and Kyser2017; Sallstedt et al. Reference Sallstedt, Bengston, Broman, Crill and Canfield2018; Zoss et al. Reference Zoss, Ferrer, Flood, Jones, Louw and Bailey2019). Stromatolites are lithified microbial build-ups that are the result of the interaction between various microbial metabolic processes and their sedimentary environment (Dupraz & Visscher, Reference Dupraz and Visscher2005; Allwood et al. Reference Allwood, Walter, Burch and Kamber2007; Sallstedt et al. Reference Sallstedt, Bengston, Broman, Crill and Canfield2018). Stromatolites in the fossil record have commonly been interpreted as products of cyanobacteria due to their remarkable similarity to modern cyanobacterial mats (Stal, Reference Stal and Whitton2012). A cyanobacterial origin has also been suggested for phosphatic stromatolites, occurring in shallow marine settings due to the presence of preserved oxygen gas bubbles (Bosak et al. Reference Bosak, Liang, Sim and Petroff2009; Sallstedt et al. Reference Sallstedt, Bengston, Broman, Crill and Canfield2018), laminated fabrics related to trapping and binding mechanisms, stable isotope analyses, mineralogy, as well as facies analyses of the host sediments (Rao et al. Reference Rao, Rao and Raju2000, Reference Rao, Michard, Naqvi, Böttcher, Krishnawamy, Thamban, Natarajan and Borole2002; Lundberg & McFarlane, Reference Lundberg and McFarlane2011; Drummond et al. Reference Drummond, Pufahl, Porto and Carvalho2015; Caird et al. Reference Caird, Pufahl, Hiatt, Abram, Rocha and Kyser2017; Sallstedt et al. Reference Sallstedt, Bengston, Broman, Crill and Canfield2018). Modern examples of such cyanobacterial phosphatic stromatolites are scarce and have only recently been described from a low-phosphorus terrestrial environment (Büttner et al. Reference Büttner, Isemonger, Isaacs, van Niekerk, Sipler and Dorrington2021). In contrast to these photosynthesis-based cyanobacterial stromatolites, phosphogenesis can result from organic matter degradation by anaerobic, sulphate-reducing bacteria (Thamdrup & Canfield, Reference Thamdrup and Canfield1996; Benitez-Nelson, Reference Benitez-Nelson2000; Arning et al. Reference Arning, Lückge, Breuer, Gussone, Birgel and Peckmann2009a; Berndmeyer et al. Reference Berndmeyer, Birgel, Brunner, Wehrmann, Jöns, Bach, Arning, Föllmi and Peckmann2012). A further relationship between bacteria involved in the sulphur cycle and phosphogenesis has been suggested for various ancient and modern phosphorite and phosphorus-rich deposits (Williams & Reimers, Reference Williams and Reimers1983; Schulz & Schulz, Reference Schulz and Schulz2005; Bailey et al. Reference Bailey, Joye, Kalanetra, Flood and Corsetti2007, Reference Bailey, Corsetti, Greene, Crosby, Liu and Orphan2013; Arning et al. Reference Arning, Lückge, Breuer, Gussone, Birgel and Peckmann2009a; Cosmidis et al. Reference Cosmidis, Benzerara, Menguy and Arning2013; Zwicker et al. Reference Zwicker, Smrzka, Steindl, Böttcher, Libowitzky, Kiel and Peckmann2021), in particular with regard to the large, colourless chemotrophic sulphide-oxidizing bacteria. These bacteria take up and release phosphate into the pore waters during early diagenesis and are capable of storing polyphosphate within their cells (Schulz & Schulz, Reference Schulz and Schulz2005; Sievert et al. Reference Sievert, Keine and Schultz-Vogt2007; Zopfi et al. Reference Zopfi, Böttcher and Jørgensen2008; Goldhammer et al. Reference Goldhammer, Brüchert, Ferdelman and Zabel2010).
Here we report chemotrophy-based phosphatic microstromatolites enclosed in black shales deposited after the Devonian–Carboniferous transition in the course of the globally coeval, transgressive Lower Alum Shale Event (Sobolev et al. Reference Sobolev, Zhuravlev and Tsyganko2000; Siegmund et al. Reference Siegmund, Trappe and Oschmann2002; Kaiser et al. Reference Kaiser, Becker, Steuber and Aboussalam2011; Becker et al. Reference Becker, Hartenfels, Weyer and Kumpan2016, Reference Becker, Hartenfels and Kaiser2021). These black shales are rich in phosphorous concretions that host phosphatic microstromatolites of various size and morphology. Using a comprehensive approach combining petrography, mineralogy and isotope geochemistry, it is suggested that the stromatolite-forming microbial communities thrived in deep-water environments and were independent of sunlight but relied on chemotrophic metabolic pathways.
2. Geological setting and locality
2.a. Tectonic setting
The Rhenohercynian Basin is part of the Avalonian Plate and was related to numerous active subduction zones associated to the closing Rheic Ocean (Oncken et al. Reference Oncken, Plesch, Weber, Ricken, Schrader, Franke, Haak, Oncken and Tanner2000). The Rhenohercynian fold and thrust belt, which comprises the Rhenish Massif, are part of the Middle European Variscides that occur as well exposed and complete Middle Devonian to Lower Carboniferous successions (Oncken et al. Reference Oncken, von Winterfeld and Dittmar1999). The Rhenohercynian Zone has been characterized as an evolving rift system developing on Upper Devonian to Lower Carboniferous subsiding shelf sediments of the Old Red Continent (von Raumer et al. Reference von Raumer, Nesbor and Stampfli2017). This zone, along with the Saxothuringian and the Moldanubian zones, divides the Central European Variscides from the northwest to the southeast (Kossmat, Reference Kossmat1927; Brinkmann, Reference Brinkmann1948). The Rhenish Massif has been associated with the Avalonian Terrain that separated from Gondwana in the Early Ordovician (Oncken et al. Reference Oncken, Plesch, Weber, Ricken, Schrader, Franke, Haak, Oncken and Tanner2000; Eckelmann et al. Reference Eckelmann, Nesbor, Königshof, Linnemann, Hofmann, Lange and Sagawe2014). The resulting Rheic Ocean began to close in the Early Devonian up to the Carboniferous and involved the formation of several microplates separating the closing ocean from the Paleotethys and the Rhenohercynian Ocean by island arcs, which accreted to Avalonia in the Early Devonian (Oncken & Weber, Reference Oncken, Weber, Dallmeyer, Franke and Weber1995). The opening of the Rhenohercynian Ocean has been related to an active Laurussian continental margin that separated the Avalonian terranes (von Raumer & Stampfli, Reference von Raumer and Stampfli2008; Zeh & Gerdes, Reference Zeh and Gerdes2010). These island arcs are documented by rocks of the Mid-German Rise, or ‘Mid-German Crystalline Zone’ (Altenberger et al. Reference Altenberger, Besch, Mocek, Zaipeng and Yong1990; Dombrowski et al. Reference Dombrowski, Henjes-Kunst, Höhndorf, Kröner, Okrusch and Richter1995), which separates the Rhenohercynian from the Saxothuringian zones (Zeh & Gerdes, Reference Zeh and Gerdes2010).
The Rhenohercynian Basin developed as an elongated, narrow trough between the Mid-German Rise to the south and the London-Brabant High in the north as part of the Old Red Continent during the Middle Devonian (Königshof et al. Reference Königshof, Becker and Hartenfels2016). The Upper Devonian sedimentary rocks of the Rhenohercynian Basin are part of the Hercynian Facies that comprise siltstones and red shales, reef carbonates and bioherms and drowned carbonate platforms (Becker et al. Reference Becker, Libowitzky, Kleinschrodt and Bohatý2016). The Late Devonian reefs were subject to several global extinction events such as the Kellwasser crisis in the latest Frasnian and the Hangenberg Event at the Devonian–Carboniferous boundary (Becker et al. Reference Becker, Hartenfels, Weyer and Kumpan2016). Deposits of the lowermost Carboniferous in the Rhenish Massif comprise cherts, organic-rich black shales and turbiditic limestones, which have been collectively associated with the ‘Kulm-Facies’. In the Early Carboniferous, the Rhenohercynian Basin was successively closed and subducted under the Mid-German High, forming an active continental margin as evidenced by extensive local volcanism (Oncken & Weber, Reference Oncken, Weber, Dallmeyer, Franke and Weber1995; Siegmund et al. Reference Siegmund, Trappe and Oschmann2002).
