1. Introduction
In the upper crust, deformation usually localizes on major faults because of the frictional mechanical behaviour and strain weakening active in the crust (e.g. Collettini et al. Reference Collettini, Niemeijer, Viti and Marone2009). A notable exception is the sedimentary cover that has the ability to fold mainly because of the mechanical stratigraphy with weak, low-stress interbeds. Thus, in contractional setting, sedimentary cover usually deforms with typical fold-and-thrust belt kinematics. In the basement, however, mainly composed of crystalline rocks, the deformation is more localized than in the sedimentary cover. Only approaching the brittle–ductile transition, and below, is the deformation distributed, mainly due to temperature increase.
Thus, for basement rocks in the upper crust up to the surface, our understanding of crustal rheology will predict localized, major faults. In some cases, however, the deformation is seen as more distributed in the field. This distributed, though brittle, deformation has been observed at a few places, mainly in the Laramide Orogenic belt (Rocky Mountains), as well as in South America: it is interpreted either as basement deformation over a fault-bend fold (Casas et al. Reference Casas, Oliva, Roman-Berdiel and Pueyo2003) or in a fault-propagation fold with triangular zones of shear ahead of or near the thrust upper tip (Erslev, Reference Erslev1991; Schmidt, Genovese & Chase, Reference Schmidt, Genovese, Chase, Schmidt, Chase and Erslev1993; Bump, Reference Bump2003; Garcia & Davis, Reference Garcia and Davis2004; Beaudoin et al. Reference Beaudoin, Leprêtre, Bellahsen, Lacombe, Amrouch, Callot, Emmanuel and Daniel2012).
The Sierras Pampeanas (Fig. 1), a modern analogue of the Laramide Rocky Mountains (Jordan & Allmendinger, Reference Jordan and Allmendinger1986), provide a unique setting for studying basement deformation in a shallow brittle setting. The Sierra Pie de Palo (Figs 1, 2, 3) is a basement uplift in the Sierras Pampeanas, NW Argentina. It is topped by a 3000 m high perched erosional surface that attests for a significant structural relief. This structure is interpreted either as a fault-bend fold on a W-verging thrust (Ramos, Cristallini & Pérez, Reference Ramos, Cristallini and Pérez2002; Vergés et al. Reference Vergés, Ramos, Meigs, Cristallini, Bettini and Cortés2007) or as a fold above an E-verging blind thrust that branches at depth on a W-verging thrust (Siame et al. Reference Siame, Bellier, Sebrier, Bourlès, Leturmy, Perez and Araujo2002, Reference Siame, Sebrier, Bellier, Bourlès, Costa, Ahumada, Gardini and Cisneros2015; Siame, Bellier & Sebrier, Reference Siame, Bellier and Sebrier2006). In both cases, these models imply some distributed deformation in the basement that necessitates a detailed study.

Figure 1. (a) Map of Central Andes (Landsat images and General Bathymetric Charts of the Oceans) together with distribution of active volcanoes (orange solid triangles, Smithsonian Institution, Global Volcanism Program) and seismicity (red solid dots, US Geological Survey seismicity catalogue, M ≥ 4.0 and depth ≤ 70 km). White solid lines (labelled in km) are isodepths of the Wadati–Benioff plane and highlight the Pampean flat slab of the Nazca plate (after Ammirati et al. Reference Ammirati, Alvarado, Perarnau, Saez and Monsalvo2013). Key: AP, Argentine Precordillera; SP, Sierras Pampeanas; JRF, Juan Fernandez Ridge. (b) Schematic regional structural map of the Sierras Pampeanas. Red solid lines denote active structures. Key: AP, Argentine Precordillera; WP, Western Argentine Precordillera; CP, Central Argentine Precordillera; EP, Eastern Central Precordillera; SPDP, Sierra Pie de Palo; SH, Sierra de la Huerta; SVF, Sierra de Valle Fértil; SMa, Sierra La Maz; SFa, Sierra de Famatina; SFi, Sierra Fiambalaà; SA, Sierra Ambato; SV, Sierra Velasco; SCh, Sierra Chepes; SC, Sierra de Còrdoba; SL, Sierra San Luis; SU, Sierra Umango; SPa, Sierra de las Planchadas.

Figure 2. Tentative schematic structural map of the Sierra Pie de Palo and surroundings showing the major faults. Modified after Siame et al. (Reference Siame, Sebrier, Bellier, Bourlès, Costa, Ahumada, Gardini and Cisneros2015). CF, Las Chacras Fault; EPF, East Pie de Palo Fault; LPF, Los Pajaritos Fault; NPF, North Pie de Palo Fault; SHF, Sierra de la Huerta Fault; TVF, Tulum Valley Fault; VFF, Valle Fértil Fault; VPF, Villicùm–Pedernal Fault. The dashed line east of the Pie de Palo is the limit between alluvial fans and the flat playa type of Quaternary sediments. Its linear geometry suggests that the alluvial fans are slightly tilted; this should be controlled by the blind E-verging thrust. Indeed, the older fan surfaces are steeper than the younger ones (the older the fan, the steeper its surface). Light blue stars locate 1944 (M w = 7.0) and 1952 (M w = 6.8) earthquakes with focal mechanism solutions after Alvarado and Beck (Reference Alvarado and Beck2006). Black stars locate fore- and main shocks of the Caucete 1977 (M s = 7.4) earthquake (focal mechanism solutions after Langer & Hartzell, Reference Langer and Hartzell1996).

Figure 3. (a) Landsat image of the Sierra Pie de Palo. Both ≈E–W (B–B′) and ≈N–S (C–C′) topographic cross-sections have been extracted from the Shuttle Radar Topography Mission 1990 digital elevation model (SRTM90 DEM) (thick solid lines). The reconstructed regional erosion surface (thick dotted lines) is after Siame et al. (Reference Siame, Sebrier, Bellier, Bourlès, Costa, Ahumada, Gardini and Cisneros2015). The two stars locate the two 1977 earthquakes with their respective focal solutions (F for fore shock and M for main shock) (Langer & Hartzell, Reference Langer and Hartzell1996). Key: GEM, Grande El Molle lineament; GL, Guayaupa–Lima lineament. (b) Geological map with main foliation orientations modified from Ramos and Vujovich (Reference Ramos and Vujovich2000), Mulcahy et al. (Reference Mulcahy, Roeske, McClelland, Jourdan, Iriondo, Renne, Vervoort and Vujovich2011) and Van Staal et al. (Reference Van Staal, Vujovich and Currie2011).
Thanks to excellent outcrop conditions in the Pie de Palo area, we combined structural and microstructural observations along its eastern margin and available data to propose a model for basement folding integrating outcrop- and crustal-scale interpretations. In particular, we propose that, in the uppermost crust, the shortening, localized on inherited foliation planes that folded and evolved as cataclastic faults, may have allowed folding of the upper crust above an E-verging blind thrust. We propose new cross-sections at crustal and lithospheric scale that are consistent with the geological and geophysical data and constitute an alternative model for Andean shortening in the specific Andean backarc province (Cuyania crust).
2. Regional setting of the Sierras Pampeanas
The Pie de Palo anticline is one of the Sierras Pampeanas ranges that extend between 27° and 33°S and 64° and 68°W (Fig. 1b). These ~N-striking ranges (NNW to NNE) correspond to Neogene–Quaternary basement-block uplifts, which are located to the east of the Andes (Fig. 1). These basement blocks extend where the Nazca slab, which is subducting eastward below the western edge of the South American plate, flattens below the Andes at a depth of ~100 km (Jordan et al. Reference Jordan, Isacks, Ramos and Allmendinger1983; Jordan & Allmendinger, Reference Jordan and Allmendinger1986; Cahill & Isacks, Reference Cahill and Isacks1992; Ramos, Cristallini & Pérez, Reference Ramos, Cristallini and Pérez2002; Anderson et al. Reference Anderson, Alvarado, Zandt and Beck2007). This segment of the Andean subduction is characterized by a ~N75°E-trending convergence with a rate of c. 75 mm a−1 (DeMets et al. Reference DeMets, Gordon, Argus and Stein1990). The flat slab geometry appears to result from the subduction of the Juan Fernandez aseismic ridge (Fig. 1a), which increases the buoyancy of the Nazca plate (Pilger, Reference Pilger1981; Gutscher et al. Reference Gutscher, Maury, Eissen and Bourdon2000). This ridge subduction initiated between 14 and 11 Ma, moving from north to south along the Chilean trench (Yanez et al. Reference Yanez, Ranero, von Huene and Diaz2001). The volcanic arc migrated eastward from ~11 Ma to ~8 Ma to shut off totally by 5 Ma (Kay & Mpodozis, Reference Kay and Mpodozis2002), and the flat geometry reached the retroarc region between Late Miocene and Pliocene (Kay & Abbruzzi, Reference Kay and Abbruzzi1996). Indeed, Andean regions with flat slab subduction (e.g. Central Peru and Sierras Pampeanas) are commonly characterized by an extinct volcanic arc and display a much wider shortening retroarc belt because it should accommodate larger amounts of shortening than the neighbouring regions characterized by steeper slabs (Jordan et al. Reference Jordan, Isacks, Ramos and Allmendinger1983; Sebrier et al. Reference Sebrier, Mercier, Macharé, Bonnot, Cabrera and Blanc1988). This shortening deformation is also possibly enhanced by thermal weakness of the crust associated with the eastward migration of the asthenospheric wedge (Ramos, Cristallini & Pérez, Reference Ramos, Cristallini and Pérez2002).
