Introduction
Since the seminal McKay et al. (Reference McKay, Gibson, Thomas-Keprta, Vali, Romanek, Clemett, Chillier, Maechling and Zare1996) paper on possible traces of life in Martian meteorite ALH84001 (Fig. 1), we have come a long way in understanding the nature of biosignatures and their preservation, through experimentation, high-resolution analysis and Mars analogue studies. McKay et al. based their conclusions on three distinct phenomena that they considered to pertain to life: (1) globular and linear mineral features that were interpreted as nanobacteria (nanobacteria were all the ‘rage’ at that period following the works of Robert Folk; e.g. Folk and Lynch, Reference Folk and Lynch1997; Folk and Lynch, Reference Folk and Lynch2001); (2) the presence of polycyclic aromatic hydrocarbons (PAHs) interpreted as the remains of carbonaceous life forms; and (3) the presence of tiny magnetite crystals very similar to those produced by terrestrial magnetotactic bacteria. In addition, all of these features occurred in association with Fe/Mg globules deposited within fractures in the meteorites that they considered to have formed via low-temperature aqueous deposition, i.e. the cumulate volcanic rocks from which the meteorite was ejected had water percolating through their fractures, just as in terrestrial surface rocks. The temperature of deposition was later confirmed by Halevy et al. (Reference Halevy, Fischer and Eiler2011) to be ~18 °C. Another important factor in the equation is the age of the meteorite: originally dated at ~4.5 Ga, subsequent investigations established an age of 4.09 Ga (Lapen et al., Reference Lapen, Righter, Brandon, Debaille, Beard, Shafer and Peslier2010), with the age of the carbonate globules dated to ~4 Ga (Borg et al., Reference Borg, Connelly, Nyquist, Shih, Wiesmann and Reese1999). The geological and environmental contexts were consistent with the presence of life forms in water-filled crustal fractures. Thus, the McKay group based their interpretation on multiple types of biogenicity criteria – morphology, organic chemistry and biomineralization, a basic prerequisite in this type of study.
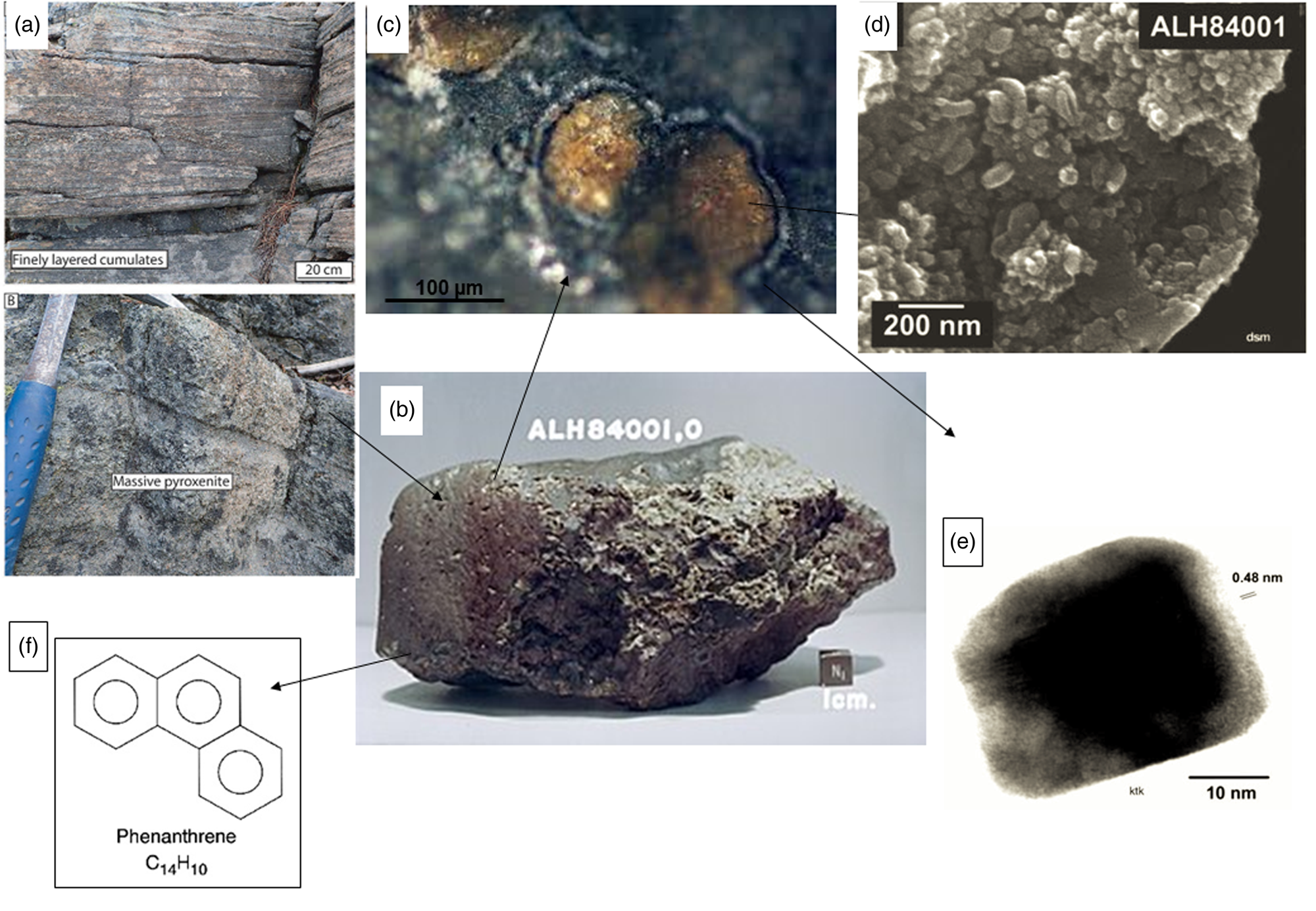
Fig. 1. Characteristics of the ALH84001 meteorite and its interpreted biosignatures. (a) Pyroxenite, similar to the parent rock of the meteorite (at ARC Centre of Excellence for Core to Crust Fluid Systems). (b) The ALH84001 meteorite (NASA). (c) Rosettes of Fe carbonate (orange), Mg carbonate (white) and magnetite between the two (black) (NASA). (d) Globular-shaped carbonate structures interpreted to be nanobacteria (Gibson et al., Reference Gibson, McKay, Thomas-Keprta, Wentworth, Westall, Steele, Romanek, Bell and Toporski2001; Elsevier, permission). (e) A tiny magnetite nanocrystal from the ALH84001 meteorite (Gibson et al., Reference Gibson, McKay, Thomas-Keprta, Wentworth, Westall, Steele, Romanek, Bell and Toporski2001; Elsevier, permission). (f) Structure of phenanthrene, one of the organic compounds found in the meteorite (Wikipedia).
The original investigation was, at the time, state of the art. Lacking was sufficient understanding of the nature of primitive microorganisms and their processes of fossilization, which has come with subsequent research. It was later determined that the carbonate ‘nanofossils’ were mineralogical artefacts (Gibson et al., Reference Gibson, McKay, Thomas-Keprta, Wentworth, Westall, Steele, Romanek, Bell and Toporski2001). Isotopic analysis established that some of the organic molecules in the meteorite that fell on the Antarctic glacier were of terrestrial origin (Jull et al., Reference Jull, Courtney, Jeffrey and Beck1998) but there was still the question of the origin of the PAHs. These kinds of organic molecules are generic and ubiquitous. PAHs occur in carbonaceous meteorites (Sephton, Reference Sephton2002) and they have been documented in Martian surface materials at Gale Crater by the instrument SAM on the NASA Mars Science Laboratory Curiosity rover (Eigenbrode et al., Reference Eigenbrode, Summons, Steele, Freissinet, Millan, Navarro-González, Sutter, McAdam, Franz, Glavin, Archer, Mahaffy, Conrad, Hurowitz, Grotzinger, Gupta, Ming, Sumner, Szopa, Malespin, Buch and Coll2018). Steele et al. (Reference Steele, McCubbin, Fries, Kater, Boctor, Fogel, Conrad, Glamoclija, Spencer, Morrow, Hammond, Zare, Vicenzi, Siljeström, Bowden, Herd, Mysen, Shirey, Amundsen, Treiman, Bullock and Jull2012) found evidence of mantle carbon in the ALH84001 meteorite, as well as in other Martian meteorites. Thus, abiotic processes could explain the presence of the PAHs. There has been more controversy over the nature of the tiny magnetite crystals, with the group of David McKay pushing the frontiers of analytical techniques to document the similarities between these crystals and those of magnetotactic microbes (Thomas-Keprta et al., Reference Thomas-Keprta, Clemett, McKay, Gibson and Wentworth2009); however, others have demonstrated the formation of magnetite crystallites with similar ‘biosignatures’ (e.g. high crystallinity, chemical purity, a single-domain magnetic structure, and well-defined crystal morphology) through abiotic processes (Golden et al., Reference Golden, Ming, Morris, Brearley, Lauer, Treiman, Zolensky, Schwandt, Lofgren and McKay2004; Till et al., Reference Till, Guyodo, Lagroix, Morin, Menguy and Ona-Nguema2017). The salutary conclusion of these investigations is the degree of detail and stringency necessary to determine the all-important criterion of biogenicity. With the exception of the organic analyses, none of this would have been possible in situ on Mars.
Despite subsequent evidence showing that plausible non-biological processes can lead to the formation of each of the individual ‘biosignatures’ identified by McKay et al. (Reference McKay, Gibson, Thomas-Keprta, Vali, Romanek, Clemett, Chillier, Maechling and Zare1996), this seminal paper kick-started the field of astrobiology. Today we have a far better understanding of microbes and how their traces can be preserved. We also have a deeper awareness of associated problems, such as the distinction between a microbial biosignature and a similar abiotic signature, or the necessity of demonstrating syngenicity, i.e. formation at the same time as the host rock. Importantly, since the time of the McKay study, many new techniques have been developed, or older ones adapted, to characterize biosignatures.
The discovery of traces of endogenous life in Martian rocks, either in situ or in returned samples, would have a profound effect on our view of the distribution of life in the Universe: it would suggest that the processes leading to the emergence of life may be common in the Solar System and, by extension, on rocky planets elsewhere. Importantly, we will want to understand more about the ecology of these life forms. For example, whether they lived at the time of the formation of the rock or whether they were later colonizers, for instance of pores and fractures. We will also want to learn about what kinds of metabolisms they used.
In the following, we will define the term ‘biosignature’, emphasizing the importance of geological and mineralogical context, as well as the fossilization and preservation of traces of life. Taking the example of the Alan Hills 84001 meteorite as a starting point, we will make a brief overview of preserved traces of life in different types of terrestrial sediments and mineral matrices of relevance for Mars. We will consider examples of lithologies and minerals detected on Mars, especially with consideration to the Mars 2020 mission to Jezero Crater on Mars that will take samples to return to Earth (Williford et al., Reference Williford, Farley, Stack, Allwood, Beaty, Beegle, Bhartia, Brown, de la Torre Juarez, Hamran, Hecht, Hurowitz, Rodriguez-Manfredi, Maurice, Milkovich, Wiens, Cabrol and Grin2018) and the ExoMars 2022 mission that will be searching for traces of life on Oxia Planum (Vago et al., Reference Vago, Witasse, Svedhem, Baglioni, Haldemann, Gianfiglio, Blancquaert, McCoy and de Groot2015, Reference Vago, Westall, Coates, Jaumann, Korablev, Ciarletti, Mitrofanov, Josset, De Sanctis, Bibring, Rull, Goesmann, Steininger, Goetz, Brinckerhoff, Szopa, Raulin, Westall, Edwards, Whyte, Fairén, Bibring, Bridges, Hauber, Ori, Werner, Loizeau, Kuzmin, Williams, Flahaut, Forget, Vago, Rodionov, Korablev, Svedhem, Sefton-Nash, Kminek, Lorenzoni, Joudrier, Mikhailov, Zashchirinskiy, Alexashkin, Calantropio, Merlo, Poulakis, Witasse, Bayle, Bayón, Meierhenrich, Carter, García-Ruiz, Baglioni, Haldemann, Ball, Debus, Lindner, Haessig, Monteiro, Trautner, Voland, Rebeyre, Goulty, Didot, Durrant, Zekri, Koschny, Toni, Visentin, Zwick, van Winnendael, Azkarate and Carreau2017).
