1. Introduction
Rangeomorphs were conspicuous members of the Ediacaran biosphere, present from roughly the end of the Gaskiers glaciation through to the beginning of the Cambrian period (c. 575–540 Ma). As the first substantial instances of large complex organisms in the fossil record, they mark a key transition in geobiological history, presaging the first appearance of unambiguous animals at c. 555 Ma. Even so, there is little consensus on the phylogenetic affiliations of rangeomorphs, with interpretations ranging from total-group cnidarians, ctenophores or sponges, to stem-group (eu)metazoans, or possibly an entirely unrelated lineage of multicellular macroscopic eukaryotes (Xiao & Laflamme, Reference Xiao and Laflamme2009). The problem with these particular fossils is not just their taxonomic placement, however, but a fundamentally deeper lack of understanding of how they were constructed, and how they worked as organisms.
Physiologically, macroscopic organisms work in much the same way as their microscopic counterparts, but with the added allometric challenges of conveying resources to internalized tissues and supporting the associated mass. Because of the fundamental reductions of surface area to volume (SA:V) that accompany increased body size, the evolution of large three-dimensional (3D) organisms necessarily involved major anatomical innovations. Even at modern levels of oxygen, for example, the maximum diameter of aerobic organisms lacking some sort of differentiated circulatory or respiratory apparatus is less than 2 mm (Catling et al. Reference Catling, Glein, Zahnle and McKay2005). There are, however, significant advantages to large body size, not least environmental buffering, systematic decreases in mass-specific metabolic demand (Glazier, Reference Glazier2006; DeLong et al. Reference DeLong, Okie, Moses, Sibly and Brown2010), and the emergence of scale-dependent mechanical, chemical and hydrodynamic properties (Sebens, Reference Sebens1987; Koehl, Reference Koehl1996; Hurd, Reference Hurd2000; Solari et al. Reference Solari, Kessler and Goldstein2007; Guizien & Ghisalberti, Reference Guizien, Ghisalberti, Rossi, Bramanti, Gori and Orejas Saco del Valle2016). Simply as a consequence of length scale (L) and background fluid velocity (U), for example, large organisms operate in a world of elevated Reynolds numbers where movement is dominated by inertial rather than viscous forces (Re = UL/v), and at elevated Péclet numbers where material exchange is dominated by advection rather than molecular diffusion (Pe = UL/D) (where v is kinematic viscosity and D is rate of diffusion). Such dynamics offer fundamentally enhanced levels of motility, feeding and gas exchange to macroscopic organisms (Butterfield, Reference Butterfield2018), provided the underlying issues of construction and resource distribution can be addressed.
Rangeomorphs are characterized by a broadly frond-like habit, built around a centimetre-scale branching element that exhibits self-similarity over three or four ‘fractal’ levels (Narbonne, Reference Narbonne2004; Brasier et al. Reference Brasier, Antcliffe and Liu2012; Hoyal Cuthill & Conway Morris, Reference Hoyal Cuthill and Conway Morris2014). Various arrangements of these elements, often in concert with basal holdfasts, elevating stalks or interconnecting rods, gave rise to a significant range of larger-scale forms including bi-terminal recliners (e.g. Fractofusus), unstalked unifoliate fronds (e.g. Charnia), stalked unifoliate fronds (e.g. Avalofractus), unstalked multifoliate fronds (e.g. Bradgatia), stalked multifoliate fronds (e.g. Rangea) and compound fronds (e.g. Hapsidophyllas) (Fig. 1). Although originally interpreted as macroscopic algae (Ford, Reference Ford1958), the abundant in situ preservation of rangeomorphs in deeper-water strata of Avalonia has convincingly ruled out a photosynthetic habit (Wood et al. Reference Wood, Dalrymple, Narbonne, Gehling and Clapham2003); and although some forms look superficially like modern sea-pens, their distinct styles of construction and growth rule out any direct affiliation to extant octocoral cnidarians (Seilacher, Reference Seilacher1989; Antcliffe & Brasier, Reference Antcliffe and Brasier2007).
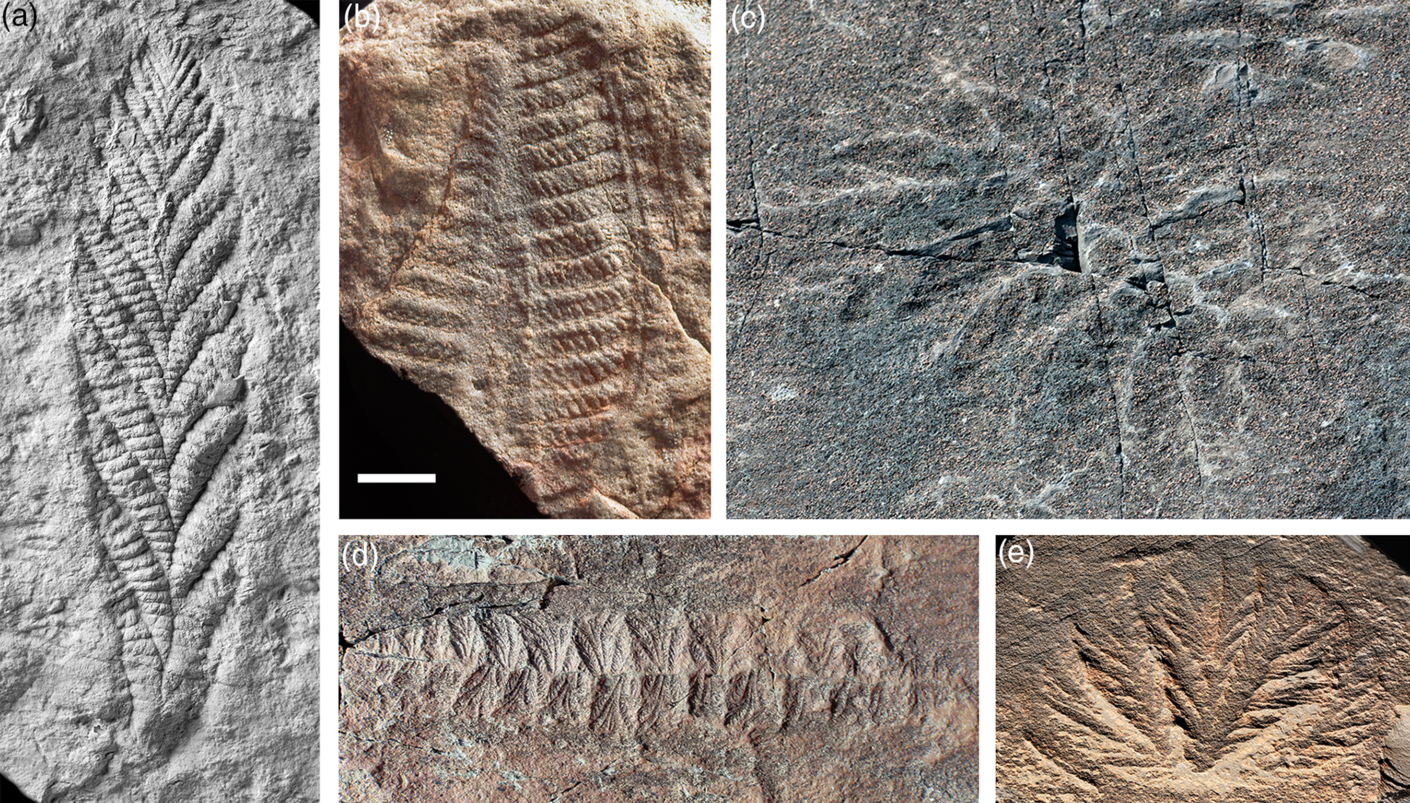
Fig. 1. Rangeomorph taxa illustrating the characteristic fractal-like branching and diversity of overall form. (a) Charnia masoni, type specimen, from Charnwood Forest, UK. (b) Rangea schneiderhoehni, type specimen, from Namibia. (c) Hapsidophyllas flexibilis, from SE Newfoundland. (d) Fractofusus misrae from SE Newfoundland. (e) Bradgatia sp. from SE Newfoundland. Scale bar: (a, e) 2 cm; (b) 1.5 cm; (c) 4 cm; and (d) 3 cm. Photo credits: (a) Phil Wilby; (b) Dima Grazhdankin; (c) Olga Zhaxybayeva; (d) Alex Liu; and (e) Jean-Bernard Caron.