2.b. Upper Devonian to Mississippian strata at Drewer
The Drewer quarry represents the northernmost locality of the Rhenish Massif cropping out within several anticlinal and synclinal structures (Becker et al. Reference Becker, Hartenfels, Weyer and Kumpan2016), which are part of a larger thrust and fold belt with numerous synclinal and anticlinal systems from the Velbert and Remscheid-Altena anticlines to the west and the larger Warstein anticline to the south (Fig. 1). Drewer is located within the Belecke anticline, which formed an intrabasinal swell in a starved basin during the Early Carboniferous (Clausen et al. Reference Clausen, Leuteritz and Ziegler1989; Korn, Reference Korn2010). It represents a key lithostratigraphic and biostratigraphic locality for the Devonian–Carboniferous boundary displaying Milankovich cyclicity, several regressions and transgressions, as well as extinction events as recorded by the Hangenberg and Lower Alum Shale Events (Becker, Reference Becker1992, Reference Becker1999; Korn et al. Reference Korn, Clausen, Belka, Leuteritz, Luppold, Feist and Weyer1994; Siegmund et al. Reference Siegmund, Trappe and Oschmann2002; Kumpan et al. Reference Kumpan, Babek, Kalvoda, Grygar, Fryda, Becker and Hartenfels2015; Becker et al. Reference Becker, Hartenfels, Weyer and Kumpan2016). These two black shale intervals have been correlated globally to coeval strata in Spain, France and Poland, as well as to Hangenberg Event strata from India (Ganai & Rashid, Reference Ganai and Rashid2019), Vietnam (Komatsu et al. Reference Komatsu, Kato, Hirata, Takashima, Ogata, Oba, Naruse, Ta, Nguyen, Dang, Doan, Nguyen, Sakata, Kaiho and Königshof2014), China (Zhang et al. Reference Zhang, Becker, Ma, Zhang and Zong2019), Morocco (Kaiser et al. Reference Kaiser, Becker and El Hassani2007), Italy (Spalletta et al. Reference Spalletta, Corradini, Feist, Korn, Kumpan, Perri, Pondrelli and Venturini2021) and the USA (Lu et al. Reference Lu, Lu, Ikejiri, Hogancamp, Sun, Wu, Carroll, Cemen and Pashin2019; Martinez et al. Reference Martinez, Boyer, Droser, Barrie and Love2019; Barnes et al. Reference Barnes, Husson and Peters2020). Facies changes are recorded at Drewer starting with the latest Famennian nodular Wocklum Limestone and the calcareous, laminated Drewer Sandstone (Luppold et al. Reference Luppold, Clausen, Korn and Stoppel1994), followed by the Hangenberg Black Shale and intercalated Hangenberg Sandstone (Clausen et al. Reference Clausen, Leuteritz and Ziegler1989). The Devonian–Carboniferous boundary at Drewer is represented by the Stockum Limestone composed of alternating limestones and marls, followed by the Lower Tournaisian Hangenberg Limestone characterized as typical cephalopod limestone (Becker et al. Reference Becker, Hartenfels, Weyer and Kumpan2016). A sharp transition to phosphorus-rich black shales associated with the global Lower Alum Shale Event (cf. Becker, Reference Becker1992) represents a carbonate production and ecosystem collapse during a maximum flooding event (Korn, Reference Korn2010; Becker et al. Reference Becker, Hartenfels, Weyer and Kumpan2016). The abrupt transition from cephalopod-rich limestones to black shales marks the lower boundary of the German Kulm facies postdating the Hangenberg Event and the Devonian–Carboniferous boundary. The uppermost strata at Drewer features grey, crinoidal limestones, which have been correlated to the Erdbach Limestone of the southern Rhenish Massif (Becker et al. Reference Becker, Hartenfels, Weyer and Kumpan2016).

Figure 1. Geologic overview of the Rhenish Massif east of the Rhine river with a focus on Upper Devonian to Lower Carboniferous strata and the location of the Drewer quarry (see red box); after Königshof et al. (Reference Königshof, Becker and Hartenfels2016).
3. Material and methods
The northwestern face of the Drewer quarry was logged over an 8-meter section, producing a detailed profile of the Lower Alum Black Shale facies. Thin sections of phosphorus-rich black shales were prepared. For bulk rock powder X-ray diffraction (XRD) analysis, samples were crushed to fine powder in an agate mortar. XRD analysis of carbonate-cemented background sediment was performed on a powder sample obtained with a handheld micro-drill from a polished slab. XRD measurements were carried out at the Crystallography Department (University of Bremen), using a Philips X’Pert Pro MD X-ray diffractometer with a Cu-Kα tube (λ = 1.541; 45 kV, 40 mA). Scanning electron microscopy (SEM) was conducted on a conventional tungsten filament SEM (FEI Inspect S) and a field-emission-gun scanning electron microscope with integrated focused ion beam (FEI QuantaTM 3D FEG) and an energy-dispersive X-ray detection unit (EDAX Apollo XV) at the Institute for Geology of the University of Vienna. Data processing was conducted using the EDAX TEAMTM V3.1.1 software. The presence of pyrite was determined using a Cameca SX-100 electron microprobe at the Faculty of Geosciences, University of Bremen. Analytical conditions included an acceleration voltage of 20 kV, beam current of 10 nA and a defocussed beam of 1–2 µm diameter. Counting times were 20 seconds on peak and 10 seconds on background. For quantification natural minerals from the collections of the University of Vienna and the Smithsonian Institution were used (Jarosewich et al. Reference Jarosewich, Nelen and Norberg1980) and the built-in PAP matrix correction.
Fourier-transform infrared (FTIR) spectra were acquired from 370 to 4,000 cm−1 on a Bruker Tensor 27 FTIR spectrometer equipped with a glo(w)bar MIR light source, a KBr beam splitter and a DLaTGS detector at the Department of Mineralogy and Crystallography of the University of Vienna. A polished thin section was pressed on the 2 × 3 mm diamond window of a Harrick MVP 2 diamond attenuated total reflectance (ATR) accessory in such a way that either the apatite or the calcite components were predominantly probed. For comparison, spectra of blackboard chalk and a pure fluorapatite crystal from Durango, Mexico, were acquired. Sample and background spectra were averaged from 32 scans at 4 cm−1 spectral resolution. Background spectra were obtained from the empty ATR unit. Data handling was performed with OPUS 5.5 software (Bruker Optik GmbH, 2005).
Sample powders for carbon and oxygen stable isotope analysis of phosphate-associated carbonate (PAC) of carbonate fluorapatite were obtained from polished rock slabs using a handheld microdrill. A total of 14 samples were prepared; four from phosphatic microstromatolites, five from the phosphatic concretions and five samples from the host rock surrounding the concretions. Stable isotope analyses were conducted at IOW using a Thermo Scientific Gasbench II connected to a Thermo Finnigan MAT 253 gas mass spectrometer via a Thermo Scientific Conflo IV split interface following Böttcher et al. (Reference Böttcher, Neubert, Escher, von Allmen, Samankassou and Nägler2018). It was assumed that phosphoric acid reaction with apatite and calcite to release carbon dioxide is associated with the same kinetic 18O/16O fractionation effect (Kolodny & Kaplan, Reference Kolodny and Kaplan1970; Loeffler et al. Reference Loeffler, Fiebig, Mulch, Tütken, Schmitt, Bajnai, Conrad, Wacker and Böttcher2019). Isotope values given in ‘‰’ are equivalent to ‘mUr’ (milli-Urey; Brand & Coplen, Reference Brand and Coplen2012). The carbon and oxygen isotope data are given with respect to the V-PDB standard with a reproducibility of better than ±0.10 mUr and ±0.15 mUr, respectively. Due to phase-specific sampling, the determined δ13C values from the phosphatic microstromatolites represent almost pure apatite-bound inorganic carbon.