Biosignatures
Biosignature definition
We define biosignatures as morphological, chemical (organic, elemental and/or mineral) and isotopic traces of organisms preserved in minerals, sediments and rocks. They represent the physical presence of the organisms as well as evidence of their metabolic activities and metabolites (Westall and Cavalazzi, Reference Westall, Cavalazzi, Thiel and Reitner2011 within put from Summons et al., Reference Summons, Amend, Bish, Buick, Cody, Des Marais, Dromart, Eigenbrode, Knoll and Sumner2011; Mustard et al., Reference Mustard, Adler, Allwood, Bass, Beaty, Bell, Brinckerhoff, Carr, Des Marais and Brake2013; Hays et al., Reference Hays, Graham, Des Marais, Hausrath, Horgan, McCollom, Parenteau, Potter-McIntyre, Williams and Lynch2017; Vago et al., Reference Vago, Westall, Coates, Jaumann, Korablev, Ciarletti, Mitrofanov, Josset, De Sanctis, Bibring, Rull, Goesmann, Steininger, Goetz, Brinckerhoff, Szopa, Raulin, Westall, Edwards, Whyte, Fairén, Bibring, Bridges, Hauber, Ori, Werner, Loizeau, Kuzmin, Williams, Flahaut, Forget, Vago, Rodionov, Korablev, Svedhem, Sefton-Nash, Kminek, Lorenzoni, Joudrier, Mikhailov, Zashchirinskiy, Alexashkin, Calantropio, Merlo, Poulakis, Witasse, Bayle, Bayón, Meierhenrich, Carter, García-Ruiz, Baglioni, Haldemann, Ball, Debus, Lindner, Haessig, Monteiro, Trautner, Voland, Rebeyre, Goulty, Didot, Durrant, Zekri, Koschny, Toni, Visentin, Zwick, van Winnendael, Azkarate and Carreau2017). Firstly, we aim to recognize the morphological expression of microorganisms, including mineral-replaced cells, colonies or microbial biofilms/mats, as well as the physical expression of the interaction of microorganisms with their sedimentary environment. Examples of this are three-dimensional microbial constructions, including microbially induced sedimentary structures (MISS, Noffke et al., Reference Noffke, Beukes, Bower, Hazen and Swift2008; Heubeck, Reference Heubeck2009; Noffke, Reference Noffke2009, Reference Noffke2010) and stromatolites (Byerly et al., Reference Byerly, Lowe and Walsh1986; Hofmann and Davidson, Reference Hofmann and Davidson1998; Allwood et al., Reference Allwood, Walter, Kamber, Marshall and Burch2006). The organic remains of microorganisms and their colonies may still be identifiable as such, if preserved under anoxic conditions, unless they have been subjected to such a high degree of metamorphism that the organic matter becomes graphitized (although carbon isotopic signatures may still be preserved in graphite). During diagenesis and metamorphism, organic molecules break up, the lower molecular weight portions degrading first leaving relics of more resistant components, such as lipids and recalcitrant biopolymers (Brocks and Summons, Reference Brocks and Summons2003). Factors influencing the composition and structure of the more recalcitrant molecules during diagenesis include oxidation, reduction, sulphurization, desulphurization and molecular rearrangement reactions. The loss of functional groups results in products with more robust aromatic and aliphatic structures that may still be identifiable as microbial hydrocarbons. Thus, the structure and composition of the degraded organic molecules can be preserved, even up to lower greenschist facies metamorphism (and occasionally even beyond if the signature is encased in a resistant mineral; Hassenkam et al., Reference Hassenkam, Andersson, Dalby, Mackenzie and Rosing2017). This preservation includes their enantiomeric signature, patterns in the odd over even carbon number, δ13C signature, diastereoisomeric preference, structural isomer preference and repeating constitutional subunits (Vago et al., Reference Vago, Westall, Coates, Jaumann, Korablev, Ciarletti, Mitrofanov, Josset, De Sanctis, Bibring, Rull, Goesmann, Steininger, Goetz, Brinckerhoff, Szopa, Raulin, Westall, Edwards, Whyte, Fairén, Bibring, Bridges, Hauber, Ori, Werner, Loizeau, Kuzmin, Williams, Flahaut, Forget, Vago, Rodionov, Korablev, Svedhem, Sefton-Nash, Kminek, Lorenzoni, Joudrier, Mikhailov, Zashchirinskiy, Alexashkin, Calantropio, Merlo, Poulakis, Witasse, Bayle, Bayón, Meierhenrich, Carter, García-Ruiz, Baglioni, Haldemann, Ball, Debus, Lindner, Haessig, Monteiro, Trautner, Voland, Rebeyre, Goulty, Didot, Durrant, Zekri, Koschny, Toni, Visentin, Zwick, van Winnendael, Azkarate and Carreau2017). These characteristics, even for relatively degraded molecules, can aid in the interpretation of biogenicity. Fortunately, given the smaller size and lower gravity of Mars, the degree of alteration of organic molecules due to metamorphism on the planet is expected to be very low, unless the rocks are affected by local impact metamorphism. It may therefore be possible to detect biomarker molecules, i.e. molecules whose structure and composition can be traced back to specific microbial precursors more easily on Mars than on Earth. To date, the oldest documented biomarkers occur in the 1.73 Ga Wollogorang Formation in the southern McArthur Basin, Australia (Vinnichenko et al., Reference Vinnichenko, Jarrett, Hope and Brocks2020). They comprise degradation products, such as C14–C19 2,3,4- and 2,3,6-trimethyl aryl isoprenoids. The latter can be correlated to carotenoids of cyanobacteria and/or green sulphur bacteria (Chlorobiaceae), while the former 2,3,4-AI series may be derived from purple sulphur bacteria (Chromatiaceae). With respect to extraterrestrial life, it is not the molecules themselves, but specific patterns that can be diagnostic, even, without knowing the nature of Martian life (if it exists), i.e. agnostic biosignatures.
Additional biogenicity signatures include those resulting from microbial metabolism, for example, the fractionation of certain isotopes, such as carbon, nitrogen or iron (Schidlowski, Reference Schidlowski2001; Teng et al., Reference Teng, Kuang, Luo and Shu2017), as well as the concentration of bio-essential elements C, H, N, O, P, S (e.g. Hickman-Lewis et al., Reference Hickman-Lewis, Garwood, Brasier, Goral, Jiang, McLoughlin and Wacey2016; Delarue et al., Reference Delarue, Robert, Derenne, Tartèse, Jauvion, Bernard, Pont, Gonzalez-Cano, Duhamel and Sugitani2020), and transition metals Fe, Ni, Cu, Mn, Co, Mo, V and As (Wogelius et al., Reference Wogelius, Manning, Barden, Edwards, Webb, Sellers, Taylor, Larson, Dodson and You2011; Hickman-Lewis et al., Reference Hickman-Lewis, Cavalazzi, Sorieul, Gautret, Foucher, Whitehouse, Jeon, Georgelin, Cockell and Westall2020a). Also resulting from metabolic activity are certain biominerals precipitated either passively or actively, including various carbonate minerals, or magnetite. Finally, the presence of microbes and their metabolic products strongly influences mineral composition, habit, dissolution and even size (Banfield et al., Reference Banfield, Moreau, Chan, Welch and Little2001).
The Allan Hills meteorite 84001 provides a salutary example of the pitfalls regarding the identification of potential biosignatures. Many of the characteristics listed above can result from abiogenic processes (Westall and Cavalazzi, Reference Westall, Cavalazzi, Thiel and Reitner2011; McMahon et al., Reference McMahon, Bosak, Grotzinger, Milliken, Summons, Daye, Newman, Fraeman, Williford and Briggs2018). Particularly with respect to possible extraterrestrial life, a multiscale, multisignature approach is favoured to enhance confidence in interpretations of biogenicity. While the above characteristics are related to life as we know it, the recently developed concept of agnostic biosignatures seeks to address the signatures of life as we do not know it. This concept concerns a broader definition of life based on activity rather than structure and is not reliant on specific biogeochemistry (Johnson et al., Reference Johnson, Anslyn, Graham, Mahaffy and Ellington2018a, Reference Johnson, Zaikova, Millan, Williams, Craft, Wagner, Bevilacqua, Bai, Fuqua and Kobs-Nawotniak2018b), concerning instead an increase in the degree of complexity of organic molecules.
The importance of geological and mineralogical context
Another aspect of biosignature study demonstrated by the ALH84001 example is that of geological and mineralogical context. The overall geological context of the meteorite is not a traditional one for life. Canonically, we expect to find traces of life associated with abundant water and, more specifically, detrital and/or chemical sediments deposited by water. This is because most primitive life forms (prokaryotes) are found in such environments. Exceptions are more evolved prokaryotes, such as cyanobacteria, that can survive in very dry environments, such as endoliths in hot or cold deserts. Indeed, Imre Friedmann, the renowned microbiologist who first identified endolithic life in the supposedly uninhabited Dry Valleys of Antarctica in the 1980s, was the first to suggest that, before disappearing from the surface of Mars altogether, Martian life, if it existed, would have adopted such endolithic habitats (Friedmann and Koriem, Reference Friedmann and Koriem1989).
In the case of the Allan Hills meteorite, the geological context is on a micro-scale, rather than a macro-scale. It is a cumulate rock, i.e. a relatively coarse-grained basaltic rock that is formed at the base of a thick lava flow. When extruded under water, the surfaces of lava flows are rapidly colonized by microorganisms as soon as they have cooled down, since organisms use them as a support for colonization, as well as a substrate from which to obtain energy through redox reactions at the water/rock interface and essential transition metals extracted from the rock (Thorseth et al., Reference Thorseth, Torsvik, Furnes and Muehlenbachs1995; Einen et al., Reference Einen, Kruber, Øvreås, Thorseth and Torsvik2006). Pores formed by gas bubbles or amygdules in the surfaces of basalt may also be colonized by microbes (Cavalazzi et al., Reference Cavalazzi, Westall, Cady, Barbieri and Foucher2011, Reference Cavalazzi, Westall, Cady, Hanslmeier, Kempe and Seckbach2012). However, it is only in the last couple of decades that we have been made aware of a vast subsurface biosphere in fractures in the terrestrial crust. Deep gold mines in South Africa showed that the subsurface down to more than 3.5 km is an important habitat where surface water trickling through fractures fuels a relatively wide array of microbial life (Hoehler and Jørgensen, Reference Hoehler and Jørgensen2013; Onstott, Reference Onstott2016). These groundwaters pick up a variety of nutrients depending on the rocks through which they flow, thus producing a distinctive fracture fluid chemistry reflecting that of the surrounding rock. They are sources of energy and nutrients for subsurface microbial life and also transport microorganisms through the subsurface (Casar et al., Reference Casar, Kruger and Osburn2021). Indeed, subsurface experiments show that iron-bearing minerals, such as pyrite and siderite, are prime sites for microbial colonization because of the high amount of energy released during redox reactions (Casar et al., Reference Casar, Kruger, Flynn, Masterson, Momper and Osburn2020). It is estimated that the continental deep subsurface is probably one of the largest reservoirs of bacteria and archaea on Earth, where microorganisms survive on minimal organic carbon sources, obtaining their energy instead from mineral sources (McMahon and Parnell, Reference McMahon and Parnell2014; Casar et al., Reference Casar, Kruger and Osburn2021). If life ever appeared on Mars, and if it still exists, extant life will most likely be found in the subsurface of the planet (Fig. 2; Onstott et al., Reference Onstott, Ehlmann, Sapers, Coleman, Ivarsson, Marlow, Neubeck and Niles2019).
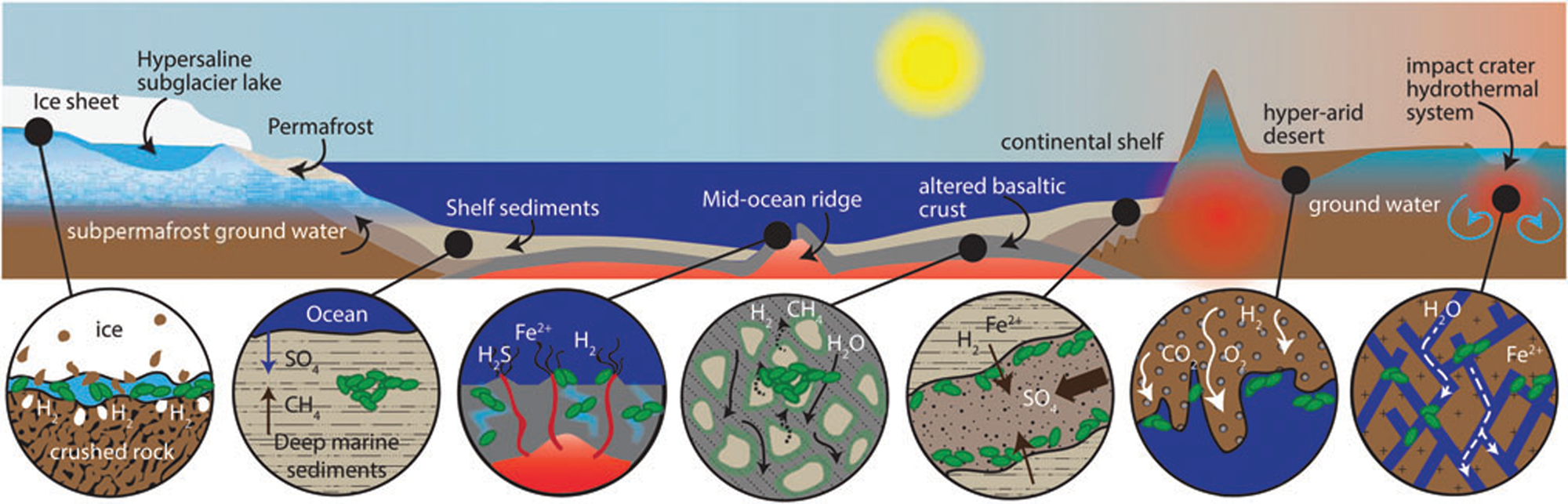
Fig. 2. Potential subsurface habitats on Mars (Onstott et al., Reference Onstott, Ehlmann, Sapers, Coleman, Ivarsson, Marlow, Neubeck and Niles2019; Liebert, permission).