Because of their anchored and generally elevated habit, rangeomorphs have traditionally been interpreted as microphagous suspension feeders, ecologically analogous to sponges or anthozoan cnidarians (Jenkins, Reference Jenkins1985). Unlike these living forms, however, rangeomorphs appear to lack tentacles, openings or any other feeding-related features, even in specimens preserving detail on a scale of tens of micrometres (Narbonne, Reference Narbonne2004). As such, it has been widely assumed that nutrient uptake took place on the outside of the organism, after the manner of osmotrophic bacteria or fungi (McMenamin, Reference McMenamin1993; Laflamme et al. Reference Laflamme, Xiao and Kowalewski2009; Sperling et al. Reference Sperling, Peterson and Laflamme2011). Certainly the characteristic ‘fractal’ branching would have increased the proportion of exposed surface area on which this might have occurred, but it remains to be demonstrated that rangeomorphs could actually feed in this fashion (Liu et al. Reference Liu, Kenchington and Mitchell2015).
1.a. Osmotrophy and dissolved organic carbon: a primer
The most immediate issue arising from the osmotrophy model for rangeomorphs is the imprecise, often inconsistent, use of the term itself. In its most general sense, osmotrophy is simply the process by which dissolved substrates are passed across cell membranes (Jumars et al. Reference Jumars, Deming, Hill, Karp-Boss, Yager and Dade1993; Karp-Boss et al. Reference Karp-Boss, Boss and Jumars1996; Thingstad et al. Reference Thingstad, Øvreås, Egge, Løvdal and Heldal2005). At least implicitly, it is limited to external, environmentally exposed surfaces, which usefully distinguishes it from otherwise similar processes of internalized uptake (e.g. in eumetazoan guts and the food vacuoles of phagocytizing protozoans). The textbook exemplars of osmotrophic feeding – heterotrophic prokaryotes and fungi – are also ‘external digesters’, where organisms actively excrete hydrolytic enzymes and recover the digested products. There are risks to this type of feeding, however, most obviously through the dispersive loss of exo-enzymes and product in aqueous environments, but also their exploitation by unrelated or non-contributing organisms (Jumars et al. Reference Jumars, Deming, Hill, Karp-Boss, Yager and Dade1993; Karp-Boss et al. Reference Karp-Boss, Boss and Jumars1996; Vetter et al. Reference Vetter, Deming, Jumars and Krieger-Brockett1998; Arnosti, Reference Arnosti2011; Richards & Talbot, Reference Richards and Talbot2013). The key to limiting such losses is containment. Fungi typically manage this through the penetration of solid substrates, whereas osmotrophic prokaryotes exploit the viscosity-dominated fluid dynamics associated with small length scales (<< Re). The diffusive boundary layer (DBL) surrounding micrometre- and sub-micrometre-sized cells is effectively impervious to advective loss, greatly facilitating the rate-limiting steps of both hydrolytic digestion and trans-membrane uptake (Jumars et al. Reference Jumars, Deming, Hill, Karp-Boss, Yager and Dade1993; Langlois et al. Reference Langlois, Andersen, Bohr, Visser, Fishwick and Kiorbøe2009; Arnosti, Reference Arnosti2011). Conversely, the DBL and its facilitation of external digestion and osmotrophy are progressively eroded at larger length scales. Indeed, the primary impediment to osmotrophic feeding in large organisms is not SA:V per se, but the challenge of digesting and incorporating substrate under elevated Re conditions.
The discussion of osmotrophy has been further confused by the term ‘dissolved organic carbon’ (DOC), the substrate on which rangeomorphs are assumed to have fed. Despite early proposals to limit the term to genuinely soluble components (Sharp, Reference Sharp1973), DOC has come to be defined operationally as the reduced carbon content of a filtered water sample, with the pore size of the filter ranging more or less arbitrarily from 0.2 to 1.0 μm (Verdugo et al. Reference Verdugo, Alldredge, Azam, Kirchman, Passow and Santschi2004). As such, DOC now includes a disparate range of materials, from fully dissolved molecules to colloids, gels, viruses and even small microbes.
Further classification of DOC is based on environmental longevity; on the one hand ‘labile DOC’ with residence times of hours to days, and on the other ‘recalcitrant DOC’, which persists from weeks to tens of thousands of years (Hansell, Reference Hansell2013; Follett et al. Reference Follett, Repeta, Rothman, Xu and Santinelli2014). In structural terms, labile DOC is represented by free monomers and small oligomers (<600 daltons) and is the only fraction available for direct osmotrophic uptake. Unsurprisingly, it has limited potential for environmental accumulation, with amino acids and sugars in the modern ocean occurring at concentrations of less than one-billionth of a gram per litre (Hansell, Reference Hansell2013; Moran et al. Reference Moran, Kujawinski, Stubbins, Fatland, Aluwihare, Buchan, Crump, Dorrestein, Dyhrman, Hess, Howe, Longnecker, Medeiros, Niggemann, Obernosterer, Repeta and Waldbauer2016). By contrast, the larger molecules and materials comprising recalcitrant DOC accumulate substantially in the oceans, collectively representing more than 200 times the carbon present in marine biomass. Even so, DOC remains conspicuously dilute in marine environments (from c. 34 to > 80 μmol kg−1), often falling below the threshold necessary to sustain microbially mediated hydrolysis (Arrieta et al. Reference Arrieta, Mayol, Hansman, Herndl, Dittmar and Duarte2015). This alone may contribute to its extended residence time.
There are multiple sinks for recalcitrant DOC in the modern oceans, including both biological remineralization and sedimentary adhesion leading to long-term burial. Of the former, a substantial fraction ends up being captured and consumed by suspension-feeding animals. Benthic sponges, for example, have an extraordinary capacity to extract colloidal DOC from large volumes of water (Yahel et al. Reference Yahel, Sharp, Marie, Häse and Genin2003; de Goeij et al. Reference de Goeij, van Oevelen, Vermeij, Osinga, Middelburg, de Goeij and Admiraal2013; Kahn et al. Reference Kahn, Yahel, Chu, Tunnicliffe and Leys2015), a habit emulated in the pelagic realm by actively swimming salps and tunicates (Flood et al. Reference Flood, Deibel and Morris1992; Sutherland et al. Reference Sutherland, Madin and Stocker2010). None of these DOC feeders can be considered osmotrophic, however, since all of the associated digestion and uptake takes place internally, by endocytic choanocytes in the case of sponges (Leys & Eerkes-Medrano, Reference Leys and Eerkes-Medrano2006) and within a differentiated gut in tunicates and other eumetazoans (e.g. Dishaw et al. Reference Dishaw, Cannon, Litman and Parker2014). At the same time, there is a proportion of DOC that physically aggregates to produce larger particulate organic carbon (POC), with the resulting flakes, gels and transparent exopolymer particles (TEP) available for consumption via conventional eumetazoan-grade capture and ingestion (Camilleri & Ribi, Reference Camilleri and Ribi1986; Verdugo et al. Reference Verdugo, Alldredge, Azam, Kirchman, Passow and Santschi2004; Mari et al. Reference Mari, Passow, Migon, Burd and Legendre2017).
Despite these various non-osmotrophic means of incorporating DOC, it is clear that a range of aquatic eukaryotes do exploit it directly. Labelling experiments, for example, have demonstrated the osmotrophic uptake of acetate, monosaccharides, amino acids and fatty acids by most major invertebrate clades (Wright & Manahan, Reference Wright and Manahan1989; Baines et al. Reference Baines, Fisher and Cole2005; Skikne et al. Reference Skikne, Sherlock and Robison2009; Gori et al. Reference Gori, Grover, Orejas, Sikorski and Ferrier-Pagès2014; Blewett & Goss, Reference Blewett and Goss2017). There are also reports of uncharacterized DOC uptake (e.g. Roditi et al. Reference Roditi, Fisher and Sanudo-Wilhelmy2000; Barnard et al. Reference Barnard, Martineau, Frenette, Dodson and Vincent2006; Rengefors et al. Reference Rengefors, Pålsson, Hansson and Heiberg2008), although it is notable that these substrates were all derived from fresh algal or arthropod lysates. In other words, the osmotrophy observed in modern eukaryotes and metazoans appears to be limited exclusively to the labile, low-molecular-weight DOC that requires no prior digestion; and even then, uptake rates are typically 2–4 orders of magnitude lower than those associated with internalized feeding (Wright & Manahan, Reference Wright and Manahan1989). Reports of aquatic invertebrates feeding osmotrophically on recalcitrant DOC (e.g. Mcmeans et al. Reference Mcmeans, Koussoroplis, Arts and Kainz2015) are likely to involve microbial intermediaries or other means of repackaging leading to internalized digestion (e.g. Camilleri & Ribi, Reference Camilleri and Ribi1986; Höss et al. Reference Höss, Bergtold, Haitzer, Traunspurger and Steinberg2001; Eckert & Pernthaler, Reference Eckert and Pernthaler2014).