4. Results
4.a. Lithostratigraphy of the Devonian–Carboniferous boundary at Drewer
The Devonian–Carboniferous boundary section at Drewer exposes the Wocklum Limestone, Hangenberg Black Shale and Sandstone, Hangenberg Limestone, Lower Alum Black Shale, equivalents of the Erdbach Limestone and the siliceous Kulm siltstones (Fig. 2; cf. Korn et al. Reference Korn, Clausen, Belka, Leuteritz, Luppold, Feist and Weyer1994; Becker et al. Reference Becker, Hartenfels, Weyer and Kumpan2016). The Wocklum Limestone is grey to dark grey, nodular, partly sparitic and is well-bedded in the upper part with a sharp transition to the Hangenberg Black Shale, which is composed of darker grey to black, slaty to flaky bands of shale that are partly unconsolidated. The overlying Hangenberg Sandstone is fine grained and well sorted, with a gradational contact to the Hangenberg Limestone above. The transitional section of the lower part of the Hangenberg Limestone comprises fine-grained siltstone with several thin nodular limestone beds, whereas the main limestone is grey and massive. The shift to the Lower Alum Black Shale is marked by thin siltstone and limestone beds, which are 10–15 cm thick (Fig. 2). The Lower Alum Black Shale measures approximately 130 cm in thickness and is described in detail below. The grey to dark grey, massive, mostly sparitic Erdbach Limestone above the Lower Alum Black Shale is approximately 80 cm thick, followed by the black, very fine-grained, siliceous Kulm siltstone.

Figure 2. Outcrop photograph and sedimentary log of the investigated outcrop in the Drewer quarry; person for scale.
4.b. Lithostratigraphy of the Lower Alum Black Shale interval at Drewer
The detailed profile of the Lower Alum Black Shale (Fig. 3) begins with the uppermost parts of the Hangenberg Limestone, a micritic, bioclastic wackestone (cf. Becker et al. Reference Becker, Hartenfels, Weyer and Kumpan2016) that grades into weakly carbonaceous siltstones representing the last phase of deposition before the Lower Alum Shale Event. The Lower Alum Black Shale interval at Drewer has been divided into nine units, which are designated as units 5a to 5i (Fig. 3). Unit 5a comprises 40 cm of dark grey clay with pyrite-bearing intervals and 0.5–10 mm thick beds. Unit 5b includes 30 cm of a similar lithology as unit 5a with clay, as well as first occurrences of isolated, tabular phosphatic concretions up to 2 cm in width and 0.5 cm in thickness. Unit 5c is similar in appearance with more individual tabular concretions, which occur more frequently in unit 5d. In this unit, various concretion morphologies from tabular to platy to nodular are present (Fig. 3). Unit 5e shows wavy lamination within the finely bedded shales, as well as cracks and fissures filled with weathered material. Unit 5f exhibits a clear transition from unit 5e marked by numerous horizontal, tabular and partly overlapping phosphorite beds (Figs. 3, 4a). This bed is characterized by its greyish colour and more abundant concretions (Fig. 3). Units 5g and 5h are replete in nodular to oval concretions measuring up to 10 cm in width that occur in close proximity to each other (Fig. 3). Unit 5i is the uppermost Lower Alum Black Shale unit at Drewer and features wavy lamination and tabular, elongated and occasionally irregular and fragmented concretions orientated with their long axes parallel to bedding (Figs. 3, 4). Some smaller concretions are spherical. This unit is capped by a sharp transition to the pyrite-bearing, dark grey, Erdbach limestone (Fig. 3).

Figure 3. Detailed lithostratigraphic log and an outcrop photograph showing the corresponding beds of the Lower Alum Black Shale at Drewer; hammer for scale.

Figure 4. Outcrop photographs of the Lower Alum Black Shale at Drewer. (a) Elongated, flat phosphatic concretions within the transition of beds 5f to 5g. (b) Bed 5h with larger, oval to spherical phosphatic concretions overlain by bed 5i and the Erdbach limestone; folding rule for scale. (c) Detail corresponding to the left red rectangle in (b) showing numerous oval phosphatic concretions within bed 5h; coin for scale. (d) Detail corresponding to the right red rectangle in (b) with spherical phosphatic concretions within laminated black shales from bed 5h; coin for scale.
4.c. Petrography, mineralogy and stable isotope geochemistry
Lower Alum Black Shale unit 5h was chosen for sampling and detailed investigation due to its high content of phosphatic concretions (Fig. 5). Two microfacies were identified in these samples referred to as microfacies 1 and 2 from hereon (cf. Fig. 5a). Microfacies 1 is composed of silty shales and mudrocks featuring a pelitic matrix with poorly sorted components that comprises around 10% of the total volume. The components are mostly angular, detrital quartz grains with subordinate occurrences of muscovite and minor pyrite. X-ray diffraction patterns further revealed the presence of minor dolomite and ferroan dolomite. Microfacies 2 contains poorly sorted, angular quartz grains with subordinate dolomite and muscovite, as well as phosphatic minerals and large phosphatic concretions (Fig. 5). Microfacies 2 contains radiolarians and subordinate conodonts, and the portion of rock fragments and quartz components is estimated at 20–30 vol.%. The phosphatic concretions occur as spherical, oval and elongated forms that are between 1 and 4 cm in size. The phosphatic concretions can be distinguished in two different types. The first type of these concretions is oval to spherical and exhibits well-defined contours at their margins. These concretions contain a matrix of cryptocrystalline carbonate fluorapatite, as well as poorly preserved radiolarians that occupy an estimated 5% of the concretion volume. The second type of concretions grew around the first type of concretions exhibiting less well-defined contours at their margins (Fig. 5) and may reach up to 8 cm in diameter. The texture, fabric and mineral content of these concretions are similar to that of microfacies 2, but they contain more carbonate fluorapatite compared to quartz.