The 27–33°S retroarc shortening province associated with the Andean flat slab segment comprises two main parts (Fig. 1b): (1) a ~40 km narrow, E-verging, thin-skinned, fold-and-thrust belt composed of Palaeozoic–Mesozoic rocks and extending to the east of the main high Andes from Sierra de Las Planchadas (27.5°S) up to the southern Precordillera in the Mendoza area (33°S) and (2) a ~350 km wide subprovince of mostly W-verging block uplifts made of Precambrian and early Palaeozoic metamorphic rocks, i.e. the Sierras Pampeanas. Overall, the E-verging thin-skinned thrusts initiated earlier (Early to Mid Miocene) than the Pampean basement uplifts, which started between the Late Miocene in the northern Sierras Pampeanas and the Pliocene–Quaternary in the southern ones (Jordan et al. Reference Jordan, Allmendinger, Damanti and Drake1993; Zapata & Allmendinger, Reference Zapata and Allmendinger1996). Indeed, these basement uplifts are located within the distal part of the Miocene foreland basin as out-of-sequence faulted blocks so that this foreland is generally described as a broken foreland (Jordan et al. Reference Jordan, Isacks, Ramos and Allmendinger1983). Typically, the sedimentary signature of these uplifts is the onset of conglomerate deposits atop the previous thin distal sedimentation (Jordan et al. Reference Jordan, Allmendinger, Damanti and Drake1993). These blocks uplifts are controlled by the reactivation of inherited crustal weaknesses, such as Proterozoic or Early Palaeozoic sutures and Late Palaeozoic or Mesozoic graben normal faults (Ramos, Cristallini & Pérez, Reference Ramos, Cristallini and Pérez2002), which explain their variable lengths from several tens to several hundreds of kilometres. Interestingly, the Pampean ranges have a wide range of altitudes between ~1000 and 6000 m, with a mean tendency to higher elevations northward and westward, which may result both from an earlier northern initiation and a larger amount of shortening closer to the Andes.
The area of interest is located on the ~400 km wide south-central profile of the Sierras Pampeanas (~31.5°S), which extends approximately between the cities of San Juan and Còrdoba (Fig. 1b). Adding the ~50 km wide, thin-skinned fold-and-thrust belt yields a width of ~450 km for the Neogene-to-present retroarc shortening province. This central profile offers the following characteristics: (1) it is located just above the central part of the flat slab segment (Anderson et al. Reference Anderson, Alvarado, Zandt and Beck2007), (2) it has been the focus of many geological studies (Ramos, Cristallini & Pérez, Reference Ramos, Cristallini and Pérez2002 and references therein), particularly in its western stretch where most of the shortening is accommodated, (3) the Neogene to present history of shortening is relatively well constrained to 90 km, yielding a regional mean rate of 4.5 mm a−1 during the last 20 Ma (Allmendinger et al. Reference Allmendinger, Figueroa, Snyder, Beer, Mpodozis and Isacks1990; Jordan, Schlunegger & Cardozo, Reference Jordan, Schlunegger and Cardozo2001), and (4) the level of crustal seismicity is the highest along this profile in the whole retroarc belt (Smalley et al. Reference Smalley, Pujol, Regnier, Chiu, Chatelain, Isacks, Araujo and Puebla1993; Siame et al. Reference Siame, Bellier, Sebrier and Araujo2005). Consequently, many seismological studies provide information on the crustal seismicity (Kadinsky-Cade & Reilinger, Reference Kadinsky-Cade and Reilinger1985; Regnier et al. Reference Regnier, Chatelain, Smalley, Chiu, Isacks and Araujo1992; Smalley et al. Reference Smalley, Pujol, Regnier, Chiu, Chatelain, Isacks, Araujo and Puebla1993; Alvarado et al. Reference Alvarado, Beck, Zandt, Araujo and Triep2005, Reference Alvarado, Pardo, Gilbert, Miranda, Anderson, Saez, Beck, Mahlburg Kay, Ramos and Dickinson2009; Alvarado & Beck, Reference Alvarado and Beck2006; Alvarado, Beck & Zandt, Reference Alvarado, Beck and Zandt2007) and the lithospheric and crustal structures (Ammirati, Alvarado & Beck, Reference Ammirati, Alvarado and Beck2015 and references therein). The main results obtained along this profile are summarized hereafter. The eastward cessation of volcanism in the Central Precordillera (68.8°W) by 7 Ma and in the Sierra de Còrdoba (65.2°W) by 4.7 Ma (Kay & Abbruzzi, Reference Kay and Abbruzzi1996) demonstrates that the flat slab geometry was installed below this profile between the Late Miocene and the Early Pliocene. The depth of the continental Moho varies along this profile: 66 km below the Central Precordillera (Ammirati et al. Reference Ammirati, Alvarado, Perarnau, Saez and Monsalvo2013), 52 km below the Sierra Pie de Palo (Calkins et al. Reference Calkins, Zandt, Gilbert and Beck2006; Ammirati, Alvarado & Beck, Reference Ammirati, Alvarado and Beck2015), while it is only 38 km and 35 km below the western and eastern parts of the Sierra de Cordoba, respectively (Perarnau et al. Reference Perarnau, Gilbert, Alvarado, Martino and Anderson2012). The highest level of seismicity (Smalley et al. Reference Smalley, Pujol, Regnier, Chiu, Chatelain, Isacks, Araujo and Puebla1993) is located between the Central Precordillera (68.8°W) and the Sierra del Valle Fértil – La Huerta (67.3°W); the base of this crustal seismicity appears deeper than 30 km and might correspond to a ~34 km deep seismic discontinuity, while a shallower one is also observed at a depth of ~18 km (Calkins et al. Reference Calkins, Zandt, Gilbert and Beck2006; Alvarado, Beck & Zandt, Reference Alvarado, Beck and Zandt2007; Ammirati, Alvarado & Beck, Reference Ammirati, Alvarado and Beck2015). This seismic discontinuity near the base of the crustal seismicity could correspond to the main Andean décollement that permits eastward propagation of the Andean shortening to the Sierra Pampeanas. Interestingly, the GPS velocity field measured in this high-seismicity zone suggests a step in their eastward decay that may agree with a shortening rate of ~5 mm a−1 (Brooks et al. Reference Brooks, Bevis, Smalley, Kendrick, Manceda, Lauría, Maturana and Araujo2003). Three destructive earthquakes with M w ~ 7 occurred within this region in 1944, 1952 and 1977. The first two had focal depths of 11 km and 12 km, respectively (Alvarado & Beck, Reference Alvarado and Beck2006), while the 1977 focus was estimated at ~25–30 km (Alvarado & Beck, Reference Alvarado and Beck2006 and references therein).
Finally, one important consequence of the slab geometry is the decrease in the thermal gradient in the overlying continental crust. Thermal modelling indicates a thermal lowering from ~30°C km−1 (situation above steeper Andean subduction segments) to ~10° km−1 for the flat segments caused by the lack of asthenospheric wedge (Gutscher et al. Reference Gutscher, Maury, Eissen and Bourdon2000). This low thermal gradient has been determined within the sediments of the Neogene basins (Collo et al. Reference Collo, Dávila, Teixeira, Nóbile, San't Anna and Carter2015) and explains why thermo-chronologic studies have major difficulties constraining the uplift rates of most of the Western Pampean ranges (Davila & Carter, Reference Davila and Carter2013), especially along the central profile of the Sierras Pampeanas (Löbens et al. Reference Löbens, Bense, Dunkl, Wemmer, Kley and Siegesmund2013). Obviously, this also agrees quite well with the uncommonly deep crustal seismicity observed in the region (Regnier et al. Reference Regnier, Chatelain, Smalley, Chiu, Isacks and Araujo1992; Smalley et al. Reference Smalley, Pujol, Regnier, Chiu, Chatelain, Isacks, Araujo and Puebla1993).
3. Geological setting of the Sierra Pie de Palo
The NNE-striking, oval-shaped Sierra Pie de Palo (Figs 2, 3) is located at 31.4°S, 67.9°W. It is 80 km long and 32 km wide, and has an altitude of 3162 m, towering above the surrounding 500–600 m elevated desert alluvial plain. This range is bounded by (Fig. 2): to the west, the Tulum Valley, a syncline of Neogene and Quaternary beds, which separates the Sierra Pie de Palo from the Eastern Precordillera; to the north, the 10 km thick Neogene to Quaternary Bermejo foreland basin; to the east, the triangular-shaped South Bermejo Valley, which is a Neogene to Quaternary synclinorium basin separating the Sierra Pie de Palo from the NNW-striking Sierra Valle Fértil – La Huerta range; and to the south, the southern continuation of the Bermejo basin which stands below the Medanos Grandes sand dunes. The first-order topography of the Sierra Pie de Palo (Fig. 3a) corresponds to a dissymmetric arch with steep eastern and northern slopes whereas the southern and western slopes are gently dipping (Siame, Bellier & Sebrier, Reference Siame, Bellier and Sebrier2006; Siame et al. Reference Siame, Sebrier, Bellier, Bourlès, Costa, Ahumada, Gardini and Cisneros2015). The western long limb of the range is dissected by two main WSW-striking, rectilinear, deep canyons Guayaupa-Lima and Grande El Molle (Fig. 3). However, topographic profiles along the top of the range do not reveal any significant vertical displacement associated with these transverse lineaments. Similarly, they do not displace the rectilinear eastern front of the Pie de Palo range, hence they should be presently inactive structures (Siame et al. Reference Siame, Sebrier, Bellier, Bourlès, Costa, Ahumada, Gardini and Cisneros2015).