Whatever the absolute quality or quantity of food, it only becomes metabolically available once it has been translocated from the external environment into a cell. Although O2, CO2 and a variety of small hydrophobic molecules diffuse more or less freely across phospholipid cell membranes, most organic molecules – including amino acids and monosaccharides – can only be taken up via membrane-embedded transporter proteins. As such, maximum uptake rates are determined by the density and specificity of transporters, and the non-trivial time required for substrate exchange (Confer & Logan, Reference Confer and Logan1991; Karp-Boss et al. Reference Karp-Boss, Boss and Jumars1996). Glucose and amino acid transporters, for example, will saturate under elevated substrate concentrations, limiting the utility of locally enhanced delivery. Even so, the vanishingly low concentrations of free monomers in the modern ocean means that the rate-limiting step for marine osmotrophy will almost always revert to the hydrolytic digestion of more recalcitrant DOC (Confer & Logan, Reference Confer and Logan1991; Moran et al. Reference Moran, Kujawinski, Stubbins, Fatland, Aluwihare, Buchan, Crump, Dorrestein, Dyhrman, Hess, Howe, Longnecker, Medeiros, Niggemann, Obernosterer, Repeta and Waldbauer2016), a process fundamentally at odds with fluid dynamics at macroscopic length scales.
Ultimately, of course, the challenge for all heterotrophic organisms is accessing food. For microbes operating under low Re and Pe regimes, the presence of a thick, essentially permanent DBL means that substrate delivery is dominated by molecular diffusion (Karp-Boss et al. Reference Karp-Boss, Boss and Jumars1996). Despite their extraordinary capacity to digest recalcitrant DOC, the physical inability of osmotrophic microbes to pump or swim substantially through water means they are prone to starvation under oligotrophic conditions (Arrieta et al. Reference Arrieta, Mayol, Hansman, Herndl, Dittmar and Duarte2015). Delivery can be dramatically enhanced in larger organisms simply through the accompanying inertial and turbulent fluid dynamics (Langlois et al. Reference Langlois, Andersen, Bohr, Visser, Fishwick and Kiorbøe2009; Singer et al. Reference Singer, Plotnik and Laflamme2012; Ghisalberti et al. Reference Ghisalberti, Gold, Laflamme, Clapham, Narbonne, Summons, Johnston and Jacobs2014; Guizien & Ghisalberti, Reference Guizien, Ghisalberti, Rossi, Bramanti, Gori and Orejas Saco del Valle2016; Butterfield, Reference Butterfield2018), but at the cost of eroding the stable DBL necessary for external digestion (Vetter et al. Reference Vetter, Deming, Jumars and Krieger-Brockett1998; Arnosti, Reference Arnosti2011; Richards & Talbot, Reference Richards and Talbot2013). In principle, then, macroscopic heterotrophs should be able to make a living under fundamentally more oligotrophic conditions than their microbial counterparts (Ghisalberti et al. Reference Ghisalberti, Gold, Laflamme, Clapham, Narbonne, Summons, Johnston and Jacobs2014), but only if there is an alternative means of digesting recalcitrant food.
2. Osmotrophic rangeomorphs?
Laflamme et al. (Reference Laflamme, Xiao and Kowalewski2009) have argued that Ediacaran rangeomorphs fed osmotrophically via their ‘fractally’ enhanced SA:V properties, noting a marginal overlap with some exceptionally large living prokaryotes. There are problems with this study, however, not least the choice of modern analogues. Of the eight ‘strictly osmotrophic megabacteria’ included in the analysis, only one is actually a free-living osmotroph: the relatively modest-sized (<15 μm diameter) archaeon Staphylothermus marinus. Two others are substantially larger, but known exclusively from the guts of aquatic vertebrates where neither substrate digestion nor advective loss are relevant factors (Sporospirillum praeclarum in tadpoles and Epulopiscium fishelsoni in surgeon fish). None of the remaining five taxa is osmotrophic, or even heterotrophic: Thiomargarita, Achromatium, Beggiatoa and Thiovulum are all sulphur-oxidizing chemoautotrophs, and Prochloron is a cyanobacterial photoautotroph. Indeed, the most likely explanation for the exceptionally large dimensions of these primary producers is the advective delivery of (freely diffusible) CO2 at larger length scales. In any event, there are no extant heterotrophic prokaryotes, free-living or otherwise, that fall within the calculated SA:V range of Ediacaran rangeomorphs (cf. Schulz & Jørgensen, Reference Schulz and Jørgensen2001; Laflamme et al. Reference Laflamme, Xiao and Kowalewski2009, fig. 4b).
The likelihood of osmotrophic feeding in rangeomorphs is also problematic in terms of metabolically available DOC. Although the Proterozoic oceans might well have contained high concentrations of total DOC (e.g. Shields, 2017), any substantial accumulations would have been chemically recalcitrant and unavailable for direct osmotrophic uptake. Arguments for the presence of abundant labile DOC in Proterozoic oceans – due to the absence of metazoan zooplankton and concomitant slow sinking of phytoplankton (Sperling et al. Reference Sperling, Peterson and Laflamme2011) – are incompatible with the voracious consumption of free monomers by heterotrophic microbes, particularly in well-oxygenated surface waters where almost all labile DOC is produced. Labile DOC might well have been intermittently elevated in the vicinity of Ediacaran rangeomorphs (Budd & Jensen, Reference Budd and Jensen2017), but never at the continuously concentrated levels enjoyed by gut-dwelling Sporospirillum and Epulopiscium (cf. Pollak & Montgomery, Reference Pollak and Montgomery1994; Schulz & Jørgensen, Reference Schulz and Jørgensen2001).
The most direct means of resolving the trade-off between effective food delivery (enhanced in larger organisms and elevated Re) and its follow-up incorporation (enhanced at low Re) is to separate the two processes. At a unicellular level, the contained intracellular digestion of POC and DOC by phagocytizing protists by-passes many of the fluid-dynamic challenges faced by osmotrophic prokaryotes, although new ones inevitably arise, not least the elevated Re and turbulence associated with larger eukaryotic cells, and their interference with effective prey capture (Dolan et al. Reference Dolan, Sall, Metcalfe and Gasser2003). At macroscopic length scales, phagocytosis becomes entirely untenable without anatomical or behavioural adaptations for attenuating flow, as seen in the ramifying aquiferous system of sponges (Leys & Eerkes-Medrano, Reference Leys and Eerkes-Medrano2006), the enveloping habit of placozoans (Smith et al. Reference Smith, Pivovarova and Reese2015) or the chambered, often channelized gastrodermal system of cnidarians (Southward, Reference Southward1955; Schick, Reference Schick1991; Harmata et al. Reference Harmata, Parrin, Morrison, McConnell, Bross and Blackstone2013; Raz-Bahat et al. Reference Raz-Bahat, Douek, Moiseeva, Peters and Rinkevich2017; Goldberg, Reference Goldberg, Kloc and Kubiak2018; Steinmetz, Reference Steinmetz2019). Such dynamics presumably account for the exclusively gastrodermal uptake of zooxanthellae in photoendosymbiotic anthozoans. In the case of rangeomorphs, furrows associated with the ‘fractally’ divided integument offer the only potential for comparable levels of isolation on the external surface, although these notably constitute just a fraction of the total surface area.