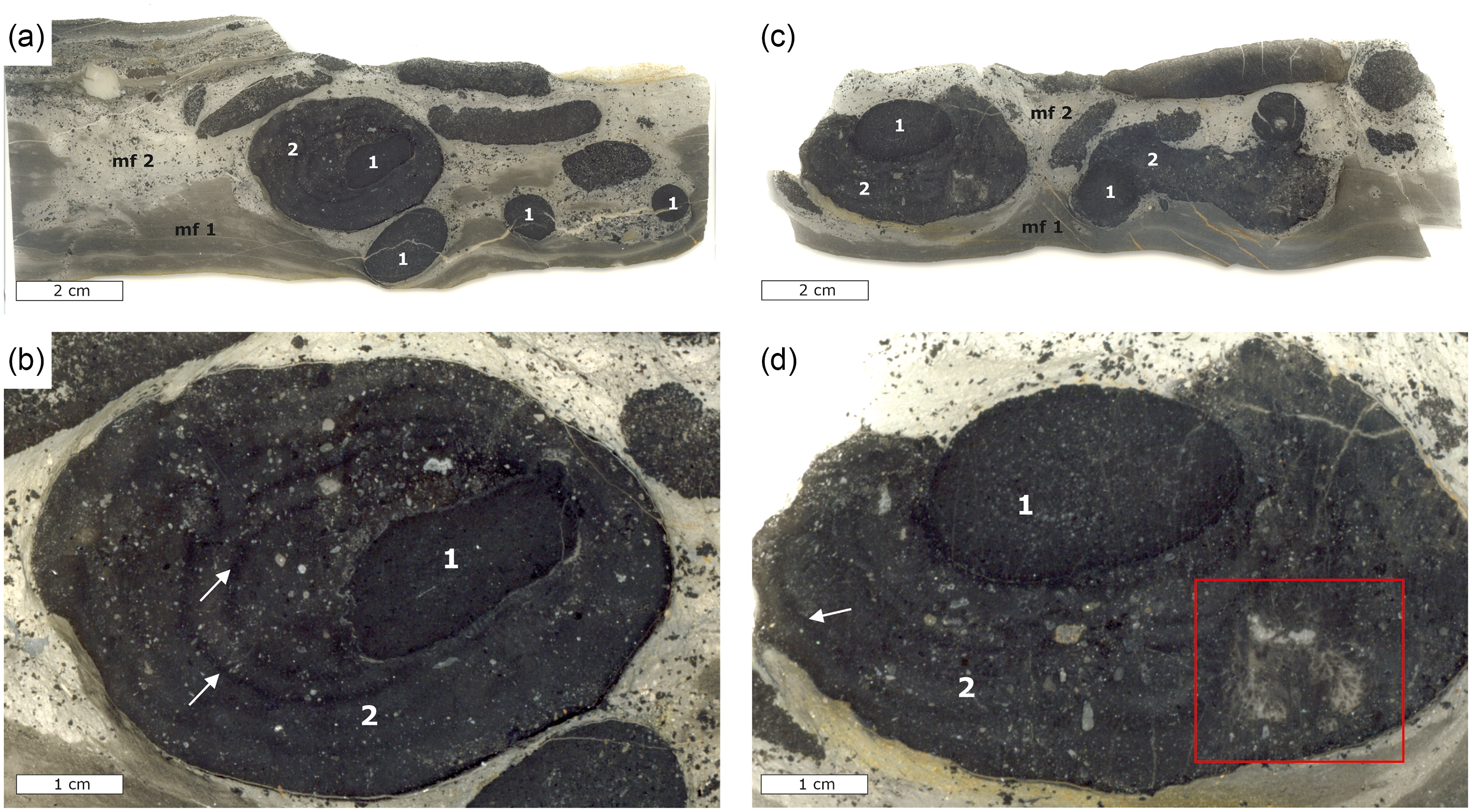
Figure 5. Thin section scans and photomicrographs of phosphatic concretions from bed 5g of the Lower Alum Black Shale; mf = microfacies, 1 = first-generation concretion, 2 = second-generation concretion. (a) Thin section scan showing two microfacies of background sediment with numerous smaller spherical, oval and elongated concretions and a large concretion floating within microfacies 2. (b) Close-up view of the larger phosphorous concretion in (a) with the first- and second-generation concretions, whereby the latter completely surrounds the former. White arrows show growth rims in the second-generation concretion. (c) Thin section scan showing microfacies 1 and 2 with numerous smaller concretions and a large concretion floating within microfacies 2. (d) Detailed view of the large concretion in (c) showing the second-generation concretion not completely surrounding the first-generation concretion. Arrows denoting a vague laminated growth pattern in the outer concretion, and the red rectangle highlighting a large aggregate of columnar branching phosphatic microstromatolites within the second-generation concretion.
Phosphatic microstromatolites occur in two morphologies. The first type are aggregates of thin columns showing well-defined lamination (Fig. 6a, b), which measure up to 250 µm in diameter and reach 600 µm in length. Some columns are shorter and show a cauliflower-like morphology (Fig. 6b). Some of the microstromatolites of this type occur within the outer parts of phosphatic concretions (cf. Fig. 5d), where much of the space between individual columns is occupied by microcrystalline silica (Fig. 6a, b). The second type of microstromatolite corresponds to smaller aggregates within the outer concretions (Fig. 6c, d). This type of microstromatolite does not exhibit any columnar or branching morphologies, yet it shows well-defined lamination within bulbous aggregates. Both types of microstromatolites contain unevenly distributed, authigenic pyrite (Figs. 6, 7). Most common are columnar and branching microstromatolites attached to the outer rim of the inner concretion (cf. Fig. 7a, c, d), with some concretions completely encased by microstromatolites (Fig. 7a–d). The individual stromatolite columns show a fine lamination (Fig. 7e, f).

Figure 6. Photomicrographs of phosphatic microstromatolites; ps = phosphatic microstromatolite, ms = microcrystalline silica, cf = carbonate fluorapatite, py = pyrite. (a, b) Large aggregates of columnar branching microstromatolites floating within the second concretion with abundant microcrystalline silica between individual columns. (b) An aggregate of more bulbous, cauliflower-shaped microstromatolites with microcrystalline silica and pyrite (opaque minerals); arrow denoting a radiolarian test. (c) Small aggregates of microstromatolites floating within the phosphatic second-generation concretion, arrow denoting a smaller aggregate. (d) Close-up view of a microstromatolite aggregate exhibiting small-scale darker and lighter laminae; arrows denoting authigenic pyrite within the phosphatic microstromatolite.

Figure 7. Photomicrographs of columnar branched phosphatic microstromatolites attached to the first-generation concretion; 1 = first-generation concretion, 2 = second-generation concretion, cv = carbonate vein, cm = clay minerals, qtz = quartz grains. (a) Columnar microstromatolites attached to the first-generation concretion. (b) Columnar, branched microstromatolites attached to the first-generation concretion, arrow denoting pyrite aggregates. (c, d) Columnar microstromatolites among clay minerals from a carbonate vein on the surface of the first-generation concretion. (e, f) Close-up view of columnar microstromatolites showing fine alterations of darker and lighter laminae among dispersed clay minerals and pyrite.
Scanning electron microscopy confirms that the microstromatolites within the phosphatic concretions are composed predominantly of apatite revealing a light grey appearance distinct from purely siliciclastic components in backscatter imaging (Fig. 8). The alternating fine laminae within the microstromatolites contain different amounts of alumosilicates and microcrystalline silica. The columnar stromatolites attached to the inner concretion show varying degrees of siliciclastics (Fig. 8a, b) and engulf radiolarian tests that consist of silica (Figs. 8c, 9c). Discernible laminae are composed mainly of alumosilicates, whereas the bulk of the stromatolites is composed of phosphorus minerals (Fig. 9b–d). Pyrite aggregates are prominent in backscatter mode by their strong white reflection (Fig. 8c, d). Electron microprobe analyses revealed that authigenic pyrite occurs within the second-generation concretions and within phosphatic microstromatolites (Fig. 8c, d), yet no pyrite was detected in the first-generation concretions. Pyrite within the second-generation concretions shows iron and sulphur contents between 40.3 and 47.1 wt% and 44.5 and 54.1 wt%, respectively (compared to 47.0 and 47.4 wt% iron and 53.8 and 54.3 wt% sulphur in the standard material), with average contents of 44.7 wt% iron and 51.4 wt% sulphur. Pyrite contains accessory elements including arsenic (averaging 0.19 wt%), cobalt (averaging 0.19 wt%), nickel (averaging 0.63 wt%), copper (averaging 0.12 wt%) and zinc (averaging 0.03 wt%). Pyrite analysed within the phosphatic microstromatolites shows average iron and sulphur contents of 44.9 and 52.8 wt%, respectively. This pyrite contains accessory elements including arsenic (averaging 0.08 wt%), cobalt (averaging 0.09 wt%), nickel (averaging 0.9 wt%), copper (averaging 0.07 wt%) and zinc (averaging 0.01 wt%).

Figure 8. Scanning electron microscopy images of phosphatic microstromatolites; 1 = first-generation concretion, 2 = second-generation concretion, ps = phosphatic microstromatolite, ms = microcrystalline silica. (a) Bulbous microstromatolite projecting from the first-generation concretion outwards and showing thin dark laminae of mostly alumosilicates, as well as finely dispersed pyrite (white reflecting minerals). (b) Columnar microstromatolite attached to the first-generation concretion with more intense, frequent interlayering of alumosilicates. (c) Bulbous to cauliflower-shaped microstromatolite with little lamination; red rectangles denoting authigenic pyrite, arrows denoting radiolarian tests. (d) Apparently free-floating microstromatolite aggregate within the second-generation concretion among abundant microcrystalline silica; red rectangles highlighting larger pyrite aggregates within the microstromatolite and the second-generation concretion.