The ALH84001 meteorite is petrographically classified as a coarse-grained orthopyroxenite comprising 97% orthopyroxene, 2% chromite, with interstitial plagioclase, augite, olivine, pyrite, apatite and silica, as well as maskelynite formed during impact shock and later secondary Fe-Mg-Mn-Ca carbonate (Harvey and McSween Jr, Reference Harvey and McSween1994; Mittlefehldt, Reference Mittlefehldt1994). Orthopyroxenes are Fe- and Mg-rich chain silicates while chromites are Fe- and Cr-rich oxides. Olivine, augite and pyrite are also Fe-rich minerals, as is a fraction of the secondary carbonate deposits. By virtue of this association of minerals, groundwaters flowing through the fractures of the consolidated basaltic flow would have derived sufficient nutrients (energy sources) to support a subsurface microbial biome. Carbon would have been limited, likely brought in from the surface along with the ground waters. Note that although magmatic carbon occurs in this rock as PAH-containing macromolecular carbon (Steele et al., Reference Steele, McCubbin, Fries, Kater, Boctor, Fogel, Conrad, Glamoclija, Spencer, Morrow, Hammond, Zare, Vicenzi, Siljeström, Bowden, Herd, Mysen, Shirey, Amundsen, Treiman, Bullock and Jull2012), such materials are generally insoluble organic carbon phases. Nevertheless, microbial utilization of mantle-derived carbon does occur (Lang et al., Reference Lang, Früh-Green, Bernasconi, Lilley, Proskurowski, Méhay and Butterfield2012), but it is not certain whether microbes can directly utilize this carbon or whether it first needs to be oxidized by the microbes into smaller, more assimilable phases. As indicated in the above discussion of subsurface life in the Earth's crust, a priori it is perfectly feasible that cracks in the Martian crust (or lava flows) could have supported microbial activity.
Had McKay et al.'s (Reference McKay, Gibson, Thomas-Keprta, Vali, Romanek, Clemett, Chillier, Maechling and Zare1996) hypothesis for evidence of life in ALH84001 been confirmed, the geological and mineralogical context would have allowed us to draw further conclusions as to the mode of life and metabolic activity of the microorganisms: they could have been either (1) anaerobic chemotrophs, more precisely lithotrophs possibly cycling iron and sulphur, or (2) other types of organisms (e.g. heterotrophs) flushed into the fractures during surface water flow. Further refinements would not be possible since we know nothing about putative Martian life.
This example demonstrates the importance of geological and mineralogical context for supporting hypothetical biosignature identification and interpretation and for obtaining environmental details that provide some indication as to the lifestyle of the potential organism. Of additional importance is the information that geological and mineralogical context can provide for understanding the potential for fossilization and long-term preservation of biosignatures. Here, again, the ALH84001 meteorite is a salutary example.
Fossilization and preservation of traces of life
We saw above that biosignatures may be organic, organo-mineral, morphological, isotopic or geochemical. However, in order to be preserved over long periods of geological time, any signature of life needs to be encased or cemented within its mineralogical context and to have survived various episodes of chemical and physical destruction, including metamorphism. Destruction could be related to percolating groundwaters or hydrothermal fluids or physical destruction due to mass wasting, erosion or impacts. While the smaller size of Mars and the lack of plate tectonics precludes the kinds of metamorphic alteration associated with rocks buried to similar depths on Earth, the numerous impacts that have marked its surface over the last >4 Ga will have left significant shock effects as well as regions of total destruction. Study of the ALH84001 meteorite shows that it was sourced from the coarse-grained, fractionated base of a consolidated melt (flow or sill) and was affected subsequently by a variety of processes on Mars. After consolidation of the flow, fractures and shock features in the rock indicate that the host formation was impacted. The rock was subjected to a high-temperature metamorphism, possibly related to the original impact (Treiman, Reference Treiman1995). Following this impact and metamorphism, groundwaters penetrated through the fractures, depositing secondary Fe-Mg-Mn carbonates along the walls of the fractures. A later shock event affected the whole rock and ejected a portion into space, which landed on the Antarctic ice cap about 13 000 years ago after >16My in space.
The processes of fossilization of microorganisms have been detailed previously in a number of publications (Westall et al., Reference Westall, Campbell, Bréhéret, Foucher, Gautret, Hubert, Sorieul, Grassineau and Guido2015a, Reference Westall, Hickman-Lewis, Hinman, Gautret, Campbell, Bréhéret, Foucher, Hubert, Sorieul, Dass, Kee, Georgelin and Brack2018; Westall and Hickman-Lewis, Reference Westall, Hickman-Lewis and Kolb2018). Briefly, microorganisms can be preserved as physical structures, i.e. as cells, colonies and biofilms, if they are rapidly coated and encased in a mineral cement (Fig. 3). This needs to occur during the life time of a microbe or colony otherwise its physical structure very rapidly degrades. Orange et al. (Reference Orange, Westall, Disnar, Prieur, Bienvenu, Le Romancer and Défarge2009) noted that the chelation of minerals to a cell surface occurs within 24 h after which purely chemical polymerization takes place creating a crust of various structures and thicknesses, depending on the cell wall structure (Westall, Reference Westall, Cosmovici, Bower and Wertheimer1997; Orange et al., Reference Orange, Westall, Disnar, Prieur, Bienvenu, Le Romancer and Défarge2009). The moulds of former cells can also be preserved in a mineral matrix (e.g. Liebig et al., Reference Liebig, Westall and Schmitz1996; Homann et al., Reference Homann, Heubeck, Airo and Tice2015). Physical microbial constructions, such as those produced by phototrophic microbial mats (e.g. stromatolites, MISS and other microbialites) are preserved by in situ mineralization of organic matter (EPS, cells and trichomes) and early cementation of host sediments (Fig. 4; e.g. Hofmann et al., Reference Hofmann, Grey, Hickman and Thorpe1999; Noffke et al., Reference Noffke, Beukes, Bower, Hazen and Swift2008). In reducing conditions, the degraded organic components of fossilized structures are encased by the surrounding mineral matrix. Oxidizing conditions, on the other hand, will destroy them. Diverse organo-geochemical signatures may be associated with either remnant organic matter in the structures or trapped within the embedding mineral matrix. For example, the silicified remains of organically preserved anaerobic phototrophic biofilms and microbial mats in Palaeoarchaean, Mars-analogue sediments from 3.3–3.5 billion-year-old fossiliferous horizons in the Barberton Greenstone Belt in South Africa, reveal organic biosignatures in the form of kerogen distributions (Westall et al., Reference Westall, de Ronde, Southam, Grassineau, Colas, Cockell and Lammer2006b, Reference Westall, Cavalazzi, Lemelle, Marrocchi, Rouzaud, Simionovici, Salomé, Mostefaoui, Andreazza, Foucher, Toporski, Jauss, Thiel, Southam, MacLean, Wirick, Hofmann, Meibom, Robert and Défarge2011), molecular structure (e.g. CH3/CH2 ratios) and δ13C ratios (Hickman-Lewis et al., Reference Hickman-Lewis, Cavalazzi, Sorieul, Gautret, Foucher, Whitehouse, Jeon, Georgelin, Cockell and Westall2020a, Reference Hickman-Lewis, Westall and Cavalazzi2020c), as well as enhanced transition metal concentrations indicative of microbial origin (Hickman-Lewis et al., Reference Hickman-Lewis, Cavalazzi, Sorieul, Gautret, Foucher, Whitehouse, Jeon, Georgelin, Cockell and Westall2020a). In general, the physical remains of cells, colonies and mats are rarely well-preserved; it is more common to find poorly preserved remains or simply generic organic matter coming from the degraded cells that are trapped in a mineral cement that remains (Walsh and Lowe, Reference Walsh and Lowe1999; van Zuilen et al., Reference van Zuilen, Chaussidon, Rollion-Bard and Marty2007).
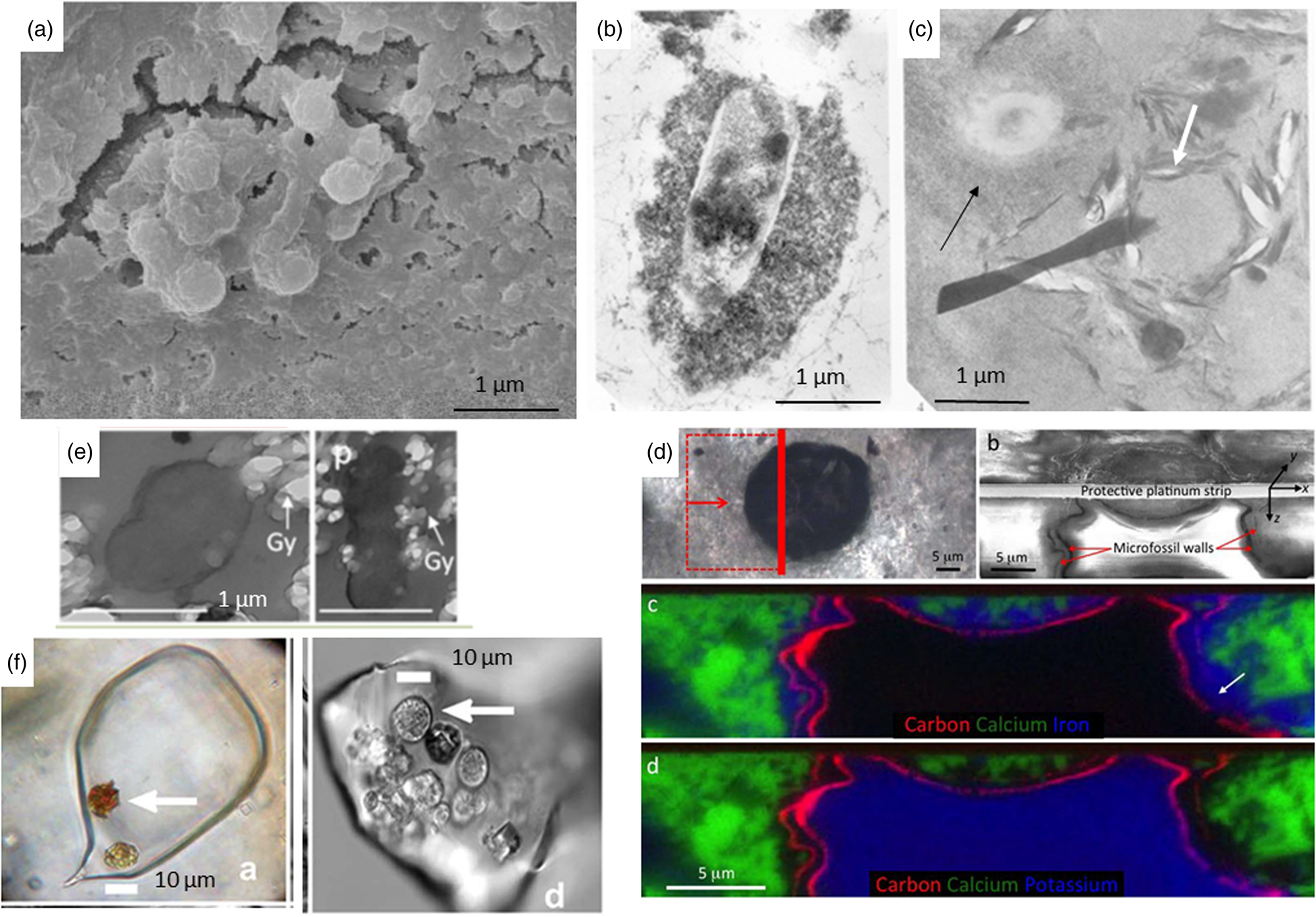
Fig. 3. (a) Calcified bacteria, SEM micrograph (Brunelli et al., Reference Brunelli, Carinci, Zollino, Candotto, Scarano and Lauritano2012; SAGE journals, permission). (b) Silicified bacteria, TEM micrograph of experimental fossilisation (Westall et al., Reference Westall, Boni and Guerzoni1995; The Palaeontological Association, permission). (c) Fossilized microorganism with a thick silica crust (black arrow) and the mould of a microorganism outlined by phyllosilicates (white arrow), TEM micrograph of experimental fossilisation (Westall et al., Reference Westall, Boni and Guerzoni1995; The Palaeontological Association, permission). (d) 1 Ga old eukaryote cell preserved by external Fe clays (c) and internal K clays (d) (Wacey et al., Reference Wacey, Saunders, Roberts, Menon, Green, Kong, Culwick, Strother and Brasier2014; Sci. Rep., permission). (e) Embedding of gypsum crystals on the outside and inside of an experimentally fossilized microorganism (Yersinia), TEM micrograph (Gaboyer et al., Reference Gaboyer, Le Milbeau, Bohmeier, Schwendner, Vannier, Beblo-Vranesevic, Rabbow, Foucher, Gautret and Guégan2017; Sci. Rep., permission). (f) Algal cells embedded in a modern halite crystal (left) and in a 150 Ka halite crystal (Sankaranarayanan et al., Reference Sankaranarayanan, Timofeeff, Spathis, Lowenstein and Lum2011; Open Commons, permission).