What the macroscopic size of rangeomorphs certainly does confer is elevated Reynolds and Péclet numbers. Ghisalberti et al. (Reference Ghisalberti, Gold, Laflamme, Clapham, Narbonne, Summons, Johnston and Jacobs2014) presciently recognized the fluid-dynamic implications of large size in Ediacaran macrofossils, noting that the turbulence generated by the interaction of physical currents and an elevated macrobenthos comprehensively overrides any diffusional limits on the delivery of dissolved and suspended resources. Although problematic in terms of osmotrophic feeding, such ‘canopy effects’ are directly applicable to freely diffusible O2 and CO2; indeed, all three of the datasets used by Ghisalberti et al. (Reference Ghisalberti, Gold, Laflamme, Clapham, Narbonne, Summons, Johnston and Jacobs2014, figs 3, S2) to illustrate this principle were specifically measures of oxygen transport. In this light, the most immediate advantage to rangeomorphs adopting a macroscopic habit was access to advective food delivery and gas exchange (Singer et al. Reference Singer, Plotnik and Laflamme2012). Additional ventilatory effects are likely to have been generated by a ciliated epithelium, allowing rangeomorphs to employ their external surface as a breathing device under both high-energy and relatively stagnant physical flow (cf. Short et al. Reference Short, Solari, Ganguly, Powers, Kessler and Goldstein2006; Shapiro et al. Reference Shapiro, Fernandez, Garren, Guasto, Debaillon-Vesque, Kramarsky-Winter, Vardi and Stocker2014; Cavalier-Smith, Reference Cavalier-Smith2017; Dufour & McIlroy, Reference Dufour and McIlroy2017).
2.a. The rangeomorph skeleton
Whatever the particular habits of Ediacaran macrofossils, they were clearly supported by some sort of skeletal superstructure, an anatomical feature that is likely to illuminate other aspects of their biology. In the absence of obvious biomineralization, this has been widely envisaged as a hydrostatic endoskeleton, comparable to the coelomic system of annelid worms (Runnegar, Reference Runnegar1982), the syncytia of giant ‘unicellular’ protists (Seilacher, Reference Seilacher1989, Reference Seilacher1992; Seilacher et al. Reference Seilacher, Grazhdankin and Legouta2003) or a sponge-grade mesenchyme-like mass (Dufour & McIlroy, Reference Dufour and McIlroy2017). More generally, Laflamme et al. (Reference Laflamme, Xiao and Kowalewski2009) have argued that ‘much of the internal body cavity… may have been filled by metabolically inactive material (inorganic, organic, or fluid).’
The key to resolving the nature of the rangeomorph skeleton lies in its taphonomic dissection. Although most rangeomorphs are preserved as more or less 2D bedding-plane imprints – a product of felling, degradational collapse and early diagenetic ‘death-mask’ cementation – there is a notable subset of specimens that have been preserved as conspicuously 3D casts and moulds (Jenkins, Reference Jenkins1985; Fedonkin, Reference Fedonkin and Bengtson1994; Dzik, Reference Dzik2002; Grazhdankin & Seilacher, Reference Grazhdankin and Seilacher2005; Vickers-Rich et al. Reference Vickers-Rich, Yu Ivantsov, Trusler, Narbonne, Hall, Wilson, Greentree, Fedonkin, Elliott, Hoffmann and Schneider2013; Sharp et al. Reference Sharp, Evans, Wilson and Vickers-Rich2017). Such sedimentary infilling points to the presence not only of large internalized chambers, but also chamber walls of sufficient integrity to act as the containing form. In Charnia specimens from the Winter Mountains of the White Sea, for example, substantial parts of the fronds have been infilled with silt early and rapidly enough to capture their full 3D profile (Fedonkin, Reference Fedonkin and Bengtson1994; Grazhdankin, Reference Grazhdankin2004; Dunn et al. Reference Dunn, Wilby, Kenchington, Grazhdankin, Donoghue and Liu2018), even as adjacent unfilled areas collapsed to yield a more typical 2D death-mask (Fig. 2).
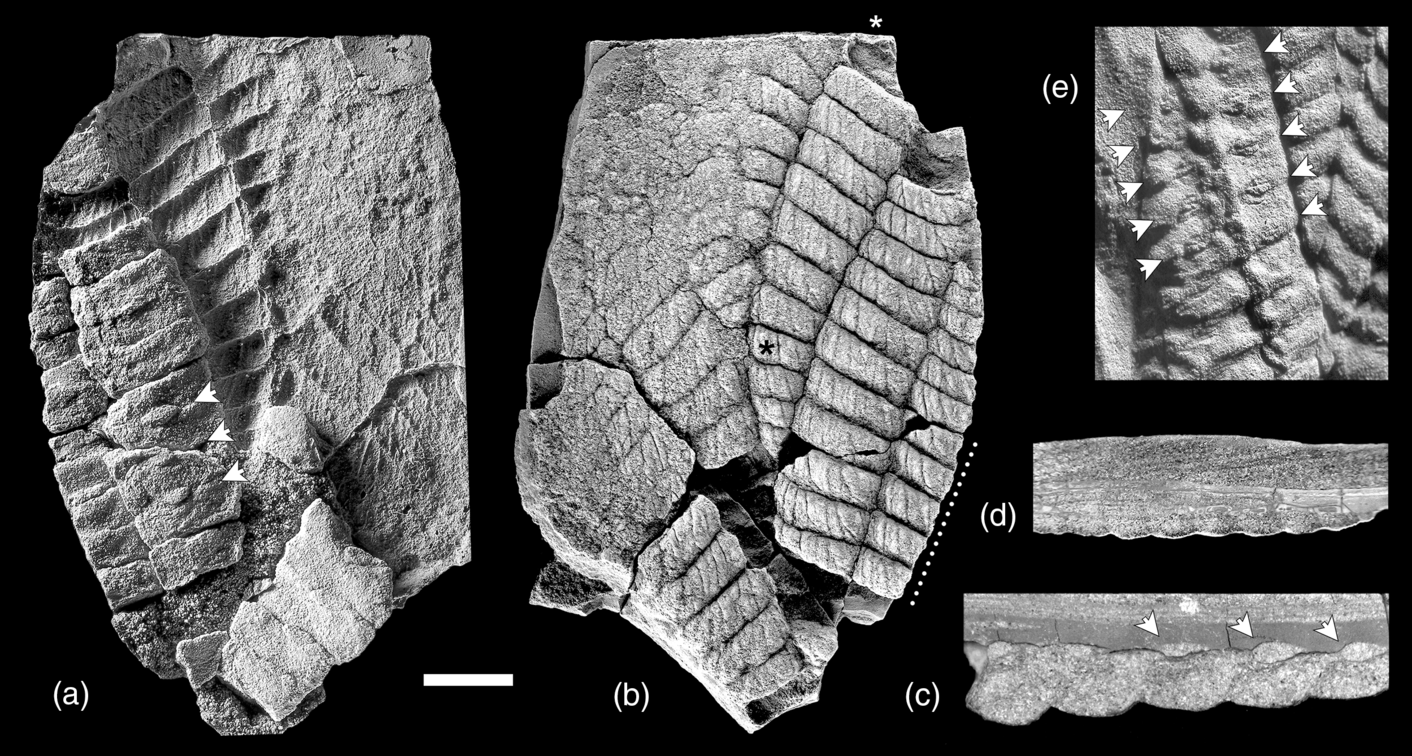
Fig. 2. Partially cast 3D specimens of Charnia from the Verkhovka Formation (Winter Mountains, White Sea, Russia), demonstrating the previous presence of water-filled chambers. (a, b) ‘Upper’ and ‘lower’ surfaces of PIN 3993-7018 (with differential transfer of the primary branch casts between the two parts); note that only some parts of this specimen have been infilled with sediment (roughly the left-hand side of (a) and the right-hand side of (b)), with the remainder experiencing a more typical ‘collapse and death-mask’ type of preservation. The three serially repeated lensoid structures preserved on the upper side of the cast (arrows in (a)) potentially represent openings into the chambers; they are not present on the lower ‘fractally’ divided side (b), and are not preserved in collapsed parts of the frond. (c) Cross-section through a silt-cast primary branch of PIN 3993-7018, locally buried in mud and showing anatomical continuity between the chambers and serially repeated lensoid structures (arrowed); line of section indicated by the dotted line in (b). (d) Cross-section through a silt-cast primary branch of PIN 3993-7018, locally buried in cross-laminated silt and showing clear evidence of erosive breaching and loss of the upper body wall; line of section indicated by asterisks in (b). (e) Detail of a further silt-cast, mud-buried specimen (PIN 3992-7020) preserving serially repeated lensoid structures on the upper, non-fractally divided surface (arrows); the full specimen is figured in Fedonkin (Reference Fedonkin and Bengtson1994). Scale bar: (a, b, e) 1 cm; (c) 2.5 mm; and (d) 5 mm. PIN – Palaeontological Institute, Moscow. Photo credits: (a, b, c, e) Dima Grazhdankin; and (d) Alex Liu.