The relatively flat areas composing the Sierra Pie de Palo range topography define a convincing surface envelope along both N–S and E–W directions (Fig. 3), shaping an anticline fold (Siame et al. Reference Siame, Sebrier, Bellier, Bourlès, Costa, Ahumada, Gardini and Cisneros2015). These flat surfaces are characteristic of most of the Sierras Pampeanas and have long been referred to as the ‘Pampean peneplain’ (Jordan et al. Reference Jordan, Zeitler, Ramos and Gleadow1989). This ‘peneplain’ appears polygenic as it formed between Middle Palaeozoic and Cretaceous times (Carignano, Cioccale & Rabassa, Reference Carignano, Cioccale and Rabassa1999; Costa et al. Reference Costa, Gardini, Diederix and Cortés2000; Rabassa, Carignano & Cioccale, Reference Rabassa, Carignano and Cioccale2010). However, the main debate concerns the timing of its exhumation. Some considered this erosion surface was mostly deformed and exhumed during the Neogene (e.g. Jordan et al. Reference Jordan, Zeitler, Ramos and Gleadow1989) while others proposed that the Sierras Pampeanas may have been exhumed since the Late Palaeozoic or Mesozoic (Carignano, Cioccale & Rabassa, Reference Carignano, Cioccale and Rabassa1999; Rabassa, Carignano & Cioccale, Reference Rabassa, Carignano and Cioccale2010; Löbens et al. Reference Löbens, Bense, Wemmer, Dunkl, Costa, Layer and Siegesmund2011, Reference Löbens, Bense, Dunkl, Wemmer, Kley and Siegesmund2013; Enkelmann et al. Reference Enkelmann, Ridgway, Carignano and Linnemann2014). In the Sierra Pie de Palo range, the age of this surface is quite imprecise as it is cutting on basement metamorphic rocks of Early Palaeozoic – Proterozoic age (Ramos & Vujovich, Reference Ramos and Vujovich2000) and covered around the range by Neogene continental deposits. These red–beige deposits correspond at the SE edge of the Pie de Palo range to the 300 m thick Niquizanga Formation and the overlying 420 m thick Río del Camperito Formation (Cuerda, Varela & Iniguez, Reference Cuerda, Varela and Iniguez1983). These two formations are correlated with the Upper Miocene – Lower Pliocene Jachal and Pliocene–Quaternary Mogna Formations, respectively; these are dated in the Bermejo basin to the north of the Pie de Palo range (Jordan et al. Reference Jordan, Allmendinger, Damanti and Drake1993; Zapata & Allmendinger, Reference Zapata and Allmendinger1996). The Niquizanga Formation, which rests directly upon the ‘Pampean peneplain’, is composed of thin clastic lacustrine beds containing evaporite facies (halite and gypsum), suggesting that the Neogene topography of the Sierra Pie de Palo range was either very subdued or even almost non-existent at that time so that the ‘Pampean peneplain’ could have been completely covered by the Neogene beds of the Bermejo foreland basin. The thickness of the Neogene beds might have totalled 2–3 km at most (e.g. Ramos & Vujovich, Reference Ramos and Vujovich2000) and more likely 1.9–2.4 km (Siame et al. Reference Siame, Sebrier, Bellier, Bourlès, Costa, Ahumada, Gardini and Cisneros2015). The first option agrees with a long history of exhumation whereas the second favours a late Cenozoic rapid exhumation. Whatever the initial thickness of Neogene deposits above the ‘Pampean peneplain’, recent cosmogenic nuclide data (10Be) favour a rapid uplift of the Pie de Palo anticline during the last 2–4 Ma (Siame et al. Reference Siame, Sebrier, Bellier, Bourlès, Costa, Ahumada, Gardini and Cisneros2015). Furthermore, the low thermal gradients reported in the Neogene basins (Davila & Carter, Reference Davila and Carter2013; Collo et al. Reference Collo, Dávila, Teixeira, Nóbile, San't Anna and Carter2015) also indicate that the Pliocene–Quaternary folding and uplift of the metamorphic basement must have occurred under near-surface conditions.
The Sierra Pie de Palo range is an actively growing basement anticline associated with a high level of crustal seismicity (Regnier et al. Reference Regnier, Chatelain, Smalley, Chiu, Isacks and Araujo1992; Smalley et al. Reference Smalley, Pujol, Regnier, Chiu, Chatelain, Isacks, Araujo and Puebla1993; Ramos, Cristallini & Pérez, Reference Ramos, Cristallini and Pérez2002; Siame et al. Reference Siame, Bellier, Sebrier, Bourlès, Leturmy, Perez and Araujo2002, Reference Siame, Sebrier, Bellier, Bourlès, Costa, Ahumada, Gardini and Cisneros2015; Siame, Bellier & Sebrier, Reference Siame, Bellier and Sebrier2006). This range is bounded by three major faults. The northern boundary of the range corresponds to the North Pie de Palo fault, a ~E–W-striking transpressive, left-lateral fault, which extends over 80 km from the Matagusanos basin, south of the Mogna anticline in the Eastern Precordillera (Zapata, Reference Zapata1998), to the Sierra Valle Fértil fault (Fig. 2). This regional structure also controls a major northward deepening in the basement depth across the southern boundary of the Bermejo basin (Jordan & Allmendinger, Reference Jordan and Allmendinger1986). It limits the northern edge of the Sierra Pie de Palo along c. 26 km, roughly at the contact between the metamorphic basement and the Neogene and Quaternary deposits. This stretch, which is referred as the Pajaritos Fault (Fig. 3) (Costa et al. Reference Costa, Gardini, Diederix and Cortés2000), displays fairly rectilinear N-facing scarp traces that are quite conspicuous at two locations (31.047°S, 67.94°W and 31.057°S, –67.84°W). The easternmost location of this Pajaritos Fault affects a series of alluvial fans and exhibits a well-developed 4 km long, north-facing fault scarp with a right-stepping relay agreeing with some left-lateral component of slip associated with a steep (45–60°S dipping) reverse movement. This Pajaritos Fault was interpreted as a lateral ramp of the Pie de Palo anticline, and its reverse slip rate determined to ~1 mm a−1 (Siame et al. Reference Siame, Sebrier, Bellier, Bourlès, Costa, Ahumada, Gardini and Cisneros2015).
The western boundary of the Pie de Palo metamorphic basement with the Tulum Valley syncline is very sinuous and the Quaternary bordering alluvial deposits form a very poorly incised series of fans. All these landform features suggest that no fault activity is occurring along this western edge of the range. Nevertheless, the southwesternmost contact of the basement with the Tulum Valley displays a topographic step between the ‘Pampean peneplain’ and the valley bottom that might record locally some vertical displacement along the SW Pie de Palo Anticline. Although, this sharper topography might also result from the fluvial erosion of the Precambrian basement by former meanders of the San Juan River, it is located on the possible northern termination of the Tulum fault system (Zambrano & Suvires, Reference Zambrano and Suvires2008) (Fig. 2). This fault system, which is not accurately delineated and limits the western edge of the Pampean Precambrian outcrops from the Palaeozoic outcrops of the Eastern Precordillera (Ramos, Cristallini & Pérez, Reference Ramos, Cristallini and Pérez2002), corresponds further south, at Cerro Salinas, to the emerging ramp of the active Pampean thrust system (Vergés et al. Reference Vergés, Ramos, Meigs, Cristallini, Bettini and Cortés2007) (Fig. 2).
The structure controlling the Pie de Palo range, which strikes N21°E and extends over 65 km, is the most debated as it has been described as dipping E (Langer & Bollinger, Reference Langer and Bollinger1988; Costa et al. Reference Costa, Gardini, Diederix and Cortés2000; Ramos, Cristallini & Pérez, Reference Ramos, Cristallini and Pérez2002) or W (Kadinsky-Cade & Reilinger, Reference Kadinsky-Cade and Reilinger1985; Reilinger & Kadinsky-Cade, Reference Reilinger and Kadinsky-Cade1985). It is noteworthy that, in some interpretations, the E-dipping structure beneath Pie de Palo might root at depth on a W-dipping thrust (Ramos, Cristallini & Pérez, Reference Ramos, Cristallini and Pérez2002) and, in some others, the W-dipping structure branches at depth on an E-dipping thrust (Siame et al. Reference Siame, Sebrier, Bellier, Bourlès, Costa, Ahumada, Gardini and Cisneros2015). The E-dipping hypothesis is based on the epicentre location of the 1977 M w 7.4 earthquake within the southern Bermejo Valley, on the observation of W-verging faults affecting the Neogene deposits along the eastern edge of the Sierra Pie de Palo (Ramos, Cristallini & Pérez, Reference Ramos, Cristallini and Pérez2002), and on the fact that both the Eastern Precordillera and the westernmost Sierras Pampeanas form a W-verging thrust system. This fault would thus ramp off from the Valle Fértil – La Huerta Fault at a depth of ~30 km. In contrast, the W-dipping hypothesis is mainly based on levelling data after the 1977 earthquake showing an uplift of the southern Pie de Palo anticline (Kadinsky-Cade & Reilinger, Reference Kadinsky-Cade and Reilinger1985) and crustal reconstructions from shallow and deep seismic profiling (Zapata, Reference Zapata1998) which favours a W-dipping, E-verging blind fault below the eastern border of the range. This hypothesis is also in good agreement with the observed first-order topographic signature of a steeper eastern flank, with higher Neogene dips along the eastern flank than the western one, and with a higher number of alluvial fans with steeper top surfaces than those that skirt over the western edge (Siame et al. Reference Siame, Sebrier, Bellier, Bourlès, Costa, Ahumada, Gardini and Cisneros2015). An intermediate model displays both vergences: in the upper part of the crust the Sierra Pie de Palo is controlled by an E-verging structure that branches at depth on a W-verging one (Siame et al. Reference Siame, Bellier, Sebrier, Bourlès, Leturmy, Perez and Araujo2002, 2005, Reference Siame, Sebrier, Bellier, Bourlès, Costa, Ahumada, Gardini and Cisneros2015). Moreover, even in the W-verging models, E-verging basement thrusts are also proposed below Pie de Palo (footwall thrust in Vergés et al. Reference Vergés, Ramos, Meigs, Cristallini, Bettini and Cortés2007).