Fig. 4. (a) Cold seeps from the Arctic (Williscroft et al., Reference Williscroft, Grasby, Beauchamp, Little, Dewing, Birgel, Poulton and Hryniewicz2017; GSA, permission). (b) Moulds of rod-shaped microbes in microbially-precipitated carbonate from a limestone cave (Cacchio et al., Reference Cacchio, Ercole, Cappuccio and Lepidi2003; Taylor and Francis, permission).
Subtler indications of microbial presence, such as their effects on mineral growth, corrosion features, or even biominerals, are very difficult to identify without additional evidence in the rock record. Of relevance for Mars, evidence of microbial corrosion tunnels in basaltic pillow lava surfaces from the Palaeoarchaean of the Barberton Greenstone Belt has been hotly contested (Furnes et al., Reference Furnes, Banerjee, Muehlenbachs, Staudigel and de Wit2004; McLoughlin et al., Reference McLoughlin, Staudigel, Furnes, Eickmann and Ivarsson2010). Furnes et al. (Reference Furnes, Banerjee, Muehlenbachs, Staudigel and de Wit2004) compared microtextures comprising radiating filaments of microcrystalline titanite in the rims of ancient pillow basalts to similar features occurring during modern microbial corrosion of volcanic glasses (Furnes et al., Reference Furnes, Banerjee, Staudigel, Muehlenbachs, McLoughlin, de Wit and Van Kranendonk2007). Nevertheless, these particular tunnel-like features have also been interpreted as abiogenic (McLoughlin et al., Reference McLoughlin, Staudigel, Furnes, Eickmann and Ivarsson2010; Lepot et al., Reference Lepot, Benzerara and Philippot2011). For example, they have been reported to lack the organic lining that would be expected were they of microbial origin (McLoughlin et al., Reference McLoughlin, Grosch, Kilburn and Wacey2012). In contrast, Foucher et al. (Reference Foucher, Westall, Brandstätter, Demets, Parnell, Cockell, Edwards, Bény and Brack2010) document fossilized (silicified) polymeric substances infilling tunnels corroded into the surfaces of volcanic glass particles from the 3.45 Ga Kitty's Gap Chert in the Pilbara Greenstone Belt, Australia. In this case, the direct association of organic polymer within tunnels at the surface of the volcanic glass, plus associated fossilized cells and colonies at the surfaces of the volcanic clasts (Westall et al., Reference Westall, de Vries, Nijman, Rouchon, Orberger, Pearson, Watson, Verchovsky, Wright, Rouzaud, Marchesini, Severine, Reimold and Gibson2006a, Reference Westall, Cavalazzi, Lemelle, Marrocchi, Rouzaud, Simionovici, Salomé, Mostefaoui, Andreazza, Foucher, Toporski, Jauss, Thiel, Southam, MacLean, Wirick, Hofmann, Meibom, Robert and Défarge2011), all later entombed by a penecontemporaneous cement of silica, is a strong indication of corrosion due to microbial metabolic processes.
Importantly, especially when considering the ancient terrestrial rock record or the search for life on Mars, several types of biosignature needs to be present to increase the probability of a reliable interpretation. This multi-signature approach was attempted by McKay et al. (Reference McKay, Gibson, Thomas-Keprta, Vali, Romanek, Clemett, Chillier, Maechling and Zare1996); however, the resulting interpretations were incorrect because of the lack of understanding of biosignatures at that time.
Carbonates
(1) Carbonates in subsurface environments: The carbonates in ALH84001 formed in a subsurface environment, in this case in an igneous rock. The McKay team had identified globular features and lineations of such features in the carbonate deposits of ALH84001 that they interpreted as nanobacteria. Carbonates are a mineral phase whose formation may be mediated by microbial metabolisms (Riding, Reference Riding1991; Spadafora et al., Reference Spadafora, Perri, McKenzie and Vasconcelos2010), often preserving physical biosignatures, such as stromatolites as well as, occasionally, individual microbial cells (e.g. Fig. 3a; Perri et al., Reference Perri, Tucker and Spadafora2012). Biomineralization may be a passive process in which a mineral forms as a result of microbial metabolic processes affecting its immediate environment, for example, changing pH, or the production of metabolites that then combine with various ions in solution. It may also be actively induced, for instance, when bacteria control the Ca2+ content in their cells by using a cross-membrane proton pump to eliminate excess ions. The expelled Ca2+ then combines with CO3− in solution to precipitate around the cell walls (Bosak and Newman, Reference Bosak and Newman2003). In the subsurface, carbonate deposits can form naturally in certain carbonate cave systems (Fig. 4b; Cacchio et al., Reference Cacchio, Ercole, Cappuccio and Lepidi2003), as well as from drip water in mines (García et al., Reference García, Márquez and Moreno2016). These environments are aerobic to microaerophilic. Fossilization of the bacterium Acinetobacter gyllenbergii, isolated from Columbian mines, was demonstrated experimentally with the precipitation of carbonate around cell walls (García et al., Reference García, Márquez and Moreno2016). Subsurface carbonates also form in non-carbonate rock lithologies. For example, carbonates in fractures in granitic host rock formed as a result of microbial oxidation of methane (Drake et al., Reference Drake, Åström, Heim, Broman, Åström, Whitehouse, Ivarsson, Siljeström and Sjövall2015). The latter preserves the most extreme 13C depletion recorded to date (−125‰) as a result of the incorporation of C from biogenic methane in this energy-limited and C-poor environment. The carbonates also host lipid-derived organic components emanating from a microbial consortium comprising anaerobic methane-oxidizing archaea and sulphate-reducing bacteria (SRB). Note, however, that the biosignatures are confined to carbon-bearing mineral phases (clays) occurring at the boundaries of the calcite grains, not within the carbonate grains themselves.
In the case of the ALH84001 meteorite, it would in theory be possible for microorganisms to mediate carbonate precipitation, but it seems more likely that such processes are limited to heterotrophs. As these organisms obtain their energy and nutrients from the degradation of pre-existing carbon, one could hypothesize that such microorganisms were washed into fractures in lava flows at the surface of Mars. However, in a subsurface, oligotrophic environment without a primary carbon source, it is questionable as to whether the microorganisms would have been able to metabolize and produce a carbonate deposit. Nevertheless, similar to the precipitates in the ALH84001 meteorite, Benzerara et al. (Reference Benzerara, Menguy, Guyot, Dominici and Gillet2003) described nano-sized bacteriomorph crystallites of carbonate in a terrestrial weathering phase of pyroxenes in the Tatahouine meteorite, in this case associated with terrestrial microbes. They concluded that, while the formation of the crystallites was microbially-induced, the structures in themselves were chemical precipitates, i.e. abiotic growth, not fossilized microbes.
(2) Surface carbonate deposits: The majority of known microbially-mediated carbonate formation occurs in subaerial environments, in particular in relationship with phototrophic microbial mats resulting in tabular or three-dimensional stromatolitic structures (Warthmann et al., Reference Warthmann, van Lith, Vasconcelos, McKenzie and Karpoff2000; Dupraz et al., Reference Dupraz, Reid, Braissant, Decho, Norman and Visscher2009; Bosak et al., Reference Bosak, Knoll and Petroff2013). While it is unclear whether Martian life could have reached the evolutionary state of phototrophy owing to the phenomenon of punctuated habitability (Westall et al., Reference Westall, Campbell, Bréhéret, Foucher, Gautret, Hubert, Sorieul, Grassineau and Guido2015a), we cannot completely discount this possibility. Therefore, a biosignature of particular relevance for anaerobic early Mars is the microbial biomineralization of carbonate mediated by consortia of anaerobic phototrophs and their accompanying heterotrophic degraders, such as SRB. In this case, SRB activity creates the chemical conditions for the precipitation of carbonate by increasing alkalinity in the environment such that Ca2+ ions released from microbial extracellular polymeric substances (EPS), an important component of phototrophic biofilms and mats, react with the CO2− dissolved in the aqueous phase to form CaCO3. SRBs in such consortia precipitating nanocrystals of aragonite and CaSO4 within degraded organic matter were documented from Mars-analogue, shallow water volcanoclastic sediments dated to 3.33 Ga from the Barberton Greenstone Belt (Westall et al., Reference Westall, de Vries, Nijman, Rouchon, Orberger, Pearson, Watson, Verchovsky, Wright, Rouzaud, Marchesini, Severine, Reimold and Gibson2006a, Reference Westall, Cavalazzi, Lemelle, Marrocchi, Rouzaud, Simionovici, Salomé, Mostefaoui, Andreazza, Foucher, Toporski, Jauss, Thiel, Southam, MacLean, Wirick, Hofmann, Meibom, Robert and Défarge2011).
Substantial carbonate precipitations in the form of mounds occur in association with cold methane seeps, as well as white smoker vents (Fig. 4a). Methane seeps are widespread and are found in the terrestrial rock record throughout the Phanerozoic (Barbieri and Cavalazzi, Reference Barbieri, Cavalazzi, Seckbach and Walsh2008). They host anaerobic chemosynthetic communities that oxidize methane and sulphide to obtain energy, using dissolved CO2 as their carbon source. An increase of pH value driven by their metabolisms leads to the precipitation of calcium carbonate, as in the phototrophic mat communities described above. Although carbonate is formed by these processes, it is rare for individual cells to be preserved although fossil microbial filaments and mats have been documented within cold seep carbonate deposits of Devonian age from Morocco (Cavalazzi, Reference Cavalazzi2007; Cavalazzi et al., Reference Cavalazzi, Barbieri and Ori2007).
(3) Carbonates on Mars: Spectral analysis from orbit of the surface of Mars has detected carbonates associated with aqueous weathering profiles across the planet's Noachian terranes (pre 3.7 Ga), suggesting a warmer, wetter context for their formation (Bultel et al., Reference Bultel, Viennet, Poulet, Carter and Werner2019). These detections are associated with surficial weathering profiles, i.e. environments in which nutrients may have been lost by leaching, thereby reducing habitability (Duddy, Reference Duddy1980; Nesbitt et al., Reference Nesbitt, Markovics and Price1980), although such environments on the modern, oxygenated and highly habitable Earth host a diverse array of life. At the ExoMars 2022 Oxia Planum landing site, there is a spectral suggestion of carbonate associated with phyllosilicates (Mandon et al., Reference Mandon, Parkes Bowen, Quantin-Nataf, Bridges, Carter, Pan, Beck, Dehouck, Volat, Thomas, Cremonese, Tornabene and Thollot2021) although the exact environment of deposition in this case has yet to be elucidated, whether weathering, lacustrine or marine (Quantin-Nataf et al., Reference Quantin-Nataf, Carter, Mandon, Thollot, Balme, Volat, Pan, Loizeau, Millot, Breton, Dehouck, Fawdon, Gupta, Davis, Grindrod, Pacifici, Bultel, Allemand, Ody, Lozach and Broyer2021). On the other hand, Jezero crater, the landing site of the Mars 2020 Perseverance rover, displays carbonates in association with a variety of interpreted paleoenvironments, including weathering profiles of volcanic ash, as well as marginal lakeshore and/or fluvial precipitations of carbonate that are compared to carbonate reefs, microbialites or tufa, having the potential to preserve the kinds of biosignatures described above (Horgan et al., Reference Horgan, Anderson, Dromart, Amador and Rice2020). Similarly, Franz et al. (Reference Franz, Mahaffy, Webster, Flesch, Raaen, Freissinet, Atreya, House, McAdam, Knudson, Archer, Stern, Steele, Sutter, Eigenbrode, Glavin, Lewis, Malespin, Millan, Ming, Navarro-González and Summons2020) evoke carbonate in the endogenous carbon cycle of Gale crater (Fig. 5). The main problem with carbonates is their propensity to corrode under the acidic weathering conditions proposed for early Mars, which had a thicker CO2 atmosphere than today (Viennet et al., Reference Viennet, Bultel and Werner2019). Such conditions would lead to the destruction or significant alteration of potential biosignatures.