Casting is a common mode of preservation in biomineralized or heavily lignified organisms, but is less expected in ‘soft-bodied’ Ediacaran forms (Seilacher, Reference Seilacher1970; Rex, Reference Rex1985; Retallack, Reference Retallack1994; Maeda et al. Reference Maeda, Kumagae, Matsuoka and Yamazaki2010). Where it does occur, the process will be similarly dependent on chambers with self-supporting walls, but proceeding on fundamentally shorter timescales. Given the rapidly collapsing 2D habit of rangeomorphs in general, it follows that the original contents of the chambers must have been correspondingly fluid. The effectively instantaneous casting of Charnia compartments (Fig. 2) is inconsistent with the original contents having the viscosity of syncytial cytoplasm (cf. Seilacher, Reference Seilacher1989, Reference Seilacher1992), mesenchyme (cf. Dufour & McIlroy, Reference Dufour and McIlroy2017) or coelomic fluids (cf. Runnegar, Reference Runnegar1982), particularly given the rapid wound-repair systems associated with these fully isolated hydrostatic skeletons (e.g. Menzel, Reference Menzel1988; Duckworth, Reference Duckworth2003; Kamran et al. Reference Kamran, Zellner, Kyriazes, Kraus, Reynier and Malamy2017). By far the most likely material filling the compartments of rangeomorphs – and conferring their primary skeletal support – is locally contained low-viscosity seawater.
In the absence of obvious openings in the body wall (Narbonne, Reference Narbonne2004), the route by which such water entered rangeomorph chambers has yet to be identified. One likely possibility is that the conduits were simply too small or ephemeral to fossilize under the associated taphonomic regimes. Even with the fundamentally greater levels of resolution seen in Burgess Shale-type preservation, for example, the ostia and associated aquiferous system of sponges have never been directly preserved (Butterfield, Reference Butterfield2003). The external openings of water-pumping siphonozooids in colonial octocorals can be similarly cryptic, even in living specimens (Fig. 3d) (Hickson, Reference Hickson1883; Brafield, Reference Brafield1969; Nonaka et al. Reference Nonaka, Nakamura, Tsukahara and Reimer2012; Williams et al. Reference Williams, Hoeksema and Van Ofwegen2012), and it is notable that the millimetre-sized siphonozooids of certain pennatulaceans fail to preserve even under the most optimized laboratory burial conditions (Norris, Reference Norris1989).
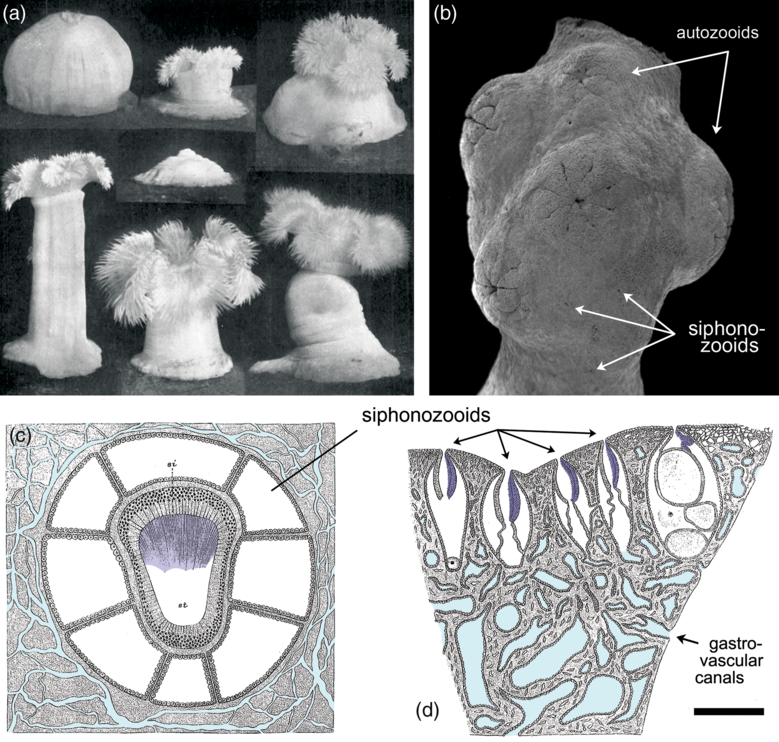
Fig. 3. Extant anthozoan cnidarians exhibiting features of relevance to the interpretation of Ediacaran rangeomorphs. (a) The modern actiniarian Metridium, demonstrating the disparate range of forms possible by a single specimen depending on the retention and deployment of seawater within the gastrovascular cavity. In the absence of muscle, such an organism would be unable to operate tentacles or a central mouth, although it could still (in principle) function as a suspension-feeding extra-cellular digestion chamber. (b) Scanning electron micrograph (SEM) of the colonial alcyonacean Corallium, showing the surface expression of retracted autozooids (muscle-powered micro-predatory feeding polyps) and cryptically embedded siphonozooids (cilia-powered atentaculate polyps specialized for circulating seawater); the latter are unlikely to be recognizably preserved in the fossil record, even under the most exceptional taphonomic circumstances. (c) Schematic transverse section through a single siphonozooid of the colonial alcyonacean Paragorgia, showing its ciliated water-pumping siphonoglyph (shaded dark blue) and interconnecting gastrovascular canal system (light blue). (d) Schematic longitudinal section of Paragorgia, showing multiple water-pumping siphonozooids with cryptically small external openings (siphonoglyphs shaded dark blue, gastrovascular canals light blue). Scale bar: (a) 2 cm; (b, d) 1 mm; and (c) 0.25 mm. (a) From Batham & Pantin (Reference Batham and Pantin1950), reproduced with permission of the Journal of Experimental Biology. (b) Modified from Nonaka et al. (Reference Nonaka, Nakamura, Tsukahara and Reimer2012). (c, d) Modified from Hickson (Reference Hickson1883).
Considering that such features are only expected under the most exceptional taphonomic circumstances, it is worth revisiting Grazhdankin’s (Reference Grazhdankin2004) original documentation of the 3D Winter Mountains Charnia. Intriguingly, the upwards-facing surfaces of at least two specimens bear serially repeated lensoid structures (Fig. 2a, e, arrows) that are not preserved in corresponding 2D fossils. The continuity of fossil-casting silt through these millimetre-sized structures (Fig. 2c, arrows) points to their likely function as anatomical conduits connecting the internal chambers to surrounding seawater. Taphonomic merger of the ‘upper’ and ‘lower’ surfaces during more typical death-mask preservation readily accounts for their absence in most Charnia fossils (Fig. 4c, d), as well as the misleading impression that the two sides of Charnia fronds were morphologically identical (cf. Dunn et al. Reference Dunn, Wilby, Kenchington, Grazhdankin, Donoghue and Liu2018). Indeed, the preservation of original spatial relationships in these exceptional specimens demonstrates that much of the underlying ‘fractal’ architecture was associated exclusively with the ‘lower’ surface (Fig. 2a, b). In view of their conspicuously mouldic expression, the ‘third-order branches’ of Charnia also appear not to define external morphology, but rather internalized mesentery-like structures – presumably with a primary purpose in expanding internalized surface area (Fig. 4).