Finally, a temporary seismic network, installed some ten years after the 1977 earthquake, allowed a much more accurate image of the microseismicity to be obtained (Regnier et al. Reference Regnier, Chatelain, Smalley, Chiu, Isacks and Araujo1992) that demonstrated the teleseismic locations are systematically mislocated with an eastward shift of c. 30 km. Accounting for this shift, the 1977 epicentre should be moved c. 30 km westward, locating the 1977 focus below the southern Pie de Palo range (see discussion). These data also show that the northern part of Pie de Palo stands above aligned seismic foci that define on a cross-section a W-dipping fault (Fig. 4a), which ramps off from a ~30 km depth and can be traced upward to a 7 km depth (Regnier et al. Reference Regnier, Chatelain, Smalley, Chiu, Isacks and Araujo1992). The foci depth distribution in the southern part of Pie de Palo provides a less clear image than the northern one; this vertical foci distribution might correspond to a pop-up structure (Fig. 4b; see discussion). In summary, the Pie de Palo anticline has been interpreted either as a fault-bend fold (W-verging structure), a fault propagation fold (E-verging structure), or a structure due to the interaction between both E- and W-verging structures. Focusing on puzzling W-verging reverse faults cropping out along the eastern edge and their mechanisms of deformation can help us to distinguish between these models and discuss how metamorphic basement is deformed under shallow conditions. Hereafter, we present new structural analyses, cross-sections and thin sections, allowing discussion of the structural evolution of the Sierra Pie de Palo at various scales.

Figure 4. Seismicity profiles below the Sierra Pie de Palo showing seismic foci in vertical cross-sections redrawn after Regnier et al. (Reference Regnier, Chatelain, Smalley, Chiu, Isacks and Araujo1992), (a) for the northern half of the sierra and (b) for the southern half of the sierra. See Regnier et al. (Reference Regnier, Chatelain, Smalley, Chiu, Isacks and Araujo1992) for the original data and precise location of the earthquakes that were projected on the sections. See Figure 3 for location of the cross-sections.
4. Structural analysis of the eastern Pie de Palo
One of the most peculiar features of the eastern side of the Pie de Palo range corresponds to several occurrences of range-facing, ~N–S-striking escarpments (Figs 3c, 5 and 6). These escarpments result from the Neogene to Quaternary activity of W-verging reverse faults that are parallel to the toe of the topography at several places. These faults are quite puzzling because their vergence apparently disagrees with the present topography as their relative vertical movement uplifts the South Bermejo Valley with respect to the Sierra Pie de Palo. These faults have been interpreted as the discontinuous surface trace of a major E-dipping, reverse fault, which would ramp off from a deep décollement related to the western border fault of the Valle Fértil – La Huerta range (Ramos & Vujovich, Reference Ramos and Vujovich2000; Ramos, Cristallini & Pérez, Reference Ramos, Cristallini and Pérez2002). To better understand the meaning of these faults, we mainly focus our observations in two areas: Niquizanga (Fig. 5) and Ampacama (Fig. 6).
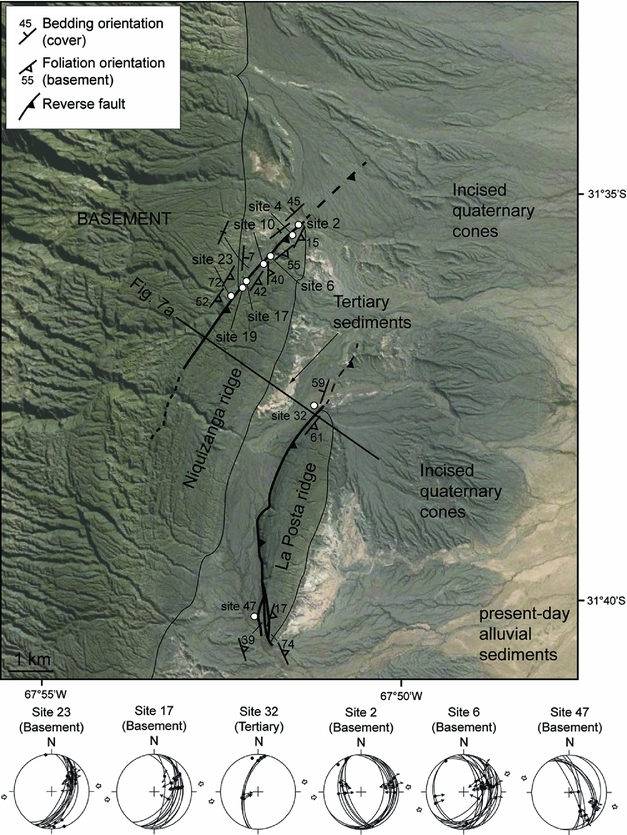
Figure 5. Structural data at Niquizanga. See Figure 3 for location. This area consists mainly of two basement ridges due to W-verging thrust faults affecting both the basement and the Cenozoic sedimentary cover. The faults are NNE–SSW and attest for an ENE–WSW shortening according to the microtectonic data. The kinematic data show axes of deformation in the basement consistent with those in the cover. Fold axes (black dots in the stereonets) are also consistent with an ENE–WSW shortening, both in the basement and the cover. In the investigated sites (white circles with numbers), the basement foliation dips eastward.

Figure 6. Structural data at Ampacama. See Figure 3 for location. This area consists of two main basement ridges due to W-verging thrust faults in the basement, thrusting above the Cenozoic sediments. The faults are NNW–SSE and attest for an E–W shortening according to the microtectonic data. The kinematic data show axes of deformation in the basement consistent with those in the cover. In the investigated sites (white circles with numbers), the basement foliation usually dips eastward, although in several places it is either vertical or west-dipping.
4.a. The Niquizanga area
In this area, two ~5 km long, basement ridges are observed (Figs 5, 7a): the western Niquizanga and the eastern La Posta ridges. These two ridges are asymmetric, with a western steep slope bordered on the west by reverse faults while their eastern slope corresponds to the Neogene unconformity. At both ridges, the basement overthrusts the Neogene sediments. These layers present a syncline geometry in the footwall, with a shallow E-dipping limb (e.g. 07°E close to site 17; Fig. 5) and a steep to vertical W-dipping one (e.g. 90° close to site 2; Fig. 5), attesting for W-verging kinematics for the fault. These faults are at least 6 km long and cannot be extended along more than 10 km. Based on their ~200 m high escarpment and the 10°E dip of the erosion surface below the Neogene sediments, the maximum fault displacement can be estimated to up to 500 m. This Niquizanga bounding fault reactivated an inherited pre-Andean shear zone (Niquizanga shear zone) that was active as an extensional structure during the Silurian (Mulcahy et al. Reference Mulcahy, Roeske, McClelland, Jourdan, Iriondo, Renne, Vervoort and Vujovich2011).

Figure 7. Cross-sections through both investigated areas. See Figures 5 and 6 for location. (a) Cross-section through Niquizanga area. Two faults are mapped and dip about 40°E, with Neogene sediments between them. The faults are parallel to the inherited foliation in the basement. (b) Cross-section through Ampacama area. Three main thrusts are represented with dips about 30°E. Along one of the faults, the fault plane is parallel to the basement foliation, while elsewhere it is strongly oblique. Between the basement thrust, the Neogene sediments mainly dip about 30°E. A fourth fault is suspected in the western part, but no Tertiary sediments crop out to confirm it.
In the basement, small faults, located in the hangingwall of the main W-verging thrusts, are characterized by brittle deformation mainly parallel to the inherited metamorphic foliation. Microtectonic observations in the basement highlight an ENE–WSW contraction (sites 2, 6, 17, 23, 47; Fig. 5). This is consistent with the microtectonic observations in the sediments (ENE–WSW to ESE–WNW contraction, site 32; Fig. 5). Thus, it is most likely that the microfaults observed in the basement have the same age as the deformation seen in the late Tertiary – Quaternary sediments. Moreover, the shortening described above is also consistent with the overall N–S to NNE–SSW strike of the Pie de Palo anticline (Fig. 5), which is a late Tertiary–Quaternary feature. This also suggests that the brittle deformation observed in the basement is late Tertiary – Quaternary in age.