Figure 9. Scanning electron microscope element mapping; ps = phosphatic microstromatolite, 2 = second-generation concretion, P = phosphorus, Si = silicon, Al = aluminium. (a) Electron backscatter image of a phosphatic microstromatolite. (c–d) Element distribution map of a phosphatic microstromatolite attached to the first-generation concretion.
FTIR ATR spectra of the mineral composition of the outer concretion, inner concretion and the phosphatic microstromatolites (cf. Fig. 5d) were compared to spectra of pure carbonate-free fluorapatite from Durango, Mexico (Becker et al. Reference Becker, Hartenfels, Weyer and Kumpan2016), a carbonate fluorapatite from Limburg an der Lahn, Germany (RRUFF data base R050529; ca. 20% of the phosphate sites is substituted by carbonate; Downs, Reference Downs2006; Lafuente et al. Reference Lafuente, Downs, Yang, Stone, Armbruster and Danisi2015) and a calcite reference acquired from a piece of blackboard chalk (Fig. 10). The wavenumber region from 400 to 1,600 cm−1 was chosen because it displays all fundamental vibrations of the phosphate and carbonate anion groups (White, Reference White and Farmer1974; Böttcher et al. Reference Böttcher, Gehlken and Steele1997; Penel et al. Reference Penel, Leroy, Rey, Sombret, Huvenne and Bres1997). Regarding the exact peak positions, it must be emphasized that strong vibrational bands in ATR spectra are slightly red-shifted to lower wavenumbers due to the impact of the complex refractive index instead of pure absorption (Harrick, Reference Harrick1967). Whereas pure, carbonate-free fluorapatite shows only the overlapping stretching bands ν1 and ν3 (ca. 1,100 to 930 cm−1) and bending modes ν2 and ν4 (ca. 630 to 540, 470 cm−1) of the phosphate groups, with a flat baseline above 1,100 cm−1, the outer and inner concretion and the microstromatolites, as well as the carbonate fluorapatite from the RRUFF database shows additional characteristic bands at approximately 1,425 and 1,450 cm−1 (double-headed arrows in Fig. 10); the latter bands have been assigned to the anti-symmetric stretching vibrations ν3 of the carbonate group replacing phosphate (substitution type B) in the apatite structure (Hofmann, Reference Hofmann1997). The spectra of these three Drewer apatites also reveal minor vibrational bands (ν2, ν3, ν4) caused by the carbonate group, positioned at about 1,400, 860 and 710 cm−1, respectively (cf. White, Reference White and Farmer1974; Böttcher et al. Reference Böttcher, Gehlken and Steele1997). These results confirm the presence of carbonate groups in the structure of apatite from the Drewer concretions and microstromatolites.

Figure 10. Fourier-transform infrared (FTIR) attenuated total reflectance (ATR) spectra. Spectra of (1) first-generation concretion, (2) second-generation concretion, and (3) phosphatic microstromatolite, as well as blackboard chalk, carbonate-bearing fluorapatite (RRUFF database; Downs, Reference Downs2006; Lafuente et al. Reference Lafuente, Downs, Yang, Stone, Armbruster and Danisi2015), carbonate-free fluorapatite from Durango, Mexico (Becker et al. Reference Becker, Hartenfels, Weyer and Kumpan2016); broken lines and shaded areas indicate the positions and areas of certain vibrations of the anion groups. The two double arrows at ca 1,425 and 1,450 cm–1 indicate the anti-symmetric stretching ν3 modes of B-type carbonate groups in apatite. Spectra have been vertically normalized and offset for better visibility. See text for details.
The carbon stable isotope compositions of carbonate fluorapatite show some differences between the phosphatic microstromatolites, the phosphatic concretions and the host rock. δ13C values of phosphatic microstromatolites are lowest ranging between −3.3 and −2.2‰, whereas those of phosphatic concretions are less negative between −1.7 and −0.8‰ (Fig. 11). The δ13C values of the host rock are between −1.7 and −1.3‰. δ18O values follow a similar trend; the microstromatolites show the most negative values between −9.1 and −7.9‰, whereas those of the concretions and the host rock lie between −8.1 and −7.3‰. The δ18O values of the host rock fall into a narrower range between −7.8 and −7.5‰.

Figure 11. Cross plot showing carbon and oxygen stable isotope compositions of phosphate-associated carbonate (PAC) of the phosphatic microstromatolites, phosphatic concretions and the phosphatic microfacies 2 of the host rock.
5. Discussion
5.a. Phosphogenesis and concretion growth
The Lower Alum Black Shale represents a period of reduced carbonate and clastic sedimentation, as well as high degrees of eutrophication and marine organic productivity leading to anoxic conditions in the sediments and possibly in the bottom waters (Siegmund et al. Reference Siegmund, Trappe and Oschmann2002; Rakocinski et al. Reference Rakocinski, Ksiazak, Pisarzowska, Zaton and Aretz2023). These conditions are similar to present-day upwelling zones, where the lack of oxygen in sediments and bottom waters facilitates the accumulation of phosphorus released during organic matter remineralization (Lomnitz et al. Reference Lomnitz, Sommer, Dale, Löscher, Noffke, Wallmann and Hensen2016). The Lower Alum Shale Event has been interpreted as a global anoxic event leading to a severe regional extinction of many invertebrate groups (Becker, Reference Becker1992; Walliser, Reference Walliser and Walliser1996; Kaiser et al. Reference Kaiser, Becker, Steuber and Aboussalam2011) culminating in a sea-level highstand during the Mississippian (Kaiser et al. Reference Kaiser, Becker, Steuber and Aboussalam2011; Becker et al. Reference Becker, Hartenfels and Kaiser2021). The Lower Alum Shale Event is also represented by black shales and cherts in the Holy Cross Mountains in Poland and from the Lydiennes Formation in the Montagne Noire, southern France. These black shale deposits provide evidence for dysoxic to anoxic conditions in bottom waters, as well as photic zone euxinia (Rakocinski et al. Reference Rakocinski, Marynowski, Zaton and Filipak2021, Reference Rakocinski, Ksiazak, Pisarzowska, Zaton and Aretz2023), probably favoured by enhanced productivity in surface waters. Since the same environmental constraints are probably applicable to the area of the Drewer locality, it suggests that the prolonged sea-level highstand with eutrophication in the photic zone (cf. Siegmund et al. Reference Siegmund, Trappe and Oschmann2002) favoured phosphogenesis at Drewer. The scarcity or the lack of bioturbation in the black shales at Drewer (Siegmund et al. Reference Siegmund, Trappe and Oschmann2002; own observation) agrees with episodically anoxic bottom water conditions.