Fig. 4. Schematic reconstruction of the constructional and functional anatomy of rangeomorphs, alongside possible taphonomic pathways. (a) Chambered construction with a central mesoglea-like layer (black) supporting a ciliated epithelium; external epidermis (brown) serves as an important locus of high Re/Pe gas exchange, whereas the internalized ‘gastrodermis’ (orange) is optimized for feeding at macroscopic length scales. Overall support is provided by transiently contained seawater (grey). (b) Suspended DOC and POC is cycled through the internalized system via ciliary transport and siphonoglyph-like pumping. Chamber walls are likely to have hosted a diverse, mostly anaerobic microbiome (coloured dots), contributing to gut-like extracellular digestion. (c) Three-dimensional casting of rangeomorph chambers following high-energy erosive breaching of the body wall. (d) Collapse and 2D ‘death-mask’ preservation where the body wall remains intact; the telescoping of spatially separated features onto a single surface yields specimens that appear similar on both surfaces, and obscures key aspects of the original anatomy.
Whether or not the serially repeated lensoid structures represent biological openings in the Charnia integument, it is unlikely that they provided the primary conduit for the infilling sediment. Comparable structures are not recorded in similarly preserved Rangea specimens (e.g. Jenkins, Reference Jenkins1985; Grazhdankin & Seilacher, Reference Grazhdankin and Seilacher2005; Vickers-Rich et al. Reference Vickers-Rich, Yu Ivantsov, Trusler, Narbonne, Hall, Wilson, Greentree, Fedonkin, Elliott, Hoffmann and Schneider2013; Sharp et al. Reference Sharp, Evans, Wilson and Vickers-Rich2017), and such openings in living organisms are almost universally guarded by ciliated and/or contractile cells. Full 3D preservation also requires sufficient ‘draft-through’ flow to deliver the casting sediment prior to degradational collapse (cf. Seilacher, Reference Seilacher1970; Rex, Reference Rex1985; Maeda et al. Reference Maeda, Kumagae, Matsuoka and Yamazaki2010), suggesting substantially larger-scale access to the internalized chambers. Given the high-energy tempestite conditions under which the Winter Mountains Charnia were buried (Grazhdankin, Reference Grazhdankin2004), the most likely route of silt-entraining currents would have been through abrasive breaches in the thin body wall (cf. de Bettignies et al. Reference de Bettignies, Thomsen and Wernberg2012). Indeed, the depositional continuity from fossil-casting silts to overlying cross-laminated horizons in PIN 3993-7018 (Fig. 2d) demonstrates the localized erosion of upwards-facing portions of the frond; it is only where the casts are locally succeeded by low-energy muddy laminae that the differentiated anatomy of this surface is preserved (Figs 2a, e, 4c). In a similar vein, Brasier et al. (Reference Brasier, Liu, Menon, Matthews, McIlroy and Wacey2013) have interpreted features of the high-relief rangeomorphs at Spaniard’s Bay as the consequence of body-wall rupture and/or removal during hydraulic scouring events, and it is notable that the majority of three-dimensionally cast rangeomorphs occur in conspicuously more abrasion- and transport-prone ‘Nama-type’ facies (Grazhdankin, Reference Grazhdankin2004; Grazhdankin & Seilacher, Reference Grazhdankin and Seilacher2005; Vickers-Rich et al. Reference Vickers-Rich, Yu Ivantsov, Trusler, Narbonne, Hall, Wilson, Greentree, Fedonkin, Elliott, Hoffmann and Schneider2013; Sharp et al. Reference Sharp, Evans, Wilson and Vickers-Rich2017).
Rapid sedimentary casting of bag-like compartments is also documented in a range of co-occurring non-rangeomorph Ediacaran taxa. Among the most spectacular examples are in situ, vertically oriented populations of Charniodiscus in Zimnie Gory sections of the White Sea (Grazhdankin, Reference Grazhdankin2014; Ivantsov, Reference Ivantsov2016), reclined but similarly frondose Pambikalbae and Arborea from Nilpena in South Australia (Jenkins & Nedin, 2007; Laflamme et al. Reference Laflamme, Gehling and Droser2018; Dunn et al. Reference Dunn, Liu and Gehling2019; Droser et al. Reference Droser, Tarhan, Evans, Surprenant and Gehling2020) and globular tripartite Ventogyrus from the Onega River area of the White Sea (Ivantsov & Grazhdankin, Reference Ivantsov and Grazhdankin1997; Fedonkin & Ivantsov, Reference Fedonkin and Ivantsov2007). All of these fossils have been variably infilled during event-bed sedimentation, yielding a taphonomic continuum from fully inflated 3D casts through to essentially 2D death-mask imprints. As with rangeomorphs, the form of the casts directly mirrors that of the external moulds, attesting to the thin deformable nature of the chamber walls (Jenkins & Nedin, 2007; Sharp et al. Reference Sharp, Evans, Wilson and Vickers-Rich2017) and the presence of a rapidly displaceable, chamber-filling fluid. Also like rangeomorphs, there is little direct evidence of the openings through which seawater may have entered in life but, similarly, no expectation that these should be recognizably preserved. Again, the infilling sediment was most likely introduced via abrasional breaches in thin body walls. Regardless of any phylogenetic connection to rangeomorphs, the localized 3D casting of these arboreomorphs and other problematica point to a similar grade of chambered construction and hydrostatic support.
2.b. Functional morphology of hydrostatic exoskeletons
The recognition of rangeomorph chambers with direct conduits to the external environment means that the contained seawater was topologically on the outside of the organism, that is, within a bag-like hydrostatic (exo)skeleton. There is nothing particularly exotic about such a system; indeed, it is the primary means of structural support among basal eumetazoans. In actiniarian (sea anemones) and pennatulacean (sea pens, etc.) anthozoans, for example, it is transiently retained seawater in the gastrovascular system that provides the antagonist against which epithelial muscle acts to generate overall form and movement (Fig. 3a) (Batham & Pantin, Reference Batham and Pantin1950; Chapman, Reference Chapman1958). At a more fundamental level, even the muscle can be dispensed with since the flow and containment of seawater within the system is based on embedded ciliary pumps, typically expressed in the form of channelized siphonoglyphs (Fig. 3c, d). This self-supporting bag-like construction offers an exceptionally parsimonious means of assembling a macroscopic organism, not based on costly biominerals, differentiated tissue systems or coelomic fluids, but on environmental water that comes for free. The only substantial costs are a mesoglea-like internal layer that defines the overall form of the inflated chamber (cf. Batham & Pantin, Reference Batham and Pantin1951; Tucker et al. Reference Tucker, Shibata and Blankenship2011) and an enveloping epithelial layer to ensure its integrity (Fig. 4a) (cf. Tyler, Reference Tyler2003; Jonusaite et al. Reference Jonusaite, Donini and Kelly2016). With a charging mechanism based on the plesiomorphic capacity of eukaryotes to pump water (Butterfield, Reference Butterfield2018), such an apparatus provides access to most of the fluid dynamic advantages of large size without the metabolic trade-offs accompanying more complex, carbon-rich body plans (Thingstad et al. Reference Thingstad, Øvreås, Egge, Løvdal and Heldal2005; Acuña et al. Reference Acuña, López-Urrutia and Colin2011; Pitt et al. Reference Pitt, Duarte, Lucas, Sutherland, Condon, Mianzan, Purcell, Robinson and Uye2013).
Most significantly, the middle Ediacaran invention of this bag-like habit solved the problem of conducting extracellular digestion at macroscopic length scales. By containing the process within an essentially impermeable integument, hydrolytic exo-enzymes could now be freely released without advective loss to the environment or competing organisms, even under the turbulent conditions associated with centimetre- to metre-length scales (cf. Sher et al. Reference Sher, Fishman, Melamed-Book, Zhang and Zlotkin2008; Agostini et al. Reference Agostini, Suzuki, Higuchi, Casareto, Yoshinaga, Nakano and Fujimura2012; Raz-Bahat et al. Reference Raz-Bahat, Douek, Moiseeva, Peters and Rinkevich2017; Goldberg, Reference Goldberg, Kloc and Kubiak2018; Steinmetz, Reference Steinmetz2019). Combined with organismal control over the cycling of seawater, the presence of a large-scale holding and mixing vessel provided both the time and hydrodynamic conditions necessary for optimal uptake and digestion, particularly in the presence of substantially expanded mesentery-like surface area (Fig. 4a, b). In modern industrial applications such structures are known as chemical reactors; in biology, they are regularly employed as guts (Penry & Jumars, Reference Penry and Jumars1987).