In addition, small-scale folds affecting both the sediments (site 32; Fig. 5) and the metamorphic foliation (sites 2, 6, 23; Fig. 5) are oriented NNW–SSE to N–S. These data also strongly suggest that all the mentioned deformation (both in the basement and the Cenozoic strata) is Plio-Quaternary in age and consistent with an overall E–W shortening.
These folds and faults affecting the basement (Fig. 8) generally present W-verging kinematics consistent with the kinematics of the two main faults. Moreover, the folds are brittle in the sense that their hinges are very localized and consist of few fractures while the limbs are very planar (Figs 8b, c, 9a). The folds also present kink geometries (Fig. 8a). These folds induce striations along the foliation planes (Fig. 9b), whose direction (roughly E–W) is also consistent with the E–W shortening. Finally, in the basement, one may also observe larger folds (metric-scale or more; Fig. 9c). These folds and their wavelength imply strong disharmony and several levels of decoupling inside the basement.

Figure 8. Field photographs of folds affecting the basement rocks. The folds display very brittle style with (a) kink geometries (site 2, Fig. 5), and (b, (c) sharp, fractured hinges with planar limbs (sites 10 and 17, respectively, Fig. 5), while the inherited ductile deformation can still be observed as boudins (c). Note that the fold axes are approximately N–S and that preferential vergence of the folds is systematically a W-vergence.

Figure 9. Field photographs of folds affecting the basement rocks. (a) Fold with sharp and fractured hinge and (b) associated foliation-parallel slip (site 2, Fig. 5). Fold and slip indicate an E–W shortening. (c) Larger-scale fold in the basement. Note the W-verging kinematics.
Parallel to the metamorphic foliation, breccias are also very frequent. They consist of brecciated elements from the basement attesting for shearing, with little or no cement (Fig. 10a). The same feature can be observed with a higher amount of calcite cement (Fig. 10b, c). In thin section, one may clearly identify the original metamorphic rocks and newly formed calcite (Fig. 11c, d).
Brecciation, shearing, and small-scale folding seem strongly controlled by the anisotropy of the basement. Indeed, these structures are linked to levels of decoupling/décollement characterized by mica-rich layers (Fig. 11a) where brittle folds are rooting. Moreover, faults such as the La Posta Fault (Fig. 5) are clearly controlled by the inherited basement foliation: at its southern end, the fault turns systematically parallel to the inherited foliation. At larger scale, the La Posta fault also follows the inherited foliation: in the northern part, both the fault and the foliation are striking NNE–SSW; in the southern part, both the fault and the foliation are N–S.
On the eastern side of the section, the Neogene sediments (Fig. 7a) are gently dipping ~5–10°E. A 720 m thick Neogene series has been described in the Niquizanga area (Cuerda, Varela & Iniguez, Reference Cuerda, Varela and Iniguez1983), south of the La Posta Ridge (Fig. 5). Accounting for the map width of these Neogene sediments and their average dip just to the north of the Niquizanga ridge, we calculated a rough estimate of 600–700 m for the total Neogene thickness including the torrential conglomerates that are conformably topping the Neogene strata. This estimate is similar to the thickness calculated by Cuerda, Varela & Iniguez (Reference Cuerda, Varela and Iniguez1983), indicating that the Neogene and basement brittle deformation, which are observed in the Niquizanga area, were formed at less than 1 km depth. Finally, no tapering structures or progressive unconformity were observed within the Neogene series. Nevertheless, the Late Neogene, Río del Camperito Formation (Cuerda, Varela & Iniguez, Reference Cuerda, Varela and Iniguez1983), which is coarser than the underlying Niquizanga Formation (see Section 3), contains metamorphic clasts from the Sierra Pie de Palo basement. These clasts indicate that these coarser Late Neogene sediments are coeval with the onset of the basement uplift.
4.b. The Ampacama section
If this area is comparable to Niquizanga in the sense that it displays several W-verging reverse faults (Fig. 6), Neogene beds have steeper dips and the basement ridges are more numerous in the Ampacama area. Indeed, we mapped seven faults: four in the northern part affecting the basement and the Neogene deposits; two in the sedimentary cover affecting both Neogene beds and Quaternary alluvial strath terraces; and one in the southern part affecting the basement and the Neogene. These faults are NNW–SSE to N–S and dip at about 40°E (Fig. 7b) and even 76°E for the southernmost one. The faults observed in the sedimentary cover may affect the basement at depth. Although this cannot be observed, it is suggested because these faults stand in the southward prolongation of the easternmost basement ridge. Most of these faults are at least 3–4 km long and have displacement up to 500 m.
The faults in both the basement and the cover witness an E–W shortening (Fig. 6), which is consistent with the microfaults reported for the southern Niquizanga area (Fig. 5).
In this area, it is noteworthy that several basement faults are parallel to the inherited metamorphic foliation, while some others are strongly oblique, or even perpendicular (Fig. 7b). Where the faults are oblique to the foliation, the latter is not characterized by a strong mineralogical segregation, i.e. the foliation is particularly not underlined by mica-rich layers (Fig. 11b).
The sedimentary layers are folded in this area, mainly as synclines between the basement ridges. Along their western limb, the dips are about 20–40°E, while in the eastern limb they can be up to 60–70°W. These geometries attest for significant asymmetries, which are also consistent with the W-verging basement thrusts.
Further west, in the Pie de Palo range, recent basement fault zones are also suspected (westernmost fault with a question mark; Figs 6, 7b). These faults are characterized by intensively fractured and faulted rocks, with a clear W-verging kinematics. However, due to the lack of Neogene sediments, their age cannot be strictly constrained. It is noteworthy that, in this area in particular as well as a few others, the basement foliation is not E-dipping, but is rather vertical to W-dipping. Further south, the foliation is also clearly folded: indeed, the orientation changes a lot in a rather restricted area (Fig. 6).
In the southernmost part of Ampacama, the sedimentary layers have dips increasing westward (from 20 to 65° and even vertical in a few places; Fig. 6, southern part). In the area where the Neogene layers are steeper (50–62°E), a sub-vertical basement slice (89°E) crops out. On its western side, a very steep (62°E) W-verging fault can be observed at the contact with the Neogene (Fig. 6). In this area, where the Neogene beds show steeper dips than in the southern Niquizanga area, the basement reverse faults affecting the Neogene also tend to be steeper.
As in the Niquizanga area, the Neogene series is composed of two sedimentary formations that are conformably capped by Early Quaternary alluvial conglomerates (Fig. 6). No detailed study is available on this Neogene series. Using the same kind of method as in the Niquizanga, we calculated two rough estimates for the Neogene maximum thickness: a first one of ~900 m to the east of the eastern basement ridge and a second one of ~1100 m on the southern part of the Neogene outcrop. These two estimates suggest that the thickness of the Ampacama Neogene series is on the order of 1000 m, hence slightly thicker than the Niquizanga Neogene formations. Nonetheless, the Ampacama thickness estimates also indicate that the Neogene deformation seen within the basement was formed under very shallow depth conditions. As in Niquizanga, the Neogene layers are characterized by lower dips on the eastern side of the section (20–35°E) while they show steeper (40–65°E) dips in the western part (Fig. 7a). No progressive unconformities or sedimentary tapering were observed in the field. Nevertheless, as in Niquizanga, the Upper Neogene Rio del Camperito Formation is coarser and contains clasts of metamorphic rocks derived from the Sierra Pie de Palo. This indicates that the basement of the Pie de Palo range was actively uplifting and under erosion during the Upper Neogene.
4.c. Synthesis
To sum up, here are our main observations. At two locations, small (in both length and displacement) W-verging reverse faults are mapped east of the Sierra Pie de Palo: these faults have lengths ranging between 2 and 6–7 km but can hardly reach 10 km; their displacements are usually less than c. 500 m. Compared to the length of the Pie de Palo Range, these faults are typically second-order structures. Most of these faults reactivated the inherited metamorphic foliation and/or shear zones, especially along mica-rich levels with an oriented fabric (Fig. 11a). Along these planes, close to and above the reverse faults, we observed foliation-parallel slip, breccias and folded foliation planes in between. The kinematics of these structures is consistent with both the microfaults measured within the sediments and the faults showing basement over sediments, as well as the overall geometry of the Pie de Palo range. This kinematics is also similar to that obtained from the inversion of earthquake focal mechanisms (Siame et al. Reference Siame, Bellier, Sebrier and Araujo2005). It is noteworthy that this top-to-the-west kinematics is most likely not inherited from pre-Andean times, as Palaeozoic top-to-the-east kinematics and associated lineations were observed in the Niquizanga shear zone. Thus, the reverse, top-to-the-west movement is most probably Neogene.