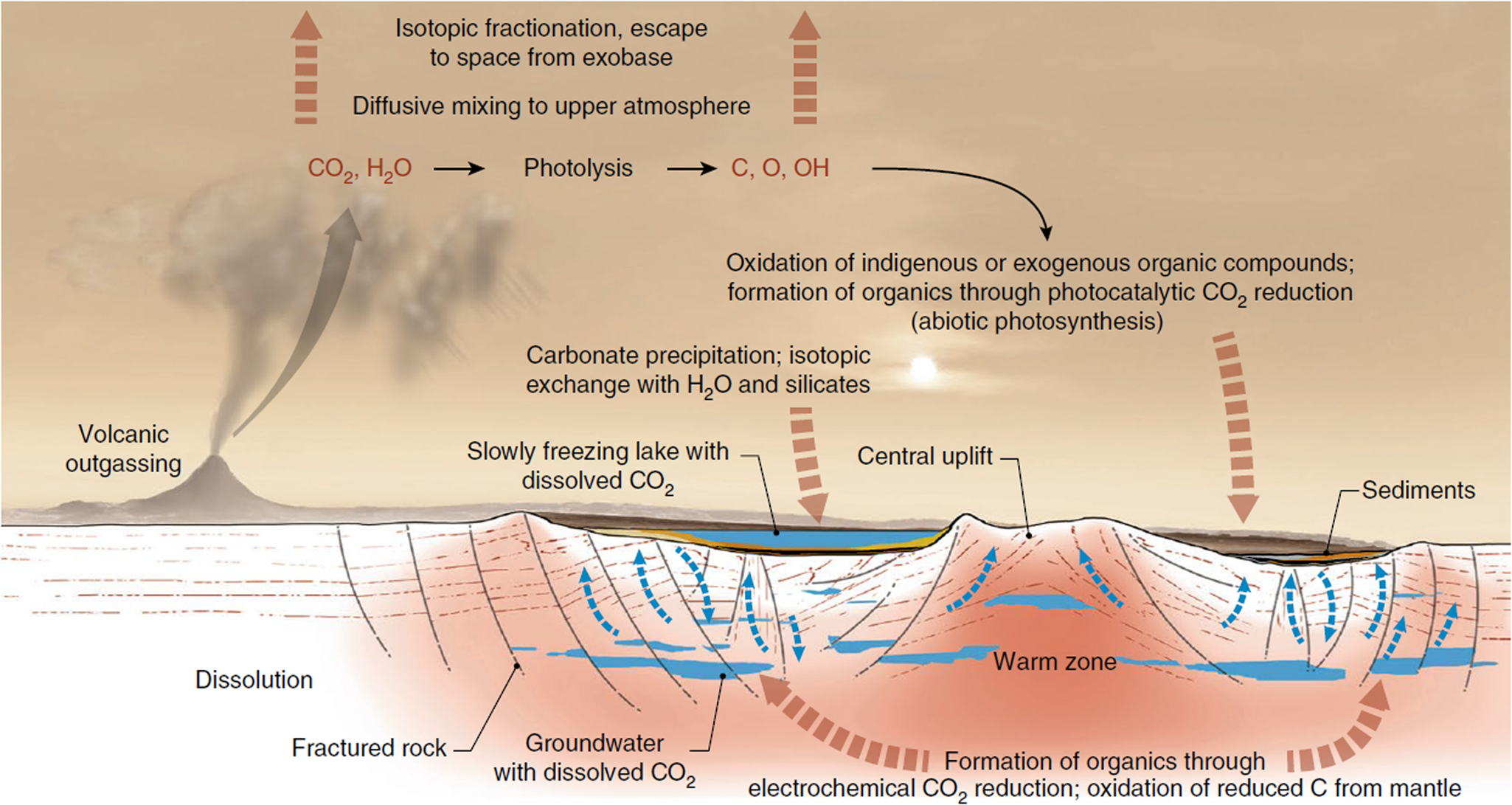
Fig. 5. Possible carbon (and carbonate) cycle at Gale crater (Franz et al., Reference Franz, Mahaffy, Webster, Flesch, Raaen, Freissinet, Atreya, House, McAdam, Knudson, Archer, Stern, Steele, Sutter, Eigenbrode, Glavin, Lewis, Malespin, Millan, Ming, Navarro-González and Summons2020; Nature, permission).
Other mineral associations with biosignatures: While not associated with the ALH84001 meteorite, other minerals that are present on Mars, including silica, phyllosilicates and evaporitic phases such as sulphates, could also preserve biosignatures. In addition, the preservation of MISS in clastic sediments should be noted, for example, in the Palaeo-Mid Archaean formations of the Barberton Greenstone Belt in South Africa and the Pilbara Greenstone Belt in Australia (Noffke, Reference Noffke2010; Noffke et al., Reference Noffke, Christian, Wacey and Hazen2013).
Silica
(1) Siliceous hydrothermal environments, both subaqueous and subaerial, are prime sites for microbial development because of the significant supply of nutrients (Walter and Des Marais, Reference Walter and Des Marais1993; Cady and Farmer, Reference Cady and Farmer1996). With their high concentrations of nutrients (either organic, such as small molecules, gases such as CH4, H2), essential transition elements (Fe, Ni, V, Co, As), and variable gradients in pH, Eh and temperature, they provide a habitat for a wide variety of microbial life (Baross, Reference Baross1998). On present-day Earth, siliceous hydrothermal environments are essentially, but not only, associated with deep sea black smokers. Upon initial formation, these >300 °C environments are sterile but later become colonized by hyperthermophilic archaea transported by aqueous currents to the edifices (Wirth et al., Reference Wirth, Luckner and Wanner2018).
We will here consider hydrothermal environments on the early Earth because of their particular relevance for early Mars, the environments of the two planets being similar (Westall et al., Reference Westall, Foucher, Bost, Bertrand, Loizeau, Vago, Kminek, Gaboyer, Campbell, Bréhéret, Gautret and Cockell2015b). Marine hydrothermal environments on the early Earth occurred at all water depths including in the shallow water basins on the submerged oceanic plateaux that characterized the early protocontinents. Indeed, they also occur in the littoral and adjacent subaerial environments (Westall et al., Reference Westall, Campbell, Bréhéret, Foucher, Gautret, Hubert, Sorieul, Grassineau and Guido2015a, Reference Westall, Foucher, Bost, Bertrand, Loizeau, Vago, Kminek, Gaboyer, Campbell, Bréhéret, Gautret and Cockell2015b; Djokic et al., Reference Djokic, Van Kranendonk, Campbell, Walter and Ward2017). Higher heat flow from the early Earth's mantle (Sleep, Reference Sleep2000; Zahnle et al., Reference Zahnle, Ardnt, Cockell, Halliday, Nisbet, Selsis and Sleep2007; Kamber, Reference Kamber2015; Labrosse, Reference Labrosse2015; Van Kranendonk et al., Reference Van Kranendonk, Smithies, Griffin, Huston, Hickman, Champion, Anhaeusser and Pirajno2015) fuelled abundant volcanic and hydrothermal activity. Rare earth element (REE) signatures including Eu and Y anomalies, Y/Ho ratio, as well as the physical evidence of hydrothermal veins and pervasive silicification of all lithologies in contact with seawater, attest to the pervasive influence of hydrothermal fluids in the global ocean (e.g. Van Kranendonk, Reference Van Kranendonk2006; Hofmann and Bolhar, Reference Hofmann and Bolhar2007; Hofmann and Harris, Reference Hofmann and Harris2008; Westall et al., Reference Westall, Foucher, Bost, Bertrand, Loizeau, Vago, Kminek, Gaboyer, Campbell, Bréhéret, Gautret and Cockell2015b, Reference Westall, Hickman-Lewis, Hinman, Gautret, Campbell, Bréhéret, Foucher, Hubert, Sorieul, Dass, Kee, Georgelin and Brack2018), as well as in restricted shallow basins on top of the oceanic plateaux where influences from non-marine waters have been additionally detected (Hickman-Lewis et al., Reference Hickman-Lewis, Gourcerol, Westall, Manzini and Cavalazzi2020b).
Most physical evidence for hydrothermal activity on the early Earth points to subaqueous systems. Due to pervasive silicification (>96% SiO2) of Palaeoarchaean sediments and the immediately underlying volcanics owing to oversaturation of silica in the early oceans, any physical expression of vents is difficult to detect, although some hydrothermal veins are readily identifiable by virtue of their cross-cutting nature (Hofmann and Bolhar, Reference Hofmann and Bolhar2007; Westall et al., Reference Westall, Campbell, Bréhéret, Foucher, Gautret, Hubert, Sorieul, Grassineau and Guido2015a). Veins can also form bedding-parallel intrusions into partially lithified sediment (Westall et al., Reference Westall, Campbell, Bréhéret, Foucher, Gautret, Hubert, Sorieul, Grassineau and Guido2015a). The 3.33 Ga Josefsdal Chert from the Barberton Greenstone Belt in South Africa comprises both volcanic sands and chemically precipitated sedimentary silica gel, now chert. Adjacent to hydrothermal vents, the volcanic sands are heavily encrusted by carbonaceous matter (CM), while silica gel mixed with very fine volcanic dust is characterized by stellate clots of CM identified as probable chemotrophic biomass (Westall et al., Reference Westall, Campbell, Bréhéret, Foucher, Gautret, Hubert, Sorieul, Grassineau and Guido2015a, Reference Westall, Foucher, Bost, Bertrand, Loizeau, Vago, Kminek, Gaboyer, Campbell, Bréhéret, Gautret and Cockell2015b; Hickman-Lewis et al., Reference Hickman-Lewis, Cavalazzi, Sorieul, Gautret, Foucher, Whitehouse, Jeon, Georgelin, Cockell and Westall2020a). The 3D, irregular, stellate formations of CM around the volcanic clasts (up to 30 μm thickness) and in irregular clots within the silica gel (up to 500 μm diameter) are categorically incompatible with abiotic CM aggregation, deposition or diffusion. In addition, the CM has enhanced concentrations of transition metals (Fe, V, Ni, As and Co), and a δ13C signature dominantly between −21.0 and −11.5‰ and as light as −41.3‰. These elemental and isotopic signatures, together with the morphological distribution of the CM and its petrographic context, suggest that this kerogen represents the remnants of lithotrophic or organotrophic consortia, possibly cycling methane or nitrogen (Hickman-Lewis et al., Reference Hickman-Lewis, Cavalazzi, Sorieul, Gautret, Foucher, Whitehouse, Jeon, Georgelin, Cockell and Westall2020a). When observed in field exposures, however, the heavy silicification makes direct detection of the clotted colonies in these sediments difficult, although sometimes a faint mottled texture reveals the presence of the carbonaceous clots.
It is rare for individual cells to be preserved morphologically in these ancient sediments, rapid silicification prior to heterotrophic degradation being a prerequisite (e.g. Fig. 3b). The rarely preserved examples show that individual cells are generally too small (<1 μm) to be analysed individually by in situ methods on Mars, although, as we have seen above, it may be possible to identify the carbonaceous remains of colonies of several 100 s μm size.
Not all Palaeoarchaean hydrothermal environments are subaqueous. Recently, subaerial sinter springs, similar to those on the modern Earth, have been described from the 3.48 Ga Dresser Formation, Pilbara, Australia. Djokic et al. (Reference Djokic, Van Kranendonk, Campbell, Walter and Ward2017, Reference Djokic, Van Kranendonk, Campbell, Havig, Walter and Guido2021) used textures and REE and yttrium geochemistry to demonstrate similarities between the Dresser geyserites and Phanerozoic hot spring sinters, noting the temporal association of a diverse suite of textural biosignatures that indicates a thriving microbial community. The biosignatures are primarily physical in nature, including stromatolites and microbial palisade fabric preserved in inferred mineralized exopolymeric substance. It is suggested that such geyserites and their textural features could be an explanation for the silica deposits identified around Home Plate in Gusev Crater (Ruff and Farmer, Reference Ruff and Farmer2016; Djokic et al., Reference Djokic, Van Kranendonk, Campbell, Havig, Walter and Guido2021).