The most basic type of gut among living animals is the single-opening ‘batch reactor’ of predatory cnidarians, where individual prey items are processed within a (transiently closed) gastrovascular cavity, followed by the regurgitation of undigested remains (Schick, Reference Schick1991; Sher et al. Reference Sher, Fishman, Melamed-Book, Zhang and Zlotkin2008; Schlesinger et al. Reference Schlesinger, Zlotkin, Kramarsky-Winter and Loya2009; Raz-Bahat et al. Reference Raz-Bahat, Douek, Moiseeva, Peters and Rinkevich2017; Steinmetz, Reference Steinmetz2019). Such behaviour, however, is predicated on the availability of suitable prey and a muscle-based means of capturing and manipulating it, for which there is no direct evidence in middle Ediacaran deposits. In this context, the more appropriate model for extracellular digestion is a continuous-flow stirred-tank reactor (CSTR), involving the continuous processing of dissolved or suspended substrate as it passes through a reaction chamber (Penry & Jumars, Reference Penry and Jumars1986). This type of unidirectional water cycling is widely employed by extant cnidarians, where cilia- and siphonoglyph-based pumping is capable of marshalling complex flow paths, even within blind-ended chambers and canals (Southward, Reference Southward1955; Holley & Shelton, Reference Holley and Shelton1984; Schick, Reference Schick1991; Parrin et al. Reference Parrin, Netherton, Bross, McFadden and Blackstone2010; Harmata et al. Reference Harmata, Parrin, Morrison, McConnell, Bross and Blackstone2013; Goldberg, Reference Goldberg, Kloc and Kubiak2018). Fully open-ended unidirectional processing has also been achieved secondarily in colonial pennatulacean and alcyonacean octocorals, through the differentiation and interconnection of water-pumping siphonozooids and stolon systems (Fig. 3c, d) (Hickson, Reference Hickson1883; Brafield, Reference Brafield1969; Williams et al. Reference Williams, Hoeksema and Van Ofwegen2012; Nonaka et al. Reference Nonaka, Nakamura, Tsukahara and Reimer2012), as well as in the atentaculate solitary coral Leptoseris fragilis via the formation of micrometre-scale gastrovascular pores (Schlicter, Reference Schlicter1991). Significantly, active gastrovascular cycling of seawater proceeds even where its skeletal function has been largely superseded by hard skeleton, demonstrating a primary purpose in feeding and internal transport. The ‘fractally’ partitioned hydrostatic exoskeleton of rangeomorphs was similarly suited to such CSTR-like processing.
The Ediacaran introduction of large, gently stirred, semi-enclosed, reaction vessels would have been equally revolutionary from a microbial point of view. Along with the massively expanded area for surface attachment, microbial residence within the rangeomorph chamber system offered both a continuously buffered habitat and essentially unlimited levels of host-delivered resources (Fig. 4b). At the same time, localized containment allowed the direct physiological coupling of otherwise incompatible modes of life. In the bilaterian gut, for example, it is clear that the anaerobic conditions necessary for optimal digestion are maintained both by and for the resident microbiome (Plante, Reference Plante1990; Friedman et al. Reference Friedman, Bittinger, Esipova, Hou, Chau, Jiang, Mesaros, Lund, Liang, FitzGerald, Goulian, Lee, Garcia, Blair, Vinogradov and Wu2018; Litvak et al. Reference Litvak, Byndloss and Bäumler2018), even as the collective ‘holobiome’ takes advantage of a fully oxygenated existence. Comparably steep redox gradients are found in the gastrovascular cavities of extant cnidarians (Agostini et al. Reference Agostini, Suzuki, Higuchi, Casareto, Yoshinaga, Nakano and Fujimura2012), offering similar opportunities for such catabolic partnerships (Viver et al. Reference Viver, Orellana, Hatt, Urdiain, Díaz, Richter, Antón, Avian, Amann, Konstantinidis and Rosselló-Móra2017; Goldberg, Reference Goldberg, Kloc and Kubiak2018). In the case of actiniarian and pennatulacean anthozoans, rhythmic cycling between hypoxic and anoxic conditions within the gastrovascular system (Brafield & Chapman, Reference Brafield and Chapman1967; Chapman, Reference Chapman1972; Jones et al. Reference Jones, Pickthall and Nesbitt1977; Brafield, Reference Brafield1980) reflects the active suppression of oxygen levels, even in the presence of regularly transiting oxygenated seawater (Penry & Jumars, Reference Penry and Jumars1987; Smith & Waltman, Reference Smith and Waltman1995; Agostini et al. Reference Agostini, Suzuki, Higuchi, Casareto, Yoshinaga, Nakano and Fujimura2012). Given the ubiquity of metabolically diverse microbes in the marine realm, the Ediacaran appearance of bag-like rangeomorphs can be viewed as the original evolutionary experiment linking high-Re oxygen-respiring multicellular eukaryotes to a low-Re, hypoxic to anoxic, microbial digester. Such symbioses will have dramatically expanded the capacity of Ediacaran eukaryotes to feed on dilute and/or recalcitrant DOC, while also tapping into the rich physiological, immunological and developmental potential of such redox-sensitive relationships (e.g. McFall-Ngai et al. Reference McFall-Ngai, Hadfield, Bosch, Carey, Domazet-Lošo, Douglas, Dubilier, Eberl, Fukami, Gilbert, Hentschel, King, Kjelleberg, Knoll, Kremer, Mazmanian, Metcalf, Nealson, Pierce, Rawls, Reid, Ruby, Rumpho, Sanders, Tautz and Wernegreen2013; Dishaw et al. Reference Dishaw, Cannon, Litman and Parker2014; Hammarlund, Reference Hammarlund2020).
In addition to optimizing digestion, a large-scale chemical reactor requires reliable delivery of reactants. Although rangeomorphs preserve little direct evidence of their water-processing habits, simply the turbulence generated by the elevated canopy and background currents will have ensured a continuous supply of food and gas exchange (Larsen & RiisgÅrd, Reference Larsen and RiisgÅrd1997; Lassen et al. Reference Lassen, Kortegrd, RiisgÅrd, Friedrichs, Graf and Larsen2006; Singer et al. Reference Singer, Plotnik and Laflamme2012; Ghisalberti et al. Reference Ghisalberti, Gold, Laflamme, Clapham, Narbonne, Summons, Johnston and Jacobs2014). By capitalizing on both the active hydrodynamics of their exposed mostly turbulent outsides, and the unique chemical and microbial milieu of their semi-contained ‘insides’, chamber-forming rangeomorphs invented a fundamentally new way of feeding, breathing and making a living.
2.c. Rangeomorph affiliations
A cnidarian grade of construction does not mean that rangeomorphs were necessarily cnidarians, but it usefully rules out a number of the usual suspects. There is, for example, no feasible means by which the cytoplasmic contents of coenocytic or syncytial eukaryotes could be replaced with sediment on timescales compatible with the 3D preservation of these soft-bodied organisms. Moreover, the absence of any known seaweeds using this sort of chambered, taphonomically castable construction makes an algal interpretation unlikely, even in photic-zone settings. Any convincing case for metazoan affiliation, however, requires the positive identification of diagnostically metazoan features, set in a phylogenetic context. Ignoring problematic ctenophores, there are currently three principal hypotheses for where rangeomorphs might reasonably be positioned within total-group Metazoa: (1) the sister-group of all extant animals (stem-group Metazoa) (Xiao & Laflamme, Reference Xiao and Laflamme2009; Budd & Jensen, Reference Budd and Jensen2017; Dunn et al. Reference Dunn, Liu and Donoghue2017; Darroch et al. Reference Darroch, Smith, Laflamme and Erwin2018); (2) the sister-group of all extant animals minus sponges (stem-group Eumetazoa) (Buss & Seilacher, Reference Buss and Seilacher1994; Dunn et al. Reference Dunn, Liu and Donoghue2017; Hoyal Cuthill & Han, Reference Hoyal Cuthill and Han2018); or (3) the sister-group of all extant cnidarians (stem-group Cnidaria) (Dunn et al. Reference Dunn, Liu and Donoghue2017).