These W-verging faults have displacement ranging between a couple of hundred metres and 500 m. In some places (Fig. 6, southern part), the upward fault propagation is accompanied by gentle folds within the Neogene. Displacements in the Quaternary strath terraces are always much lower than within the Neogene formations. The northward prolongation of the Niquizanga basement ridge is at the limit of identification within the Quaternary alluvial surface. On the other hand, the surface of the Quaternary alluvial does not appear affected by the northward prolongation of the La Posta Ridge, while the underlying Neogene is still tilted (Fig. 5). In the Ampacama area, where the Neogene beds are more deformed, a tier of Middle to Late Quaternary strath terraces is conspicuously gently folded, the highest, hence oldest, surface terrace showing steeper dips than the younger ones. Nevertheless, the Quaternary terraces’ displacements and dips are much smaller than those observed within the Neogene. All these data provide clear evidence that these W-vergent reverse faults are typically early within the deformation timing of the Sierra Pie de Palo. Moreover, the fact that steeper fault dips are always associated with the steeper Neogene dips also strongly suggests that these second-order faults are early in the folding timing of the Sierra Pie de Palo
In between the two described areas, neither basement rocks nor reverse faults are clearly identified along the central-eastern side of the Sierra Pie de Palo. The only dubious candidate for such a faulted basement ridge could stand in the Casas Viejas area (31.38°S, 67.81°W) (Fig. 3b). However, it is difficult in this area to distinguish between the Neogene beds and a highly hydrothermal-altered basement. Either such basement ridges are present, but buried below the Neogene beds, or they did not develop in the same way in the central part of the fold as in the areas of Niquizanga and Ampacama. It is worth mentioning that similar range-facing scarps, striking NW–SE, are also observed within Quaternary alluvial fans of the NE corner of the Pie de Palo range (31.09°S, 67.8°W), 5–7 km to the SE of the Pajaritos fault (Figs 2, 3).
All these observations suggest that E–W shortening was accommodated at the surface in several places by shear along foliation planes and inherited shear zones. In the next section, we detail how these newly created shear zones reactivating the foliation may evolve through time, how the Sierra Pie de Palo developed and what implications this has for the crustal structure.
5. Mode of upper crustal shortening
5.a. Foliation-parallel shear
We showed above that most of the small reverse faults observed along the eastern side of the Sierra Pie de Palo initiated as foliation-parallel shear zone, controlled by (i) the inherited anisotropy of the foliation and (ii) the presence of low-strength mineral phases such as micas.
Micas are weak mineral phases, and few experimental works have underlined that mica-rich aggregates are weaker than other rocks (such as quartzo-feldspathic ones) over a broad range of mid-crustal depths (Shea & Kronenberg, Reference Shea and Kronenberg1992). Although the deformation at Sierra Pie de Palo occurred at much shallower depth than mid-crust, we may assume that the micas also behave as weak phases at shallow depths. In this case, they would allow accommodation of a large amount of strain, hence localizing the deformation and constituting shear zones.
Probably more significant than the intrinsic mechanical properties of these mineral phases, their anisotropy has been shown to control the rock strength. Indeed, in rock mechanics experiments, the weakening is controlled by the orientation of micas relative to the stress orientation (Shea & Kronenberg, Reference Shea and Kronenberg1993). This means that the softening is controlled by both the presence of micas (or any other phyllosilicate) and, more importantly, their arrangement (e.g. Bos & Spiers, Reference Bos and Spiers2001, Reference Bos and Spiers2002; Niemeijer & Spiers, Reference Niemeijer, Spiers, Bruhn and Burlini2005; Jefferies et al. Reference Jefferies, Holdsworth, Wibberley, Shimamoto, Spiers, Niemeijer and Lloyd2006; Collettini et al. Reference Collettini, Niemeijer, Viti and Marone2009; Niemeijer, Marone & Elsworth, Reference Niemeijer, Marone and Elsworth2010; Holdsworth et al. Reference Holdsworth, van Diggelen, Spiers, de Bresser, Walker and Bowen2011).
Hereafter, we propose a field-based conceptual model for the evolution of mica-rich foliated basement rocks deformed at low depths (Fig. 12). Initially, the foliation has overall a shallow dip (i.e. before Late Neogene folding), then it is activated as reverse shear zones (Fig. 12b) on weak mica layers. At small scale, these weak layers act as local décollement, and thin quartzo-feldspathic layers start forming asymmetric folds consistent with the sense of shear (Fig. 12b). If deformation continues, the folded thin quartzo-feldspathic layers evolve as breccias subsequently more or less cemented by calcite (Fig. 12c). Ultimately, this zone can turn into a localized zone of shear. Thus, at larger scale, once this zone can accommodate significant deformation, it may act as a décollement level for larger folds involving a thicker pile of metamorphic rocks (Figs 9c, 12d). This model accounts for the discrete shear zones/faults, large as well as small folds observed in the field.

Figure 12. Conceptual model of shear zone development. (a) Inherited metamorphic foliation with both quartzo-feldspathic and mica-rich layers. (b) Shearing induces disharmony with folding of thin quartzo-feldspathic layers embedded between mica-rich layers that most likely accommodate foliation-parallel shear. Layer-parallel shortening of the upper quartzo-feldpsathic layer is represented for balancing purposes, although this has not been clearly observed in the field. (c) Breccias resulting from the breakdown of the folded quartzo-feldspathic layer. The shearing localizes in this brecciated zone. (d) Ultimately, this brecciated layer may evolve as a new local décollement allowing the development of larger folds affecting the upper quartzo-feldspathic layers.
This model assumes that the deformation seen in the hangingwall of the main reverse faults can be interpreted as ‘immature’ structures that formed the reverse faults. Thus, foliation-parallel slip was localized along the main reverse faults as well as distributed in their hangingwall (and footwall?). Additionally, some reverse faults are simply the reactivation of inherited shear zones (see above, Niquizanga thrust). The reactivation of other inherited Pampean thrusts/shear zones was already suggested from seismic line interpretation in the W-verging model (Vergés et al. Reference Vergés, Ramos, Meigs, Cristallini, Bettini and Cortés2007).
As mentioned in Section 4, these structures formed at very shallow depth, i.e. c. 1 km, based on the estimated thickness of the Neogene sediments around Sierra Pie de Palo. This explains why only very brittle structures are observed, such as very localized shear zones, faults, brittle folds with very localized and fractured hinges, planar limbs, and breccias (Figs 8–10).
At such shallow depth, the basement rocks are usually deformed in a very localized mode, i.e. with large faults or a series of smaller ones (e.g. Scholz, Reference Scholz2002; Handy, Hirth & Hovius, Reference Handy, Hirth, Hovius, Handy, Firth and Hovius2007). For the Pie de Palo Range, along with the small thrusts mapped in the field, the main feature is the anticline shape of this range, especially highlighted by relicts of the pre-Neogene erosional surface (Siame et al. Reference Siame, Sebrier, Bellier, Bourlès, Costa, Ahumada, Gardini and Cisneros2015). In the next section, we discuss the mode and kinematics of such a basement shortening.
5.b. Fault vergence at the Sierra Pie de Palo
The deformation of the eastern Sierra Pie de Palo consists of several W-verging reverse faults. These faults, which have small lengths (2–8 km) and small displacements (less than 0.2 to 0.5 km), are narrowly spaced (0.5–3 km) and correspond mostly to foliation-parallel, brittle shear zones that start developing within weak foliation levels of the basement during the early stage of folding. Consequently, their significance needs to be discussed in the framework of the folding models which have been proposed for the Pie de Palo anticline, i.e. W-verging fault-bend fold (Ramos, Cristallini & Pérez, Reference Ramos, Cristallini and Pérez2002; Vergés et al. Reference Vergés, Ramos, Meigs, Cristallini, Bettini and Cortés2007) or E-verging fault-propagation fold whose blind thrust branches at depth on a W-verging thrust (Siame et al. Reference Siame, Bellier, Sebrier, Bourlès, Leturmy, Perez and Araujo2002; Siame, Bellier & Sebrier, Reference Siame, Bellier and Sebrier2006) or not (Kadinski-Cade and Reilinger, Reference Reilinger and Kadinsky-Cade1985), especially in terms of the mechanism that distributes deformation in the basement.
According to Ramos, Cristallini & Pérez (Reference Ramos, Cristallini and Pérez2002), the W-verging Niquizanga Fault (at Niquizanga Ridge; Figs 5, 7), which is located at the rear of the Pie de Palo fault-bend fold, is a rather steep reverse fault ramping off below the eastern South Bermejo Basin, at more than 20 km depth. Such a fault contradicts the long-term topography (the southern Bermejo Valley should be uplifted and the Sierra Pie de Palo should be subsiding) and could only fit with an out-of-sequence structure. However, this option disagrees with our observations because the W-verging faults have short lengths, appear tilted with the sedimentary bedding and can thus hardly ramp off at 20 km. Moreover, their displacement pre-dates or more likely is coeval with the folding, hence partly early in the deformation timing of the Pie de Palo Anticline.
Another alternative associated with a fault-bend fold might correspond to what was proposed for the Alpine basement units in the Pyrenees Axial Zone (Casas et al. Reference Casas, Oliva, Roman-Berdiel and Pueyo2003). There, steep Meso-Cenozoic faults affect both the basement and its Mezosoic cover. They are interpreted as faults initiated in the basement where it passed above a flat–ramp–flat system. Indeed, in such a case, the structural configuration and kinematics implies internal deformation within the basement (Casas et al. Reference Casas, Oliva, Roman-Berdiel and Pueyo2003). Such a model could be contemplated for the Pie de Palo anticline assuming a fault-bend fold (Ramos, Cristallini & Pérez, Reference Ramos, Cristallini and Pérez2002; Vergés et al. Reference Vergés, Ramos, Meigs, Cristallini, Bettini and Cortés2007). Where the basement passes above a flat–ramp or a ramp–flat transition, there might be either contraction or extension in the upper unit, respectively. The W-verging reverse faults observed in the field might then witness this later configuration. However, this would imply that they formed at depth, as the flat–ramp transition is in the deep crust. This is unlikely given the brittle structures observed in the field.