Silica as a chemical sediment was ubiquitous on the early Earth both because of generally high silica concentrations in the sea water (Siever, Reference Siever1992) due to high rates of weathering of exposed landmasses and lack of removal by organisms using silica as a framework for their cells (e.g. diatoms that evolved later), and because of elevated hydrothermal influence. Another significant silica contribution comes from the devitrification of subaqueous volcanic sediments. This high concentration of silica in the early oceans was the prime reason for the silicification of early sediments and volcanic rocks exposed to seawater. It is also the reason for the rapid preservation of microbial biosignatures, even in non-hydrothermal, oligotrophic environments, as described by Westall et al. (Reference Westall, Cavalazzi, Lemelle, Marrocchi, Rouzaud, Simionovici, Salomé, Mostefaoui, Andreazza, Foucher, Toporski, Jauss, Thiel, Southam, MacLean, Wirick, Hofmann, Meibom, Robert and Défarge2011, Reference Westall, Campbell, Bréhéret, Foucher, Gautret, Hubert, Sorieul, Grassineau and Guido2015a).
Silica can also be concentrated in evaporitic settings, contributing to the entombment of microorganisms inhabiting the environment. Examples include the ~800 Ma Draken Formation in Svalbard where enhanced silica concentrations in an evaporitic environment resulted in the silicification of cyanobacterial mats (Foucher and Westall, Reference Foucher and Westall2013). While we do not expect to find cyanobacterial ecosystems on Mars, this example demonstrates the high fidelity of preservation by evaporitic silica and, in addition, documents a different type of biosignature, metastable minerals. In this example, metastable opal occurs within the organically preserved cell envelopes of the cyanobacteria while the enclosing silica cement is recrystallized to quartz, the organic phase enhancing the stability of the metastable mineral. It is further noteworthy that a beautifully preserved sequence of delicate microbial biofilms in the 3.33 Ga Josefsdal Chert formed in an evaporitic environment as attested to by the pseudomorph evaporite minerals embedded in their surfaces (Westall et al., Reference Westall, Cavalazzi, Lemelle, Marrocchi, Rouzaud, Simionovici, Salomé, Mostefaoui, Andreazza, Foucher, Toporski, Jauss, Thiel, Southam, MacLean, Wirick, Hofmann, Meibom, Robert and Défarge2011). NanoSIMS scans across the thicknesses of the biofilms document that they had been thoroughly silicified.
Continuing with the theme of weathering profiles on Mars evoked above, Rutledge et al. (Reference Rutledge, Horgan, Havig, Rampe, Scudder and Hamilton2018) documented high rates of glacial alteration of basaltic volcanic materials and the subsequent precipitation of amorphous (opaline) silica on rock surfaces in subglacial conditions on Iceland, analogous to those on early Mars; however, no biosignatures occur in these phases. Interestingly, a significant amount of poorly crystalline silica phases is mixed in with the detrital products of glacial weathering of the volcanic rock. X-ray amorphous silica is an important constituent of the sediments in Gale Crater where it can constitute up to 90% of the rock (Rapin et al., Reference Rapin, Chauviré, Gabriel, McAdam, Ehlmann, Hardgrove, Meslin, Rondeau, Dehouck, Franz, Mangold, Chipera, Wiens, Frydenvang and Schröder2018).
(2) Silica on Mars: Hydrated silica deposits have been detected from orbit in many locations at the surface of Mars. Sun and Milliken (Reference Sun and Milliken2018) determined that the mineral phases detected fall into two categories: amorphous and/or dehydrated silica-bearing bedrock deposits, and more crystalline and/or hydrated silica-bearing aeolian deposits. The latter provide evidence of impact-related, hydrothermally produced opaline silica and the former altered volcanic phases or dehydrated silica (Pineau et al., Reference Pineau, Le Deit, Chauviré, Carter, Rondeau and Mangold2020).
The most significant deposit of silica on Mars found to date is that around Home Plate, where amorphous SiO2.nH2O occurs in what may be an ancient volcanic hydrothermal setting in Gusev crater (Fig. 6(a) and (b); Squyres et al., Reference Squyres, Arvidson, Ruff, Gellert, Morris, Ming, Crumpler, Farmer, Marais, Yen, McLennan, Calvin, Bell, Clark, Wang, McCoy, Schmidt and de Souza2008; Ruff et al., Reference Ruff, Farmer, Calvin, Herkenhoff, Johnson, Morris, Rice, Arvidson, Bell, Christensen and Squyres2011). This deposit has been attributed to either fumarole-related, acid-sulphate leaching or precipitation from hot spring fluids with the latter being a privileged hypothesis based on analogue textural observations (Ruff and Farmer, Reference Ruff and Farmer2016) from hot springs at El Tatio in Chile. These authors suggest that the nodular and digitate silica structures at El Tatio, produced by a combination of biotic and abiotic processes, are very similar to those observed at Home Plate. Djokic et al. (Reference Djokic, Van Kranendonk, Campbell, Havig, Walter and Guido2021) also suggest similarities between textural biosignatures in Palaeoarchaean geyserites and those of Home Plate.
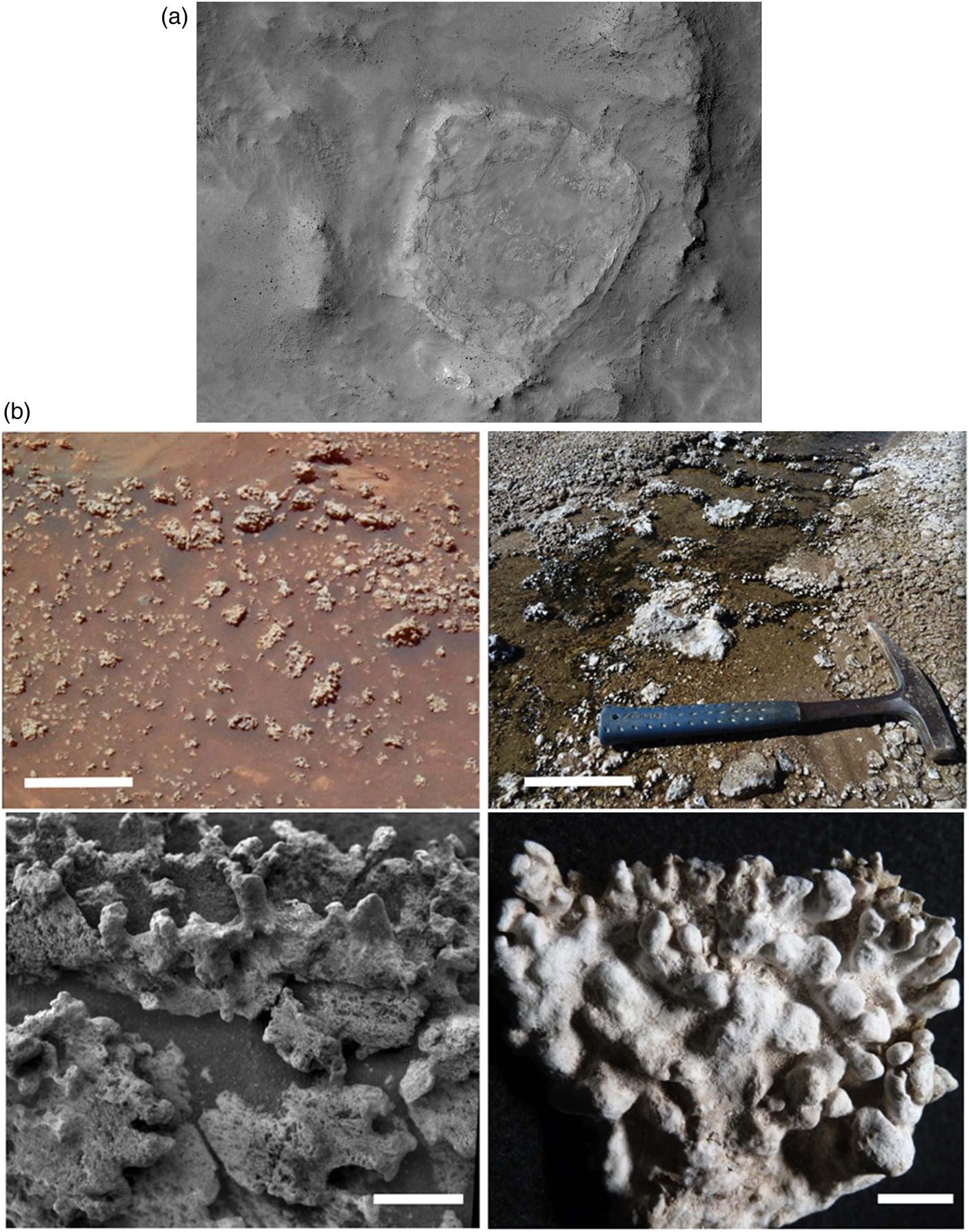
Fig. 6. (a) High-resolution image of Home Plate from orbit (Tao et al., Reference Tao, Conway, Muller, Putri, Thomas and Cremonese2021). (b) Comparison of the Home Plate silica deposits in Gusev Crater, Mars (left), with the El Tatio hot spring deposits in the Atacama (right); field views above, details of the silica spicules below (Ruff and Farmer, Reference Ruff and Farmer2016).
Hydrated (opaline) silica deposits detected in Jezero crater are of interest because they are a good mineral phase for biosignature preservation (Tarnas et al., Reference Tarnas, Mustard, Lin, Goudge, Amador, Bramble, Kremer, Zhang, Itoh and Parente2019). Associated with specific rock units, such as the opal-magnesite-smectite series detected in the olivine-rich unit and the opal-Al-phyllosilicate phase series co-located with the basement unit, the formation processes of the hydrated silica deposits are not yet understood. Possible candidates are: primary volcanism, diagenesis, authigenic formation in a lacustrine environment, detrital transport of material formed authigenically in the Jezero watershed, or transport to Jezero crater via aeolian processes.
Hydrated silica has been detected from orbit at the edges of deltaic fan deposits formed in relationship with a standing body of water in Oxia Planum, the chosen landing site for the ExoMars 2022 mission (Quantin-Nataf et al., Reference Quantin-Nataf, Carter, Mandon, Thollot, Balme, Volat, Pan, Loizeau, Millot, Breton, Dehouck, Fawdon, Gupta, Davis, Grindrod, Pacifici, Bultel, Allemand, Ody, Lozach and Broyer2021). Here, the hydrated silica deposits overlie clay-rich facies and indicate a changing hydrological regime possibly related to marine regression, or at least the drying of a water-filled basin, concurrent with the global hydrological trajectory of Mars through the Noachian and Hesperian periods.
Phyllosilicates
Direct morphological evidence of life will be difficult to detect on Mars because of (1) the very small size of the individual microorganisms, (2) the difficulty of distinguishing eventual microbial ‘clotted’ textures from abiotic ones, and (3) the possibility that distinctive macroscopic features, such as complex stromatolites, may not occur on Mars. Phyllosilicate minerals, however, have a propensity to accumulate and preserve organic matter, which chelates onto and in between the silicate sheets. As such, they are favoured mineral phases for the conservation of potential organic biosignatures (Bishop et al., Reference Bishop, Loizeau, McKeown, Saper, Dyar, Des Marais, Parente and Murchie2013; Vago et al., Reference Vago, Westall, Coates, Jaumann, Korablev, Ciarletti, Mitrofanov, Josset, De Sanctis, Bibring, Rull, Goesmann, Steininger, Goetz, Brinckerhoff, Szopa, Raulin, Westall, Edwards, Whyte, Fairén, Bibring, Bridges, Hauber, Ori, Werner, Loizeau, Kuzmin, Williams, Flahaut, Forget, Vago, Rodionov, Korablev, Svedhem, Sefton-Nash, Kminek, Lorenzoni, Joudrier, Mikhailov, Zashchirinskiy, Alexashkin, Calantropio, Merlo, Poulakis, Witasse, Bayle, Bayón, Meierhenrich, Carter, García-Ruiz, Baglioni, Haldemann, Ball, Debus, Lindner, Haessig, Monteiro, Trautner, Voland, Rebeyre, Goulty, Didot, Durrant, Zekri, Koschny, Toni, Visentin, Zwick, van Winnendael, Azkarate and Carreau2017), although environmental parameters on Mars, such as UV radiation and acid leaching, can negatively influence the long-term preservation of organic molecules, even associated with phyllosilicates (Gil-Lozano et al., Reference Gil-Lozano, Fairén, Muñoz-Iglesias, Fernández-Sampedro, Prieto-Ballesteros, Gago-Duport, Losa-Adams, Carrizo, Bishop, Fornaro and Mateo-Martí2020).