Phosphogenesis in black shales and other organic-rich deposits is commonly manifested in the form of laminated crusts, lithified hardgrounds and gravels, disseminated nodules and oval to spherical concretions (Glenn et al. Reference Glenn, Föllmi, Riggs, Baturin, Grimm, Trappe, Abed, Galli-Oliver, Garrison, Ilyin, Jehl, Rohrlich, Sadaqah, Schildowski, Sheldon and Siegmund1994; Scasso & Castro, Reference Scasso and Castro1999; Arning et al. Reference Arning, Lückge, Breuer, Gussone, Birgel and Peckmann2009a, Bradbury et al. Reference Bradbury, Vandeginste and John2015; Filek et al. Reference Filek, Homayer, Feichtinger, Berning, Pollerspöck, Zwicker, Smrzka, Peckmann, Kranner, Mandic, Reichenbacher, Kroh, Uchman, Roetzel and Harzhauser2021). Today, authigenic phosphate crusts, nodules and concretions precipitate in organic-rich sediments at shallow depth (Soudry, Reference Soudry, Riding and Awramik2000; Arning et al. Reference Arning, Lückge, Breuer, Gussone, Birgel and Peckmann2009a), deposited either below upwelling or oxygen minimum zones (Föllmi, Reference Föllmi1996; Arning et al. Reference Arning, Birgel, Schulz-Vogt, Holmkvist, Jørgensen, Larson and Peckmann2008). The depletion of oxygen is a critical factor because it favours the preservation of organic matter, which is required as a phosphorus source during phosphogenesis (Filippelli, Reference Filippelli2011). The Lower Alum Black Shale at Drewer exhibits two concretion types, which are interpreted as representing two different generations of growth (Fig. 5). The first generation is represented by spherical to oval phosphate aggregates, which are overgrown by a second generation that accreted a larger volume of phosphorite (Fig. 5c, d). The first generation of phosphatic concretions grew in organic-rich sediments corresponding to microfacies 1 and were possibly exposed by winnowing. After their exposure, the phosphatic concretions were apparently transported from their formation site into sediments corresponding to microfacies 2. The composition of first-generation concretions differs from microfacies 2, which also suggests that they did not grow in microfacies 2 sediments but were redeposited before the second generation of concretions formed. Different formation conditions for the two generations of concretions are further indicated by the absence of authigenic pyrite within the first-generation concretions, while pyrite is abundant within the second-generation concretions and in the microstromatolites. In contrast to the first-generation concretions, second-generation concretions incorporated more non-phosphate minerals including clay minerals during growth (Figs. 5, 8, 9). These non-phosphate minerals were most likely incorporated from microfacies 2, suggesting that the second-generation concretions grew in situ within this microfacies. In addition, the second-generation concretions exhibit several growth rims that suggest multiple phases of concretion growth within microfacies 2 sediments (Fig. 5b, d). The presence of pyrite dispersed within the second-generation concretion (Fig. 8d) suggests that conditions remained sulphidic within the sediment during the growth of the phosphatic concretions within the Lower Alum Black Shale at Drewer.
5.b. Phosphatic microstromatolites – Palaeoenvironment and palaeoecology
Three possible mechanisms for microbial phosphorus accumulation include the remineralization of organic matter by sulphate-reducing bacteria, the release of internally stored (poly)phosphate by giant chemotrophic sulphide-oxidizing bacteria and the reductive dissolution of iron oxides that release adsorbed phosphorus (Froelich, Reference Froelich1988; Schulz & Schulz, Reference Schulz and Schulz2005; Arning et al. Reference Arning, Lückge, Breuer, Gussone, Birgel and Peckmann2009a; Berndmeyer et al. Reference Berndmeyer, Birgel, Brunner, Wehrmann, Jöns, Bach, Arning, Föllmi and Peckmann2012). Microbial sulphate reduction is the quantitatively dominant anaerobic process in the remineralization of organic matter in modern continental margin sediments (Ferdelman et al. Reference Ferdelman, Fossing and Neumann1999; Brüchert et al. Reference Brüchert, Jørgensen, Neumann, Riechmann, Schlösser and Schulz2003). Sulphate reduction is considered as a main cause of liberating phosphorus from organic matter, enabling the formation of phosphorite crusts and nodules in modern upwelling sediments (Arning et al. Reference Arning, Lückge, Breuer, Gussone, Birgel and Peckmann2009a, Reference Arning, Birgel, Brunner and Peckmann2009b). The Lower Alum Black Shale would have provided much organic matter for sulphate-reducing bacteria to release dissolved phosphorus to sedimentary pore water. The distribution, abundance and morphology of authigenic pyrite are further indicators for sulphate reduction in ancient phosphate-rich deposits (Wilkin et al. Reference Wilkin, Barnes and Brantley1996; Cosmidis et al. Reference Cosmidis, Benzerara, Menguy and Arning2013; Rickard, Reference Rickard2021). Pyrite is abundant within the second-generation phosphatic concretions and within the microstromatolites (Figs. 8a, c, d, 9f). The remineralization of organic matter and phosphorus release by sulphate reduction probably contributed substantially to phosphogenesis in the organic-rich sediments at Drewer, suggesting that phosphogenesis during early diagenesis was the primary process during growth of the second-generation concretions and microstromatolites.
Phosphorus-rich deposits in modern upwelling regions provide habitats for giant chemotrophic sulphide-oxidizing bacteria (Schulz & Schulz, Reference Schulz and Schulz2005; Arning et al. Reference Arning, Birgel, Schulz-Vogt, Holmkvist, Jørgensen, Larson and Peckmann2008; Crosby & Bailey, Reference Crosby and Bailey2012). The release of phosphate from internally stored (poly)phosphate compounds to pore waters has been demonstrated for the genera Beggiatoa and Thiomargarita, which can act as microbial drivers of phosphogenesis in organic-rich sediments (Schulz & Schulz, Reference Schulz and Schulz2005; Goldhammer et al. Reference Goldhammer, Brüchert, Ferdelman and Zabel2010). Studies on ancient phosphate-rich deposits suggest that sulphide-oxidizing bacteria similar to present-day Thiomargarita and Beggiatoa existed since the Proterozoic (Bailey et al. Reference Bailey, Joye, Kalanetra, Flood and Corsetti2007; Lepland et al. Reference Lepland, Joosu, Kirsimäe, Prave, Romashkin, Crne, Martin, Fallick, Somelar, Ürpraus, Mänd, Roberts, van Zuilen, Wirth and Schreiber2013; Czaja et al. Reference Czaja, Beukes and Osterhout2016); these chemotrophic bacteria are known to dwell in specific horizons at the redox boundary or in narrow zones at the sediment–water interface, contributing to the formation of phosphorite laminites and crusts on the seafloor (Jørgensen & Revsbach, Reference Jørgensen and Revsbach1983; Arning et al. Reference Arning, Birgel, Schulz-Vogt, Holmkvist, Jørgensen, Larson and Peckmann2008, Reference Arning, Lückge, Breuer, Gussone, Birgel and Peckmann2009a). Although evidence of the involvement of sulphide-oxidizing bacteria in phosphogenesis at Drewer is lacking, their possible contribution cannot be excluded.
An alternative microbial consortium that could have formed the phosphatic microstromatolites are microorganisms forming Frutexites, which are laminated, arborescent ferruginous or manganiferous microstromatolites that form in low-energy environments typified by low sedimentation rates and limited oxygen availability (Reitner et al. Reference Reitner, Wilmsen and Neuweiler1995; Woods & Baud, Reference Woods and Baud2008; Lazar et al. Reference Lazar, Gradinaru and Petrescu2013). Although it is not entirely clear which microbial consortia are involved in the formation of ancient Frutexites, iron enrichment appears to be a primary feature leading to the interpretation that iron-oxidizing bacteria in conjunction with either nitrate or sulphate reduction are key players in their development (Jakubowicz et al. Reference Jakubowicz, Belka and Berkowski2014; Heim et al. Reference Heim, Quéric, Ionescu, Schäfer and Reitner2017). Many Frutexites have been interpreted as stromatolites dwelling in deep-water or cryptic habitats that show no preferred phototactic growth direction (Böhm & Brachert, Reference Böhm and Brachert1993; Gischler et al. Reference Gischler, Fuchs, Bach and Reitner2021). Although the morphology and size of the Drewer microstromatolites are similar to reported Frutexites, the lack of iron oxides in the samples and the formation environment of the concretions argues against this interpretation (cf. Crosby et al. Reference Crosby, Bailey and Sharma2014). If bottom and pore waters were indeed anoxic to sulphidic at Drewer as suggested by trace element analyses (Siegmund et al. Reference Siegmund, Trappe and Oschmann2002; Becker et al. Reference Becker, Hartenfels, Weyer and Kumpan2016) and by the presence of pyrite, an accumulative mechanism by the formation and subsequent dissolution of iron oxides would be difficult to achieve and attain, although this may depend on the intensity and expansion of reducing bottom water conditions (Dellwig et al. Reference Dellwig, Leipe, März, Glockzin, Pollehne, Schnetger, Yakushev, Böttcher and Brumsack2010).