Despite the potential for confusing non-preservation with a true absence of derived characters (Sansom et al. Reference Sansom, Gabbott and Purnell2010), it is clear that rangeomorphs lacked a number of key crown-cnidarian attributes, not least an ability to move or respond usefully to sedimentary inundation. Under comparable levels of event-bed sedimentation, modern actiniarian and pennatulacean cnidarians engage in pronounced whole-organism or whole-colony contraction, an escape response that both fluidizes surrounding sediments and precludes any infilling of gastrovascular compartments (Batham & Pantin, Reference Batham and Pantin1950; Kastendiek, Reference Kastendiek1976; Norris, Reference Norris1989; Holst & Jarms, Reference Holst and Jarms2006; Chimienti et al. Reference Chimienti, Angeletti and Mastrototaro2018). The conspicuously unresponsive habit of rangeomorphs reliably demonstrates their lack of cnidarian-grade muscle. It is also consistent with a lack of (muscle-activated) tentacles and a localized mouth, which in turn implies absence of a cnidarian-grade nerve net, predatory cnidae or predation-based feeding.
In the absence of muscle and associated systems, there appears to be little more to rangeomorphs than perforated bags of water charged by ciliary pumps. But even this represents a fundamental departure from protistan or sponge-grade multicellularity (Arendt et al. Reference Arendt, Benito-Gutierrez, Brunet and Marlow2015). At macroscopic length scales, such a membranous structure can only be realistically achieved with the mechanical reinforcement afforded by specialized intercellular adhesion molecules and a collectivized, extracellular, basement membrane (Tyler, Reference Tyler2003; Nielsen, Reference Nielsen2008; Jonusaite et al. Reference Jonusaite, Donini and Kelly2016). This type of differentiated epithelium is a uniquely eumetazoan feature, and its (inferred) identification in thin-walled rangeomorphs convincingly places these problematic fossils within total-group Eumetazoa (Budd & Jensen, Reference Budd and Jensen2017). The degree to which they can be more precisely resolved depends on the identification of additional phylogenetically informative characters. As pre-muscular, epithelial, tank-based digesters, they offer a compelling model for stem-group eumetazoans. To the extent that macroscopically responsive striated muscle appears to have evolved independently in cnidarians and bilaterians (cf. Steinmetz et al. Reference Steinmetz, Kraus, Larroux, Hammel, Amon-Hassenzahl, Houliston, Wörheide, Nickel, Degnan and Technau2012), they might further be viewed as pre-muscular, pre-predatory, stem-group cnidarians (cf. Marcum & Campbell, Reference Marcum and Campbell1978; Dunn et al. Reference Dunn, Liu and Donoghue2017).
There is much discussion over the nature of the ancestral (eu)metazoan, but the development of a gastrula phase – where the outside surface of a spherical blastula becomes sufficiently invaginated to act as an ‘inside’ – was undoubtedly a key innovation (Nielsen, Reference Nielsen2008; Arendt et al. Reference Arendt, Benito-Gutierrez, Brunet and Marlow2015). Although topologically equivalent to a solitary bag-like cnidarian, neither the gastrula nor its hypothetical ‘gastraea’ counterpart in early metazoan evolution is obviously comparable to macroscopic rangeomorphs, presumably because a large centralized mouth has no function in the absence of muscle, tentacles or, indeed, any food particles large enough to require such an apparatus. In this context, there is a compelling argument for viewing these macroscopic fossils not as single organisms, but as integrated suspension-feeding colonies, broadly analogous to those of extant pennatulacean and alcyonacean octocorals. The serially repeated pore-like structures in three-dimensionally preserved Charnia (Fig. 2) certainly point to colony-like modularity (cf. Dewel, Reference Dewel2000; Dewel et al. Reference Dewel, Dewel and McKinney2001; Hoyal Cuthill & Conway Morris, Reference Hoyal Cuthill and Conway Morris2014; Dececchi et al. Reference Dececchi, Narbonne, Greentree and Laflamme2017; Kenchington et al. Reference Kenchington, Dunn and Wilby2018), while the quantum increase in length scales associated with coloniality would have provided fundamentally enhanced access to water-borne resources without the costs of developing a more sophisticated body plan (cf. Acuña et al. Reference Acuña, López-Urrutia and Colin2011; Pitt et al. Reference Pitt, Duarte, Lucas, Sutherland, Condon, Mianzan, Purcell, Robinson and Uye2013). Unlike predatory octocorals, however, all of the constituent individuals or modules of this hypothetical pre-muscular, colonial rangeomorph would have been deployed as cilia-powered, broadly gastraea-like ‘siphonozooids’, with a primary purpose in circulating water (Figs 3c, d, 4). This does not mean that they are homologous with the siphonozooids of crown-group cnidarians of course (cf. Landing et al. Reference Landing, Antcliffe, Geyer, Kouchinsky, Bowser and Andreas2018). A colonial or modular suspension feeding habit is likely to have evolved independently in any number of stem-group metazoan lineages, just as it has among extant groups (Ryland & Warner, Reference Ryland and Warner1986).
3. Conclusion
Rangeomorphs remain one of the most deeply problematic groups in the fossil record, even as ongoing work reveals novel developmental, anatomical and ecological detail (e.g. Sharp et al. Reference Sharp, Evans, Wilson and Vickers-Rich2017; Kenchington & Wilby, 2017; Dunn et al. Reference Dunn, Wilby, Kenchington, Grazhdankin, Donoghue and Liu2018; Kenchington et al. Reference Kenchington, Dunn and Wilby2018; Liu & Dunn, Reference Liu and Dunn2020). The present study yields yet further levels of biological resolution:
-
1. Rangeomorphs were not osmotrophic. The hydrodynamics associated with organisms of this size are physically and biochemically incompatible with such a habit.
-
2. Rangeomorphs were supported by a hydrostatic exoskeleton composed of seawater, as demonstrated by the ready castability of internalized chambers during event-bed sedimentation.
-
3. The rangeomorph integument was thin-walled, comprising a biomechanically reinforced epithelium and associated mesoglea-like layer. This plastic, bag-like structure was breachable under high-energy siliciclastic sedimentation, but had sufficient integrity to allow three-dimensional casting in silt and sand.
-
4. Serially repeated lensoid structures developed (unilaterally) on at least some rangeomorph taxa potentially represent the openings through which seawater circulated in life. Smaller and/or non-preserved channels may also have fulfilled this role, analogous to the cryptic siphonozooids of some modern octocorals.
-
5. The flow of seawater through the rangeomorph chamber system is likely to have been driven by collective ciliary pumping, a plesiomorphic property of both metazoans and eukaryotes.
-
6. Rangeomorph chambers provided the controlled hydrodynamic and physiological circumstances necessary to conduct extracellular digestion and phagocytosis at macroscopic length scales.
-
7. The constructional and functional anatomy of rangeomorphs identifies them as pre-muscular, total-group Eumetazoa.
Prior to the appearance of rangeomorphs there were just two feeding strategies available to free-living heterotrophic organisms: external digestion plus osmotrophy as practiced by prokaryotes and fungi, and the more active capture and internal digestion of phagocytizing protozoans (and sponges). Chamber-forming eumetazoans broke into this ancient duopoly, not by beating microbes at their own game, but through the invention of a revolutionary new technique for harvesting and processing food. By exploiting the unique potential of large size and compartmentalization, eumetazoans tapped into both the turbulent hydrodynamics of their ‘outside’ (discovering an effectively inexhaustible source of both food and gas exchange) and the controlled conditions of their ‘inside’ (allowing both extracellular digestion and ‘osmotrophic’ uptake to be conducted on an industrialized, CSTR-like scale). The key to all of this biological potential was a hydrostatic exoskeleton based on a bag-like epithelium charged by ciliary pumps. In one form or another, such construction underpins the physiology of all eumetazoan life.
Acknowledgements
I thank Graham Budd, Dima Grazhdankin and Alex Liu for valuable discussion, and journal reviewers for their constructive feedback. Fossil images were generously contributed by Dima Grazhdankin, Alex Liu, Jean-Bernard Caron, Phil Wilby and Olga Zhaxybayeva. Figure 3a is reproduced with permission of the Journal of Experimental Biology. This research was supported by Natural Environment Research Council (NERC) grant NE/P002412/1.