Considering that these small W-verging faults are observed in the eastern part of the Pie de Palo anticline, which corresponds to a forelimb accounting for the shape of the erosional surface (Fig. 3), makes it necessary to test the E-verging fault propagation fold model. In such a model, the W-verging faults could represent intercutaneous structures such as have been observed at several places (e.g. Mackay et al. Reference Mackay, Varsek, Kubli, Dechesne, Newson and Reid1996). If so, the slip on an E-verging blind basement fault would be distributed on several W-verging small basement faults. However, one would expects such an intercutaneous wedge structure to develop rather late in the deformation timing. Thus, this option remains possible, but not very likely because our observations suggest these small W-verging faults started to develop early in the sequence of shortening.
Considering that these small W-verging reverse faults are located in the forelimb of the Pie de Palo anticline, where the dips are steepest (30–90°), one can surmise that they are linked to a mechanism of folding. This fault pattern being due to foliation-parallel slip resembles the bedding-parallel slip in the flexural-slip fold model. The role of basement pre-existing fabric has been highlighted in several basement-cored folds (Schmidt, Genovese & Chase, Reference Schmidt, Genovese, Chase, Schmidt, Chase and Erslev1993). The mode of folding described above may belong to ‘mode 2’ of Schmidt, Genovese & Chase (Reference Schmidt, Genovese, Chase, Schmidt, Chase and Erslev1993). However, in this latter work, the fabric allowed the deformation to be distributed in the basement in the sense that the zone of deformation below the forelimb is large. Nonetheless, the mode of deformation is not folding s.s. It rather corresponds to a distributed mode of faulting that induced a fold in the overlying sedimentary cover.
Such a zone of deformation in the basement has been described in a few places and often interpreted as a triangular shear zone, large toward the basement top, rooting and thinning downward in the upper tip of a thrust. Such a model has been generalized for the sedimentary rocks by Erslev (Reference Erslev1991) as a trishear mode of folding and applied for the basement to explain distributed faulting below the basement/cover interface (Bump, Reference Bump2003; Garcia & Davis, Reference Garcia and Davis2004). If the fault upper tip is initially located in the basement, there might be, near the fault tip, a triangular zone of distributed deformation active until the fault potentially propagates to the basement/cover interface. In this zone, the basement may deform by pervasive faults and fractures, either pre-existing or newly created (Garcia & Davis, Reference Garcia and Davis2004); this induces a curved basement/cover interface because of distributed deformation. If such a model applies for Sierra Pie de Palo, an E-verging basement thrust should terminate upward with a distributed zone of shear characterized by W-verging small faults.
Such switch of vergence makes the trishear model unlikely for the Sierra Pie de Palo, although it cannot be ruled out. Thus, finally, the small W-verging faults more probably witness a foliation parallel-slip mode of folding associated with an E-verging fault-propagation fold, similar to what has been proposed for sedimentary rocks (Suppe & Medwedeff, Reference Suppe and Medwedeff1990). Above the blind thrust tip, a fold developed to accommodate shortening. In this fold, the mode of folding, i.e. the way the distributed deformation is accommodated, is characterized by slip along inherited foliation planes (or inherited shear zones). Even if our observations cannot provide any kind of evidence to root a trishear model, one cannot totally discard it where the E-verging fault tip propagates at depth. In such a case it would coexist with the foliation-parallel slip model we propose for the Pie de Palo anticline.
Interestingly, unfolding the Neogene Pie de Palo anticline reveals a pre-existing series of antiforms and synforms (Fig. 13a) suggesting that the Neogene and Quaternary shortening should have amplified inherited basement antiformal geometries (Fig. 13b): in the western part of the sierra, the inherited foliation appears folded, with dips much higher than the erosional surface (Figs 3, 7, 13). In the western Pie de Palo limb, several pre-Andean E-dipping thrusts are mapped and most of them are associated with E-dipping foliation (e.g. Mulcahy et al. Reference Mulcahy, Roeske, McClelland, Jourdan, Iriondo, Renne, Vervoort and Vujovich2011; Van Staal et al. Reference Van Staal, Vujovich and Currie2011). However, W-dipping foliation can also be observed in some places (e.g. Mulcahy et al. Reference Mulcahy, Roeske, McClelland, Jourdan, Iriondo, Renne, Vervoort and Vujovich2011; Van Staal et al. Reference Van Staal, Vujovich and Currie2011), especially at the sierra top (Ramos & Vujovich, Reference Ramos and Vujovich2000). Thus, in the western limb, a series of antiforms and synforms of foliation is inherited, while at the sierra top and in the eastern limb a single asymmetric antiform is inherited from a pre-Andean event (Fig. 13). Thus, we propose that the foliation-parallel slip mechanism for folding is relevant and could be the main folding mechanism determining the present-day shape of the Pie de Palo anticline. In such a case, as mentioned above, this mechanism would somewhat resemble the flexural-slip model, hence, foliation-parallel slip in the basement would play the role of bedding-parallel slip in the cover. To accommodate the shortening in a flexural-slip fold, there is partitioning between bedding-parallel slip and layer internal deformation (fractures, veins, pressure-solution, twinning). In our model of the foliation-parallel slip mode of folding, there is also a partitioning between slip along inherited foliation planes (reverse faults; Figs 7, 12, 13) and internal deformation of basement rocks (here, fractures mainly; Figs 8 and 9). Such deformation is most likely similar to that observed in the field: ‘brittle’ folds with planar limbs and localized hinges (Figs 8 and 9).

Figure 13. (a) Cross-section of the Pie de Palo (see Fig. 3 for location) and (b) its restoration. In (a) the foliation traces are from field observation and geological maps (see Fig. 3) for the surface ones. East of Pie de Palo, at depth, the inherited foliation is projected southward from seismic lines located NE of Pie de Palo (Zapata, Reference Zapata1998). Seismic foci in the northern part of the Pie de Palo (Regnier et al. Reference Regnier, Chatelain, Smalley, Chiu, Isacks and Araujo1992) were projected on the section (dots). Pal. for Palaeozoic; Neog. for Neogene.
It is noteworthy that the reverse faults interpreted as witnessing flexural-slip mechanism are spaced a few hundred metre to a couple of km apart (Figs 5, 6). This fits well with the fact that the displacements along these faults are on the order of about a few hundred metres (Fig. 7), as predicted by Alonso (Reference Alonso1989).
In some places where the foliation was previously intensively folded, at smaller scale than the basement antiform (see spatial variations of foliation orientation in Fig. 5), new faults were laterally propagated and created oblique to the local foliation, to ensure a kinematics consistent at fold scale.
Therefore, the W-verging small reverse faults seen along the eastern side of the Pie de Palo anticline agree with an E-verging fault propagation fold. Moreover, the dip of the Neogene series in the eastern Pie de Palo is very high at some places (more than 60°; Fig. 6), which does not fit well with a fault-bend fold model where such dips should represent the dip of the W-verging thrust. Thus, although the W-verging model cannot be totally discarded we have shown that structural data permit the suggestion of an alternative model with a major crustal blind thrust and an associated fault-propagation fold (Figs 13, 14). Moreover, this surmised major crustal blind thrust is quite consistent with local microseismic data (Regnier et al. Reference Regnier, Chatelain, Smalley, Chiu, Isacks and Araujo1992) showing a W-dipping alignment of foci (average dip around 30–35°E), especially in the northern part of Pie de Palo (Figs 4a, 13, 14). Two mechanisms can fit with this latter structural model: intercutaneous faults or foliation-parallel slip mechanism, this second mechanism being much more likely. Finally, the Neogene Pie de Palo fold appears to have developed on inherited basement antiformal and synformal geometries.

Figure 14. Crustal-scale cross-section of the Sierra Pie de Palo, the Sierra Valle Fértil and the Precordillera (see Fig. 1b for location). (a) From Vergés et al. (Reference Vergés, Ramos, Meigs, Cristallini, Bettini and Cortés2007); (b) in Siame et al. (Reference Siame, Sebrier, Bellier, Bourlès, Costa, Ahumada, Gardini and Cisneros2015); and (c) this study. In (c), the front of the Precordillera has been modified after Alonso et al. (Reference Alonso, Gallastegui, Rodriguez Fernandez and Garcia-Sansegundo2014). The asterisk near the star along the western crustal E-verging thrust represents an alternative solution for the 1944 focus (from Meigs and Nabelek, Reference Meigs and Nabelek2010). The 1977M earthquake focus have been translated 30 km to the west as suggested in Regnier et al. (Reference Regnier, Chatelain, Smalley, Chiu, Isacks and Araujo1992).
6. Crustal and lithospheric structure
Our main results are twofold: first, we show how the inherited metamorphic foliation controlled and influenced the formation of small faults in the basement, and second, we argue that the overall kinematics of the Sierra Pie de Palo as well as the depth distribution of seismic foci are consistent with an E-verging fault-propagation crustal fold. In the following sections, we propose a more speculative discussion at both crustal and lithospheric scales.
6.a. Crustal implications
We have proposed that the dominant folding mechanism should be foliation-parallel slip and that it induced the formation of small reverse faults, the foliation being not parallel to the basement/cover interface. Such interpretation has also been proposed for other Andean structures (e.g. Alonso et al. Reference Alonso, Rodriguez_Fernandez, Garcia-Sansegundo, Heredia, Farias and Gallastegui2005). Alternatively, these small structures may also be due to intercutaneous wedge processes. However, in both cases the resulting crustal thrust should be E-verging. It is noteworthy that an E-verging blind thrust below the Sierra Pie de Palo is opposite to the main kinematics of the Pampean province.