The hypothesis is that phyllosilicates on Mars may have chelated the organic remnants of microbial life. These minerals can also physically fossilize microorganisms and their EPS. Wacey et al. (Reference Wacey, Saunders, Roberts, Menon, Green, Kong, Culwick, Strother and Brasier2014) noted the role of authigenic clay precipitation in organism preservation based on experimental investigations and studies of phenomena in the natural environment, ranging from marine settings, lakes, rivers and hydrothermal systems. A variety of clay mineral types, including Fe-rich phyllosilicates (e.g. nontronite), as well as Al clays (kaolinite and halloysite), and Mg or K-rich clays, are implicated in this process (Fig. 3(d)). The composition of the authigenic minerals that precipitate under the influence of microbial mediation depends on factors, such as pH, Eh, sulphate concentration, ionic availability and the nature of the biological substrate, which will affect its affinity for specific ions.
Authigenic precipitation or chelation of phyllosilicates may occur on the surfaces of individual organisms in multispecies communities. Under specific physico-chemical conditions, this leads to the replacement of whole microbial mats by phyllosilicates. Phyllosilicates chelating to the surfaces of microorganisms form a ‘halo’ around them. Such phenomena have been observed in Oligocene (~30 Ma) deep sea sediments (Monty et al., Reference Monty, Westall and van der Gaast1991; Westall and Rincé, Reference Westall and Rincé1994), as well as in artificial fossilization of microbes in their deep sea sedimentary matrices (Fig. 3(c); Westall et al., Reference Westall, Boni and Guerzoni1995). In all cases, there is an intimate intergrowth of phyllosilicates with microbial polymer (EPS) such that fibril-like strands of polymer stretch between packets of phyllosilicates (Westall et al., Reference Westall, Foucher, Bost, Bertrand, Loizeau, Vago, Kminek, Gaboyer, Campbell, Bréhéret, Gautret and Cockell2015b).
The importance of phyllosilicates in the preservation of soft-bodied organisms was highlighted in ~500 My Burgess Shale-type deposits hosting soft-bodied fauna, where kaolinite played an important role in the preservation process (Gaines et al., Reference Gaines, Briggs and Yuanlong2008; LaFlamme et al., Reference Laflamme, Schiffbauer, Narbonne and Briggs2011). Experimental studies showed how this process worked. Working with cyanobacteria, well known for their formation of significant mats that covered the Ediacaran seafloors, thus entombing the soft-bodied macrobiota, Newman et al. (Reference Newman, Mariotti, Pruss and Bosak2016) exposed the microorganisms to elevated concentrations of clay minerals and silica, showing that clays coated the sheaths of the microorganisms through two mechanisms: trapping and indirect precipitation. Extending the importance of phyllosilicate precipitation to the cell walls of microorganisms, Wacey et al. (Reference Wacey, Saunders, Roberts, Menon, Green, Kong, Culwick, Strother and Brasier2014) studied 1 Ga lake sediments of the Torridon Group, Scotland, and described a microbially-mediated Fe-rich phyllosilicate phase that occurs in direct contact with cellular material; the Fe-rich phyllosilicates replace the most labile biological material, while a K-rich clay occurs within and exterior to cell envelopes, forming where the supply of Fe was exhausted (Fig. 3(d)).
Phyllosilicates on Mars: Orbital spectral measurements of the surface of Mars have identified large areas covered by diverse phyllosilicate phases. Their distribution and associations with other mineral phases suggest major compositional changes through time related to changing global environmental conditions on Mars (e.g. Poulet et al., Reference Poulet, Bibring, Mustard, Gendrin, Mangold, Langevin, Arvidson, Gondet, Gomez, Berthé, Bibring, Langevin, Erard, Forni, Gendrin, Gondet, Manaud, Poulet, Poulleau, Soufflot, Combes, Drossart, Encrenaz, Fouchet, Melchiorri, Bellucci, Altieri, Formisano, Fonti, Capaccioni, Cerroni, Coradini, Korablev, Kottsov, Ignatiev, Titov, Zasova, Mangold, Pinet, Schmitt, Sotin, Hauber, Hoffmann, Jaumann, Keller, Arvidson, Mustard and Forget2005; Bishop et al., Reference Bishop, Fairén, Michalski, Gago-Duport, Baker, Velbel, Gross and Rampe2018). There is much debate as to the formation mechanisms and environmental constraints for the clays observed at Mars’ surface. Their relative abundance in the Noachian suggests that, in its early history, Mars had a long-lived warmer and wetter climate, although this scenario is at odds with climate models, which suggest that Noachian Mars was cold. Nevertheless, geomorphological features unambiguously indicate water activity at the surface of the planet. Noting that the time necessary for clay minerals to form in cold environments is extremely long, and taking into account climate models, geomorphological signatures and mineral phases, Bishop et al. (Reference Bishop, Fairén, Michalski, Gago-Duport, Baker, Velbel, Gross and Rampe2018) hypothesized phyllosilicate formation during sporadic, short-term warm and wet periods on a generally cold early Mars. They concluded that associations of Mg-rich clay-bearing rocks with mixed Fe/Mg smectite, chlorite, talc, serpentine and zeolite likely formed in subsurface hydrothermal environments. On the other hand, other assemblages exhibiting vertical zonation of Al/Fe3+-rich and Fe/Mg smectites, as well as sulphates are interpreted to have formed in different physico-chemical environments with different rock/water ratios on the surface of Mars. Another possibility is that the phyllosilicates could have formed preferentially in the water region in contact with a ‘hot’ ground – that is, heated from the interior, but not necessarily in the subsurface. In this scenario, most of Mars would have been cold (consistent with the then reduced insolation and with climate models), but with topical warm environments, where water flow and alteration could proceed mediated by internal heat. So, for example, some of the ancient hydrous features we observe today could have flowed under a top-frozen layer of water.
Clays at Gale crater: Gale crater is a ~150 km diameter impact crater of Mars located at the limit of the cratered highlands of the south and the low plains of the north. Its age is about 3.6–3.8 Ga (Noachian-Hesperian boundary) according to the crater counts (Thomson et al., Reference Thomson, Bridges, Milliken, Baldridge, Hook, Crowley, Marion, de Souza Filho, Brown and Weitz2011). Orbital observations by the visible-infrared spectrometer, Compact Reconnaissance Imaging Spectrometer for Mars (CRISM) and the spectro-imager, Thermal Emission Imaging System (THEMIS) characterized the mineral phases in the crater, while the Mars Science Laboratory mission's rover Curiosity has been assessing past habitability in the region. Mount Sharp (formally named Aeolis Mons), the large central mound inside the crater, has relatively flat strata that may have preserved evidence of the evolution of the Mars environment (Grotzinger et al., Reference Grotzinger, Crisp, Vasavada, Anderson, Baker, Barry, Blake, Conrad, Edgett, Ferdowski, Gellert, Gilbert, Golombek, Gómez-Elvira, Hassler, Jandura, Litvak, Mahaffy, Maki, Meyer, Malin, Mitrofanov, Simmonds, Vaniman, Welch and Wiens2012). The older, lower part of the mound contains clays minerals mixed with hydrated sulphates, indicating aqueous activity, while the younger layers higher on the mound are covered by a dust mantle (Milliken et al., Reference Milliken, Grotzinger and Thomson2010; Thomson et al., Reference Thomson, Bridges, Milliken, Baldridge, Hook, Crowley, Marion, de Souza Filho, Brown and Weitz2011; Wray, Reference Wray2013).
Fluvial valley walls and crater rims and floors contain olivine-bearing bedrock associated with Fe/Mg phyllosilicates, kaolin family minerals, hydrated silica and other hydrated/hydroxylated phases (Wray, Reference Wray2013; Carter et al., Reference Carter, Viviano-Beck, Loizeau, Bishop and Le Deit2015; Ehlmann and Buz, Reference Ehlmann and Buz2015). CRISM spectra of the walls and rims show absorption bands that can be associated to Fe/Mg phyllosilicates, such as nontronite and saponite, while the Mount Sharp spectra exhibit different spectral properties characteristic of Al phyllosilicates, including montmorillonite and kaolinite (Buz et al., Reference Buz, Ehlmann, Pan and Grotzinger2017). The detection of different types of phyllosilicates thus suggests protoliths of a different nature or the formation of clays by fluids of different composition. There are several suggested scenarios to explain the origin of the clays on the crater floor: either the clays were transported to the crater floor from the already partially altered crater rim; or they were formed in situ by alteration of the mafic bedrock by water and subsequently altered as a result of burial diagenesis (Buz et al., Reference Buz, Ehlmann, Pan and Grotzinger2017).
Clay minerals at Jezero Crater: The 3.7 Ga old Jezero Crater at the edge of Isidis Planitia hosts a well-formed delta and deposits indicating the presence of lakes in the past. At the landing site of the Mars 2020 mission and the Perseverance rover, there is a diversity of phyllosilicate-bearing units that may have high biosignature preservation potential, including Al-clays and potential Fe-Mg-bearing smectites (Goudge et al., Reference Goudge, Mustard, Head, Fassett and Wiseman2015; Brown et al., Reference Brown, Viviano and Goudge2020; Horgan et al., Reference Horgan, Anderson, Dromart, Amador and Rice2020). These units are predominantly associated with the western delta, although zones of phyllosilicate-rich mineralogy have also been identified dispersed throughout the crater (Horgan et al., Reference Horgan, Anderson, Dromart, Amador and Rice2020), including the region close to the rover landing site. Additionally, localized zones of phyllosilicate richness have been noted within the marginal olivine carbonate unit (Goudge et al., Reference Goudge, Mustard, Head, Fassett and Wiseman2015; Horgan et al., Reference Horgan, Anderson, Dromart, Amador and Rice2020). Systematic variations in CRISM spectral parameters have been used to infer variability in Fe/Mg-phyllosilicates and Mg-carbonates between the different (stratigraphic) units of Jezero crater; however, these interpretations await validation by surface observations and analyses. The regional-scale origin of phyllosilicate-rich phases and their relationships with the surrounding crater floor and margin units have been addressed in detail by Brown et al. (Reference Brown, Viviano and Goudge2020); these scenarios imply a complex geological history at Jezero crater with aqueous periods that may have been conducive to habitability throughout the Noachian and earliest Hesperian. Of particular interest for habitability and palaeoenvironment is the unresolved origin of Fe/Mg-phyllosilicates, which may have formed during near-surface weathering and diagenesis, through post-impact hydrothermal activity, or both (Ehlmann et al., Reference Ehlmann, Bell, Brown, Horgan, Hurowitz, Kelemen, Mangold, Mayhew, Quantin, Rapin, Razzell Hollis, Scheller, Shuster, Stack, Sun, Tarnas and Treiman2021).
Clay minerals at Oxia Planum: Oxia Planum is a ~4 Ga depression located near the equator of the planet, on the eastern border of Chryse Planitia. It is the landing site chosen for the ESA (European Space Agency)-Roscosmos ExoMars 2022 mission. The Rosalind Franklin rover will touch down on 10 June 2023 to begin its search for traces of past and present life on Mars and to study the aqueous environment (Vago et al., Reference Vago, Westall, Coates, Jaumann, Korablev, Ciarletti, Mitrofanov, Josset, De Sanctis, Bibring, Rull, Goesmann, Steininger, Goetz, Brinckerhoff, Szopa, Raulin, Westall, Edwards, Whyte, Fairén, Bibring, Bridges, Hauber, Ori, Werner, Loizeau, Kuzmin, Williams, Flahaut, Forget, Vago, Rodionov, Korablev, Svedhem, Sefton-Nash, Kminek, Lorenzoni, Joudrier, Mikhailov, Zashchirinskiy, Alexashkin, Calantropio, Merlo, Poulakis, Witasse, Bayle, Bayón, Meierhenrich, Carter, García-Ruiz, Baglioni, Haldemann, Ball, Debus, Lindner, Haessig, Monteiro, Trautner, Voland, Rebeyre, Goulty, Didot, Durrant, Zekri, Koschny, Toni, Visentin, Zwick, van Winnendael, Azkarate and Carreau2017). Oxia Planum is particularly flat and contains clay-rich lithofacies that suggest past water activity in the region. The clay deposits show absorption bands centred at 1.9 and 2.3 μm that are characteristic of Fe/Mg clays, probably a vermiculite-smectite type of clay (Carter et al., Reference Carter, Quantin, Thollot, Loizeau, Ody and Lozach2016). In some places, an additional spectral absorption at 2.5 μm likely suggests the presence of a carbonate or other type of clay mineral. If carbonate phases are present, the site may have been preserved from any acidic environment, which enhances its potential for preserving traces of life (Mandon et al., Reference Mandon, Parkes Bowen, Quantin-Nataf, Bridges, Carter, Pan, Beck, Dehouck, Volat, Thomas, Cremonese, Tornabene and Thollot2021).