5.c. Stable isotopes and a scenario of microstromatolite and concretion formation
The δ13CPAC values of the phosphatic microstromatolites between −3.5 and −2‰ (Fig. 11) probably do not record early- to mid-Tournaisian seawater dissolved inorganic carbon (DIC). Carbon isotope stratigraphy from the Devonian–Carboniferous boundary suggests high rates of organic carbon burial and drawdown of atmospheric carbon dioxide as evidenced by positive δ13C excursions across this interval from localities in Europe, China and the USA (Buggisch & Joachimski, Reference Buggisch and Joachimski2006; Myrow et al. Reference Myrow, Strauss, Creveling, Sicard, Ripperdan, Sandberg and Hartenfels2011; Kumpan et al. Reference Kumpan, Babek, Kalvoda, Matys Grygar and Fryda2014; Kaiser et al. Reference Kaiser, Aretz, Becker, Becker, Königshof and Brett2015; Qie et al. Reference Qie, Liu, Wang, Mii, Zhang, Huang, Yao, Algeo and Luo2015). These excursions show a variety of δ13C peaks between +2 and +6‰ and are difficult to correlate globally as they differ between various regions and basins, which makes a reliable reconstruction of the carbon isotope composition of global ocean DIC difficult (Kaiser et al. Reference Kaiser, Aretz, Becker, Becker, Königshof and Brett2015; Paschall et al. Reference Paschall, Carmichael, Königshof, Waters, Ta Hoa, Komatsu and Dombrowski2019; Barnes et al. Reference Barnes, Husson and Peters2020). A lack of positive δ13C excursions during the Middle and Late Devonian of the Drewer section was noted by Buggisch & Joachimski (Reference Buggisch and Joachimski2006), who attributed this lack to a stratigraphic gap where no carbonates were deposited. Kumpan et al. (Reference Kumpan, Babek, Kalvoda, Grygar, Fryda, Becker and Hartenfels2015) investigated the δ13C record at Drewer, yet concluded that diagenetic alteration had compromised any reliable δ13C record of seawater. However, a positive δ13C excursion was documented in the Hasselbachgraben section near Oberrödinghausen, which is in the vicinity of the Drewer locality (Kaiser et al. Reference Kaiser, Steuber, Becker and Joachimski2006). These δ13C excursions were assigned to the Hangenberg Shale Event and interpreted as a sea-level highstand at the peak of the Hangenberg crisis (Kaiser et al. Reference Kaiser, Steuber, Becker and Joachimski2006). Although chemostratigraphic data directly related to the Lower Alum Shale Event are sparse (e.g. Saltzman et al. Reference Saltzman, Groessens and Zhuravlev2004), Buggisch et al. (Reference Buggisch, Joachimski, Sevastopulo and Morrow2008) reported a positive carbon isotope excursion during this event from coeval sections across Europe, reporting δ13Ccarbonate values as high as +5‰.
The δ13C composition of seawater DIC during the Late Devonian Hangenberg Shale Event until and after the Lower Alum Shale Event was strongly influenced by positive δ13C excursions reflecting perturbations in the global carbon cycle. Moreover, carbonate δ13C values from the latest Devonian through to the mid-Tournaisian never dropped below 0‰, which is distinct from δ13C values determined for the phosphatic stromatolites and concretions at Drewer (cf. Fig. 11). The δ13C values of the Drewer phosphatic microstromatolites are more negative compared to the δ13C value of Early Carboniferous seawater DIC (Saltzman et al. Reference Saltzman, Groessens and Zhuravlev2004; Buggisch et al. Reference Buggisch, Joachimski, Sevastopulo and Morrow2008). Moreover, the δ13C values of phosphatic microstromatolites are also more negative than δ13C values of the phosphatic concretions and the host material of microfacies 2 (Fig. 11). Inorganic carbon incorporated by phosphate minerals during phosphogenesis was likely a mixture of seawater DIC and DIC that was formed during the anaerobic microbial remineralization of organic matter in the sediment.
The lower δ13C values of the Drewer microstromatolites compared to phosphatic concretions and host rock suggest that the metabolism of the mat-forming microorganisms may have been responsible for local fractionation of stable carbon isotopes within the microbial mats that formed the microstromatolites. All δ13C values are substantially lower than what would be expected by fractionation during Calvin cycle carbon fixation mediated by oxygenic phototrophic microbial communities (O’Leary, Reference O’Leary1988; Laws et al. Reference Laws, Popp, Bidigare, Kennicutt and Macko1995). Although a covariance of δ13C and δ18O values of microstromatolites (r 2 = 0.94) and the phosphatic concretions (r 2 = 0.97) may argue for some degree of diagenetic overprint with meteoric waters during burial diagenesis (cf. Brand & Veizer, Reference Brand and Veizer1981; Banner & Hanson, Reference Banner and Hanson1990; Heydari et al. Reference Heydari, Wade and Hassanzadeh2001; Tong et al. Reference Tong, Wang, Peckmann, Cao, Chen, Zhou and Chen2016), the δ13C values of the microstromatolites are still more negative than those determined for the phosphorous concretions and the host rock, suggesting that the signal of microbial fractionation has been preserved to some degree. Moreover, environmental perturbations related to climatic warming may cause shifts of δ13C and δ18O to more negative values as observed for the Paleocene – Eocene Thermal Maximum and several oceanic anoxic events (Zachos et al. Reference Zachos, Schoutefn, Bohaty, Sluijs, Brinkhuis, Gibbs, Bralower and Quattlebaum2006; Ullmann et al. Reference Ullmann, Thibault, Ruhl, Hesselbo and Korte2014). Therefore, the observed covariance between δ13C and δ18O values of the phosphatic concretions and microstromatolites may additionally be impacted by climatic warming, elevated organic matter burial and increasing seawater temperatures from the late Famennian to the early Tournaisian (cf. Kaiser et al. Reference Kaiser, Steuber and Becker2008). Based on the temperature dependence of oxygen isotope fractionation between carbonate minerals, carbonate-bearing apatite and water (O’Neil et al. Reference O’Neil, Clayton and Mayeda1969; Loeffler et al. Reference Loeffler, Fiebig, Mulch, Tütken, Schmitt, Bajnai, Conrad, Wacker and Böttcher2019) and neglecting site-specific isotope fractionation (Zheng, Reference Zheng2016; Aufort et al. Reference Aufort, Segalen, Gervais, Paulatto, Blanchard and Balan2017), the observed maximum isotope variation (Fig. 11) would indicate a temperature rise by up to 8 and 11°C. This seems to be unrealistically high, therefore, at least parts of the oxygen isotope shift may be caused by a lowering in the oxygen isotope composition and therefore salinity of the pore water. Taken together, it is likely that the δ13C values of the phosphatic microstromatolites do not reflect ambient seawater composition, suggesting that the incorporated carbon within the carbonate fluorapatite reflects microbial fractionation related to a non-phototrophic metabolism.