According to seismological data, we consider here that the E-verging blind thrust below the Sierra Pie de Palo should root at the base or below the upper seismogenic crust, i.e. at ~40 km (Fig. 14c) as already proposed by Siame et al. (Reference Siame, Sebrier, Bellier, Bourlès, Costa, Ahumada, Gardini and Cisneros2015) for a slightly different structural interpretation (Fig. 14b). In our model, the thrust to the SW of the Sierra Pie de Palo (Tulum Fault) is considered as a back thrust branched at depth on the main E-verging thrust. This configuration is in agreement with the seismicity (Figs 13, 14), especially considering that the 1977 earthquake should be located 30 km west (Fig. 14c) of its initial location (Fig. 14a, b) as stated in Regnier et al. (Reference Regnier, Chatelain, Smalley, Chiu, Isacks and Araujo1992). The 20°E dip of the erosional surface (backlimb west of the Tulum W-verging thrust, below the Neogene sediments of the Tulum valley; Figs 2, 13a, 14) thus approximately represents the dip of the E-verging thrust at depth, consistent with the model of fault-propagation fold.
The E-verging thrust ramps off a deep crustal décollement (either at the base of the seismogenic crust or in the lower crust; Fig. 14c) as well as the E-verging basement thrust that activated the Eastern Precordillera (Meigs et al. Reference Meigs, Krugh, Schiffman, Vergés and Ramos2006). This Precordillera is W-verging because of the change of vergence in the décollement at the base of the cover (Fig. 14c). This is consistent with the interpretation of Vergés et al. (Reference Vergés, Ramos, Meigs, Cristallini, Bettini and Cortés2007), Meigs et al. (Reference Meigs, Krugh, Schiffman, Vergés and Ramos2006) and Rockwell et al. (Reference Rockwell, Ragona, Meigs, Owen, Costa and Ahumada2014), if we consider that the 1944 earthquake is due to a displacement at the cover base where the thrust vergence changes (Fig. 14c) at 9–11 km (Alvarado & Beck, Reference Alvarado and Beck2006; Meigs et al. Reference Meigs, Krugh, Schiffman, Vergés and Ramos2006). Alternatively, this 1944 earthquake could have ruptured deeper (~25 km) on the E-verging basement ramp (Meigs & Nabelek, Reference Meigs and Nabelek2010), which is located below the Eastern Precordillera (Smalley et al. Reference Smalley, Pujol, Regnier, Chiu, Chatelain, Isacks, Araujo and Puebla1993) (Fig. 14c).
These two E-verging structures, which are located below the Eastern Precordillera and the Pie de Palo Anticline, stand between the thin-skinned Western and Central Precordillera to the west and the Pampean W-verging Sierra de Valle Fértil to the east. It is noteworthy that the crust below the Sierra Pie de Palo and the Valle Fértil is considered as different (e.g. Ramos, Reference Ramos2004): the crust below Valle Fértil and further east is Pampia, while below Sierra Pie de Palo and the Precordillera it is Cuyania. Thus, there might be a link between these different crusts and the different vergences in these domains: in the Cuyania domain, the thrusts are E-verging at the surface (except in the Eastern Precordillera) while in the Pampia crust the thrusts appear predominantly W-verging.
6.b. Lithospheric structure
In the North American Laramide province of the Rocky Mountains, the presence of large basement uplift (similar to the Sierras Pampeanas; e.g. Jordan & Allmendinger, Reference Jordan and Allmendinger1986) is probably due to subcrustal shear, crustal buckling and faulting above a décollement in the lower crust, or crustal buckling and faulting superimposed on a lithosphere buckling (Erslev, Reference Erslev, Kalstrom and Keller2005; Yonkee & Weil, Reference Yonkee and Weil2015; see a synthesis in Lacombe & Bellahsen, Reference Lacombe and Bellahsen2016). In all cases, the driving force arises from the push of a flat slab transmitting stresses far in the interior of the overriding plate. In the Andean foreland of NW Argentina, both vergences are found in the mountain range and are most likely controlled by inherited structures (Ramos, Cristallini & Pérez, Reference Ramos, Cristallini and Pérez2002).
The E-verging thrusts beneath the Sierra Pie de Palo and the Eastern Precordillera could be considered as a ‘normal kinematics’: they are the eastward thick-skinned propagation at depth of the E-verging thin-skinned system of the Precordillera. As classically considered, the flat slab geometry induced stresses to be propagated further down in the crust and to the east. The W-verging Pampean structures (such as Valle Fértil) are strongly controlled by structural inheritance, i.e. E-dipping inherited Triassic normal faults that are reactivated during the Neogene and Quaternary (Ramos, Cristallini & Pérez, Reference Ramos, Cristallini and Pérez2002).
The Cuyania crust below the Sierra Pie de Palo is very thick (52–60 km), while the thickness of the Pampean crust, further east, decreases eastward from ~50 km to 35 km (Ammirati et al. Reference Ammirati, Alvarado, Perarnau, Saez and Monsalvo2013; Ammirati, Alvarado & Beck, Reference Ammirati, Alvarado and Beck2015). Moreover, these authors interpreted the Cuyania lower crust as eclogitized. As the shortening is rather low in this region (Fig. 13), it cannot only explain the eclogitization; it should rather result from the initial thickness of the Cuyania crust. Thus, it is likely that Cuyania was already quite thick, at least in its eastern part, before the Neogene shortening.
Below the Precordillera, the crust is thicker (~65 km; e.g. Ammirati et al. Reference Ammirati, Alvarado, Perarnau, Saez and Monsalvo2013). Considering the amount of shortening in the Precordillera (135 km), the Cuyania crust must have been significantly underthrusted below the Andean Cordillera, i.e. below the Chilenia upper crust (Cristallini & Ramos, Reference Cristallini and Ramos2000). Thus, it is likely that, for balancing purpose, the Chilenia lower crust was underthrusted below Cuyania (Cristallini & Ramos, Reference Cristallini and Ramos2000) (Fig. 15). This process is coeval with the Tertiary formation of the Principal and Frontal Cordilleras as well as the Western and Central Precordilleras. During this process, the Chilenia lower crust was possibly eclogitized as well as the Cuyania lower crust further east.

Figure 15. Lithospheric-scale section of the Andes modified from Cristallini and Ramos (Reference Cristallini and Ramos2000) (see Fig. 1a for location). The crustal structure at Pie de Palo and below the Precordillera were modified according to the present study. The Moho and lower crustal structure (eclogitized root) are from Ammirati et al. (Reference Ammirati, Alvarado, Perarnau, Saez and Monsalvo2013) and Ammirati, Alvarado & Beck (Reference Ammirati, Alvarado and Beck2015). The Eastern Sierras Pampeanas structure is from Ramos et al. (Reference Ramos, Cristallini and Pérez2002) and Perarnau et al. (Reference Perarnau, Gilbert, Alvarado, Martino and Anderson2012).
When the slab dip decreased, at c. 10 Ma, the stresses were transferred to the Cuyania and Pampia crust. It is noteworthy that it may also correspond to the period when the crust below the Cordillera and Precordillera became too thick and too strong because of its eclogitized root. The shortening propagated through a deep décollement between the seismogenic crust and the eclogitized root, in the Cuyania crust at ~40 km depth, and furthermost to the Sierra de Córdoba region within the Pampia / Río de la Plata crusts at c. 35 km depth.
7. Conclusions
We have investigated the small W-verging reverse faults along the eastern side of the Sierra Pie de Palo anticline. We showed that these faults are less than 8 km long and have displacement of a few hundred metres. These faults are thus relatively shallow and can hardly root in the deep crust as previously proposed (e.g. Ramos, Cristallini & Pérez, Reference Ramos, Cristallini and Pérez2002). These faults show typical brittle structures in the basement. They initiated in the basement by shearing along inherited weak foliation levels or inherited shear zones, particularly within mica-rich layers, inducing a small-scale folding of quartzo-feldspathic layers, and subsequently their brecciation. They evolved as thrust faults propagating in the Neogene cover as thrusts or folds, and are even faulting Quaternary strath terraces.
These faults, which are only seen along the eastern side of the Sierra Pie de Palo, may attest for basement folding at crustal scale, with foliation-parallel slip as folding mechanism, although alternative models may also be considered. The highly deformed eastern Sierra Pie de Palo most likely represents a forelimb and thus suggests for E-verging blind thrust at depth. This vergence is opposite to the common Pampean vergence but may be considered typical for Cuyania crust.
Such E-verging thrust may be rooting at depth westward beneath the Andean Cordillera (as well as the other Pampean W-verging thrusts, linked through a mid- to lower-crustal décollement) where the Chilenia lower crust is underthrusted and eclogitized below the Cuyania crust.
Acknowledgements
This work was done within a joint programme between ISTeP (Sorbonne University – University Pierre and Marie Curie, Paris) and CEREGE (Aix-en-Provence). SPOT images were provided by the Tectoscope-Andes and ISIS programmes (CNES/INSU/CNRS). We thank the ECOS-SUD programme for travel grants made to N. Bellahsen, M. Sebrier and L. Siame for field work in Argentina. We are indebted to Carlos Costa (Universidad Nacional de San Luis) for logistical help and many discussions on the geology of the Sierra Pampeanas and Argentina in general. We also thank E. Ahumada and H. Cisneros for invaluable help during fieldwork and J. L. Alonso and an anonymous reviewer for comments that greatly improved the initial version of the manuscript.