The layered clay-bearing unit has a total thickness of 100 m, with each layer varying from 0.7 to 3 m in thickness. According to HiRISE images, the clay-rich facies corresponds to a fractured material of different tonalities that is widely exposed and can be divided into two sub-units: the first one has a reddish tone with smaller fractures and the second one overlaps the first one and has a bluish tone with wider fractures (Quantin-Nataf et al., Reference Quantin-Nataf, Carter, Mandon, Thollot, Balme, Volat, Pan, Loizeau, Millot, Breton, Dehouck, Fawdon, Gupta, Davis, Grindrod, Pacifici, Bultel, Allemand, Ody, Lozach and Broyer2021). The colour difference is explained by mineralogical composition, rather than variable surficial dust or sand cover (Mandon et al., Reference Mandon, Parkes Bowen, Quantin-Nataf, Bridges, Carter, Pan, Beck, Dehouck, Volat, Thomas, Cremonese, Tornabene and Thollot2021). The clay-rich deposits cover terrains of mid- and late-Noachian age, draping the paleotopography, therefore, the unit is mid-Noachian or younger. These strata are systematically overlain by a thin, mid-toned, mantling unit that appears to be indurated and layered with a thickness of ~5 m (Quantin-Nataf et al., Reference Quantin-Nataf, Carter, Mandon, Thollot, Balme, Volat, Pan, Loizeau, Millot, Breton, Dehouck, Fawdon, Gupta, Davis, Grindrod, Pacifici, Bultel, Allemand, Ody, Lozach and Broyer2021). The clays may have a marine or lacustrine sedimentary origin. Other units present in the region include deltaic and fluvial deposits, remnant rounded buttes and a dark resistant unit. The latter is recognizable by its rough, dark and massive appearance and it notably overlies the other units. Its thickness is ~20 m, it appears to be composed of mafic minerals, and is probably of volcanic origin, possibly Amazonian in age (Carter et al., Reference Carter, Quantin, Thollot, Loizeau, Ody and Lozach2016; Quantin-Nataf et al., Reference Quantin-Nataf, Carter, Mandon, Thollot, Balme, Volat, Pan, Loizeau, Millot, Breton, Dehouck, Fawdon, Gupta, Davis, Grindrod, Pacifici, Bultel, Allemand, Ody, Lozach and Broyer2021). Thus, volcanic rocks covered the clays and other aqueous deposits, preserving potential biosignatures from the severe radiation and oxidation of the surface of the planet. The clay-bearing facies have been exposed by erosion relatively recently.
Evaporite minerals
Inorganic precipitation from supersaturated solutions forms evaporitic deposits in a variety of marine and terrestrial environments. Evaporite deposits – soluble salts precipitating from brines (Warren, Reference Warren2016) – and minerals have good biosignature preservation potential because they can embed and preserve sediments containing organic matter, as well as trapping microorganisms including endoliths and fluid-gas-solid inclusions. Such deposits can be preserved over long periods of geological time (e.g. Shkolyar and Farmer, Reference Shkolyar and Farmer2018; Benison, Reference Benison2019). Rapid growth of halite and gypsum from brines may easily entrap primary fluid inclusions that could serve as proxies for environmental conditions, including life. A number of taphonomic processes in evaporites and postdepositional changes, such as sediment phase solubility, porosity, permeability and postdepositional contamination, which can negatively affect the preservation of biosignatures (e.g. Barbieri, Reference Barbieri, de Vera and Seckbach2013). Nevertheless, there are several factors that can enhance biosignature preservation even though the window for preservation occurs under a narrow range of conditions that exist immediately after deposition (e.g. Shkolyar and Farmer, Reference Shkolyar and Farmer2018; Benison, Reference Benison2019). For instance, in hypersaline sabka environments, the dense-stratified brines can increase bottom-water anoxia and promote organic preservation (e.g. Westall and Cavalazzi, Reference Westall, Cavalazzi, Thiel and Reitner2011). On Earth, many kinds of halophiles have been isolated from a wide range of evaporitic environments, including sabka, salt marshes, undersea salt domes and subterranean halite deposits from evaporated ancient seas (e.g. Warren, Reference Warren2016; Benison, Reference Benison2019). Microorganisms have been isolated from salt crystals, evaporite deposits and associated fluid inclusions, in the rock record (Stan-Lotter et al., Reference Stan-Lotter, Pfaffenhuemer, Legat, Busse, Radax and Gruber2002; Vreeland et al., Reference Vreeland, Jones, Monson, Rosenzweig, Lowenstein, Timofeeff, Satterfield, Cho, Park and Wallace2007; Schubert et al., Reference Schubert, Lowenstein, Timofeeff and Parker2010; Fendrihan et al., Reference Fendrihan, Dornmayr-Pfaffenhuemer, Gerbl, Holzinger, Grösbacher, Briza, Erler, Gruber, Plätzer and Stan-Lotter2012; Carnevale et al., Reference Carnevale, Gennari, Lozar, Natalicchio, Pellegrino and Dela Pierre2019). For example, great palaeobiodiversity is described for the Miocenic Messinian evaporitic deposits, Italy (Carnevale et al., Reference Carnevale, Gennari, Lozar, Natalicchio, Pellegrino and Dela Pierre2019). Recently, the unique extreme environments at the Dallol geothermal area, Ethiopia (Cavalazzi et al., Reference Cavalazzi, Barbieri, Gómez, Capaccioni, Olsson-Francis, Pondrelli, Rossi, Hickman-Lewis, Agangi, Gasparotto, Glamoclija, Ori, Rodriguez and Hagos2019; Cavalazzi and Filippidou, Reference Cavalazzi, Filippidou, Branislav, Joseph and Roger2021) have provided the opportunity to study life at its biophysical limits in a hypersaline poly-extreme environment (Belilla et al., Reference Belilla, Moreira, Jardillier, Reboul, Benzerara, López-García, Bertolino, López-Archilla and López-García2019; Gómez et al., Reference Gómez, Cavalazzi, Rodríguez, Amils, Ori, Olsson-Francis, Escudero, Martínez and Miruts2019). Importantly, MISS are common in such environments where evaporate minerals may form on and within the microbial mats (Noffke, Reference Noffke2021).
Evaporites on Mars: Evaporite minerals and deposits, such as hydrated Mg or Fe sulphates, have been observed on Mars both from orbit and in situ (Squyres et al., Reference Squyres, Knoll, Arvidson, Clark, Grotzinger, Jolliff, McLennan, Tosca, Bell and Calvin2006; Rapin et al., Reference Rapin, Meslin, Maurice, Vaniman, Nachon, Mangold, Schröder, Gasnault, Forni and Wiens2016), suggesting formation under evaporitic conditions within the context of past shallow lake, mudflat/sandflat and eolian environments, as well as past shallow acid saline groundwaters (Grotzinger et al., Reference Grotzinger, Arvidson, Bell Iii, Calvin, Clark, Fike, Golombek, Greeley, Haldemann and Herkenhoff2005; Hynek et al., Reference Hynek, Osterloo and Kierein-Young2015; Lynch et al., Reference Lynch, Horgan, Munakata-Marr, Hanley, Schneider, Rey, Spear, Jackson and Ritter2015; Pondrelli et al., Reference Pondrelli, Rossi, Le Deit, Fueten, Schmidt, van Gasselt, Hauber and Pozzobon2017; Benison, Reference Benison2019). Sulphate- and other salt-bearing deposits occur in several places on Mars, including Meridiani Planum, Gusev Crater and Gale Crater (Grotzinger et al., Reference Grotzinger, Arvidson, Bell Iii, Calvin, Clark, Fike, Golombek, Greeley, Haldemann and Herkenhoff2005; Ehlmann et al., Reference Ehlmann, Anderson, Andrews-Hanna, Catling, Christensen, Cohen, Dressing, Edwards, Elkins-Tanton, Farley, Fassett, Fischer, Fraeman, Golombek, Hamilton, Hayes, Herd, Horgan, Hu, Jakosky, Johnson, Kasting, Kerber, Kinch, Kite, Knutson, Lunine, Mahaffy, Mangold, McCubbin, Mustard, Niles, Quantin-Nataf, Rice, Stack, Stevenson, Stewart, Toplis, Usui, Weiss, Werner, Wordsworth, Wray, Yingst, Yung and Zahnle2016; Pondrelli et al., Reference Pondrelli, Rossi, Le Deit, Fueten, Schmidt, van Gasselt, Hauber and Pozzobon2017). The thermochemical stability of such deposits under Martian conditions would enhance their potential for preserving possible halophilic microorganisms.
At Gale crater, for example, muddy to sandy sediments deposited in a lacustrine environment are characterized by a network of fractures filled in with light-toned deposits of sulphate evaporites, as well as nodules and raised ridges of similar materials. The fractures formed in the consolidated sediments owing to subsequent compression and extension related to fluid overpressure and cracking (De Toffoli et al., Reference De Toffoli, Mangold, Massironi, Zanella, Pozzobon, Le Mouélic, L'haridon and Cremonese2020). They were then infilled by briny solutions that deposited the evaporite mineral suites. Rapin et al. (Reference Rapin, Ehlmann, Dromart, Schieber, Thomas, Fischer, Fox, Stein, Nachon and Clark2019) documented bulk enrichments within the bedrock of significant amounts of calcium sulphate intercalated with a stratum enriched in hydrated magnesium sulphate resulting from early diagenetic, pre-compaction salt precipitation from brines concentrated by evaporation. Such environments would be habitable for halophilic-type microorganisms.
Experimental studies document the ability of halophiles to survive when exposed to surface Mars conditions (e.g. UV radiation, cold temperatures and desiccation; Oren, Reference Oren2014). For all these reasons, evaporite deposits are a key exploration target for the ongoing astrobiological exploration of Mars and for future Mars Sample Return programs (Hays et al., Reference Hays, Graham, Des Marais, Hausrath, Horgan, McCollom, Parenteau, Potter-McIntyre, Williams and Lynch2017).
Concluding remarks and significance for Mars missions
The McKay et al. (Reference McKay, Gibson, Thomas-Keprta, Vali, Romanek, Clemett, Chillier, Maechling and Zare1996) paper was a seminal benchmark that stimulated the opening of the field of astrobiology and the search for life on Mars. Despite the fact that the weight of subsequent experiments and understanding of the microbial world and its preservation in the rock record shows that the original conclusions by McKay et al. were incorrect, their multiscale and multi-technique approach to documenting a variety of types of complementary, potential biosignatures paved the way for future work. A key parameter that was missing was the in situ geological context (which could, however be surmised).
Most important over the last quarter century is the cross-disciplinary understanding between experts in specific domains that is the only path to providing a holistic understanding of traces of past microbial life, both on Earth and elsewhere in the Solar System. It is the complementary expertise that will enable deciphering the rock record of Mars with the missions Mars Science Laboratory, Mars 2020 and ExoMars 2022 to determine habitability and to identify any potential evidence of past life (without excluding the minute possibility of extant life).
In this mini-review, we have discussed the nature of biosignatures and their formation and preservation through the lens of the ALH84001 study. We have documented a non-exhaustive but pertinent variety of mineral phases that can preserve biosignatures, including carbonates, silica, phyllosilicates and evaporite phases, both in surface environments and in the subsurface. This latter point is of particular interest for the ExoMars 2022 mission because of the ability of the rover to drill to depths of up to 2 m, a first in the history of Mars exploration. The importance of this feat is that organic biosignatures (or even abiotic/prebiotic organic signatures) will be better protected from the deleterious effects of surface oxidation and UV radiation at such depths. Secondly, the targeted site, Oxia Planum, comprises clay-enriched lithologies. As we have seen above, phyllosilicates have high propensity for trapping, chelating and protecting organic molecules. Thirdly, Oxia Planum at ~4–3.9 Ga is the oldest location yet to be investigated, having formed during a period when Mars had wetter and more habitable conditions. Thus, if there are preserved traces of Martian life at the landing site, the ExoMars 2022 rover, Rosalind Franklin, should be able to uncover them.
Supplementary material
The supplementary material for this article can be found at https://doi.org/10.1017/S1473550421000264.
Conflict of interest
None.