The phosphatic concretions formed in anoxic sediments with the oxic–anoxic interface likely present in the water column in a regime of low water energy and low sedimentation rates. Some first-generation concretions are completely surrounded by the phosphatic microstromatolites (Figs. 5c, d, 6a, c, d), with the concretions as a substrate for the growth of stromatolite-forming microbial mats. The growth direction of the microbial mats was highly variable, showing no unidirectional upward growth direction as would be expected for phototactic growth as observed for photosynthesis-based cyanobacterial stromatolites (Allwood et al. Reference Allwood, Walter, Kamber, Marshall and Burch2006, Reference Allwood, Walter, Burch and Kamber2007). Interestingly, chemotrophic microbial mats growing around carbonate concretions have also been observed at methane seeps in the Black Sea (Reitner et al. Reference Reitner, Peckmann, Blumenberg, Michaelis, Reimer and Thiel2005). There, the mat-forming methanotrophic archaea and sulphate-reducing bacteria form thin layers around the concretions, showing a growth mode similar to the Drewer microstromatolites. It cannot be ruled out that some of the microstromatolites formed during reworking of the concretions at the sediment water interface, yet it is unlikely that the delicate branching stromatolites (Fig. 7) would have been preserved during physical reworking, sedimentation and burial of the concretions. The envisaged scenario of microstromatolite formation on phosphatic concretions is depicted schematically in Fig. 12. The first-generation concretions formed within deep-water anoxic, organic carbon-rich sediments during the global sea-level highstand of the Lower Alum Shale Event. The high content of organic matter and anoxic conditions allowed for the accumulation of phosphorus and the formation of phosphatic concretions (Fig. 12a). These first-generation concretions were subsequently moved from their original locus of formation, either by winnowing, bottom currents, or mass wasting and transported into a host sediment consisting of microfacies 2 sediments (Figs. 5, 12b). The phosphatic concretions were then colonized within the soft and porous sediment by microbial mats, probably including sulphate-reducing bacteria. These bacterial mats used the first-generation concretions as templates for biofilm attachment, growing on all sides of the concretions by displacive growth, pushing the soft surrounding sediments outwards, away from the concretion (Fig. 12c). The stromatolite-forming microbial mats showed no preferred growth direction that would suggest phototactic growth, which is in line with the interpretation that the phosphatic concretions and the microstromatolites formed within the sediments. The metabolic activity of sulphate-reducing bacteria gradually shifted the environmental conditions in the sediments from anoxic to sulphidic during a second phase of phosphatic concretion growth (Fig. 12d), as suggested by the occurrence of pyrite within the second-generation concretions (Fig. 8a, d).
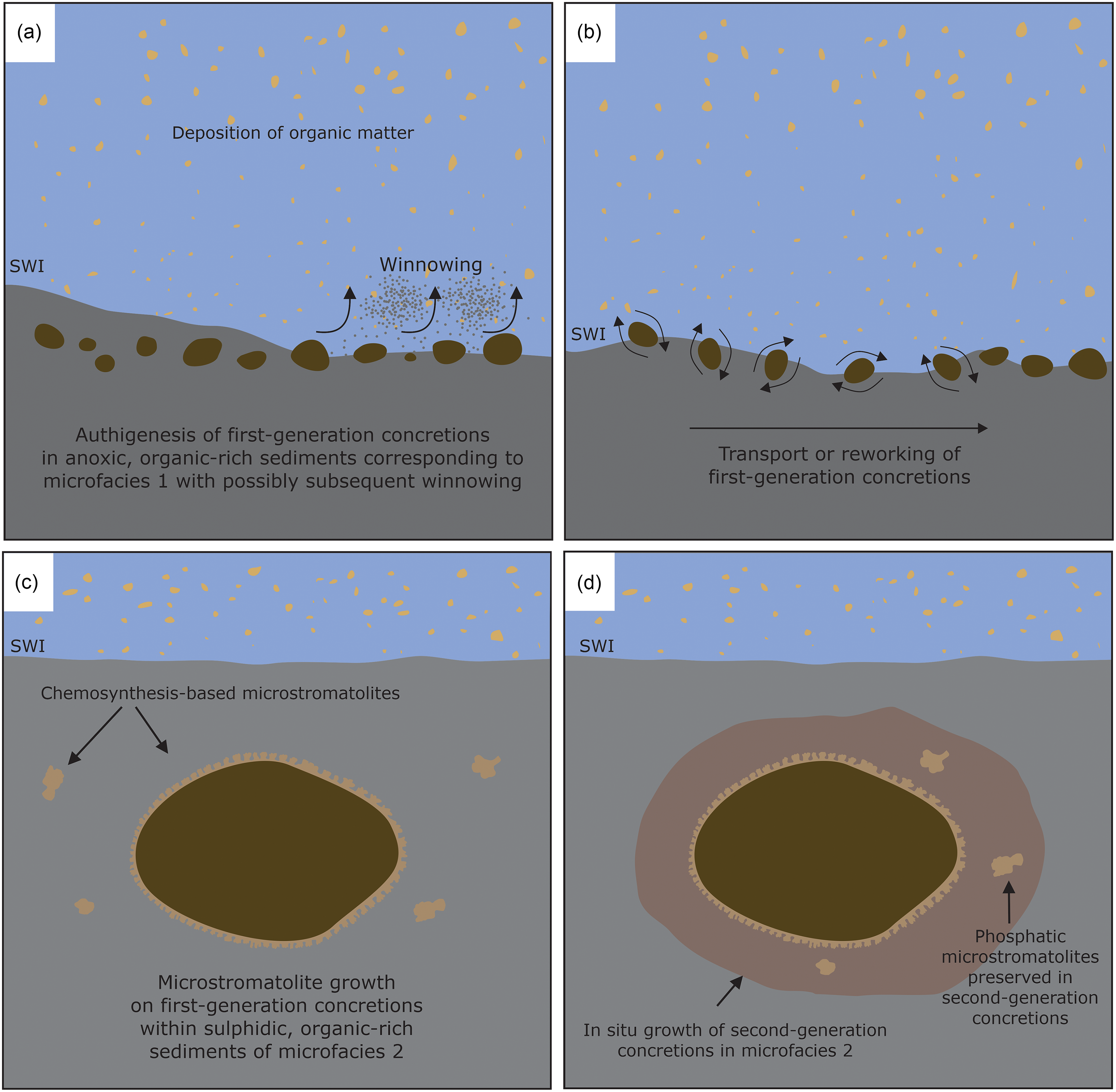
Figure 12. Schematic cartoon illustrating the formation of phosphatic concretions and phosphatic microstromatolites at Drewer, SWI = sediment–water interface. (a) Autochthonous formation of first-generation concretions in anoxic, organic-rich sediments. (b) Transport and redeposition of first-generation concretions. (c) Growth of phosphatic microstromatolites on first-generation concretions within sulphidic sediments. (d) Formation of second-generation concretions around first-generation concretions during sulphidic conditions in the sediment.
6. Conclusions
The Lower Alum Shale Event is archived within black shales deposited at the base of the Carboniferous, cropping out in the Rhenish Massif at Drewer. These black shales are rich in phosphate minerals, specifically apatite, and contain abundant phosphatic concretions that grew in two generations under anoxic to sulphidic conditions within the sediments during a sea-level highstand. The phosphatic concretions also served as a substrate for phosphatic microstromatolites, which are present in various morphologies including branched and cauliflower-shaped types attached to the first-generation concretions, as well as individual aggregates present within the second-generation concretions. Carbon stable isotope analyses of PAC derived from the phosphatic microstromatolites reveal low δ13C values compared to dissolved inorganic carbon of Early Carboniferous seawater, possibly pointing to a chemotrophic metabolism of the mat-forming microorganisms. Together with the inferred environmental conditions during black shale deposition and the formation of phosphatic concretions, these results suggest that the stromatolite-forming microbial mats thrived in an aphotic environment. Stromatolites through Earth’s history have mostly been interpreted as being the remnants of photosynthetic cyanobacteria, and therefore seen as indicators for shallow water environments. This study, however, shows that microbial communities forming stromatolites can also inhabit deep-water settings, and that the mat-forming microorganisms do not necessarily depend on photosynthesis as primary metabolic pathway. The reconstruction of environmental conditions and microbial metabolisms during stromatolite growth should therefore always regard chemotrophy as a feasible alternative to the common interpretation of stromatolites formed by cyanobacteria in shallow water settings within the photic zone.
Acknowledgements
We thank Esther T. Arning (Potsdam, Germany) for help with the collection of samples during a first field campaign and Christoph Vogt (Bremen, Germany) for XRD analyses. Experimental and method development work on CHAP was supported by DFG to MEB during the EXCALIBOR project. Comments of two anonymous reviewers helped to improve the manuscript.
Competing interests
There is no conflict of interest to report.