Introduction
Understanding the origin and characteristics of basal ice beneath glaciers and ice sheets is important for glaciologists and geomorphologists because basal ice influences ice motion, provides information on glaciological processes and conditions at the base of ice masses, and is important for glacial sediment entrainment, transfer and deposition (Reference Hubbard and SharpHubbard and Sharp, 1989; Reference KnightKnight, 1997). Dispersed facies basal ice (defined as dispersed debris particles and aggregates within a clean ice matrix, using the terminology of Reference LawsonLawson, 1979) is of particular interest because it has commonly been associated with formation in the deep interior of ice masses and is therefore potentially important in conveying information about subglacial processes and conditions in highly inaccessible locations (e.g. Reference SugdenSugden and others, 1987). Furthermore, numerous studies have described a basal ice facies at many different ice masses, which is descriptively similar at all sites, and could be classified as dispersed facies basal ice (hereafter referred to simply as ‘dispersed facies’), although several different names have been applied (e.g. the ‘dispersed facies’ of Reference LawsonLawson (1979), the ‘clotted facies’ of Reference Knight, Sugden and MintyKnight and others (1994) and the ‘clear facies’ of Reference Hubbard, Tison, Janssens and SpiroHubbard and others (2000); Table 1).
Table 1. Key characteristics of dispersed facies basal ice (and descriptively similar basal ice facies with different names) outlined in previous studies

Basal ice stratigraphy around glacier margins can be very variable, but the generalized stratigraphy proposed by Reference KnightKnight (1994) is of a lowermost, debris-rich, layered facies termed ‘stratified facies’ overlain unconformably by dispersed facies. Dispersed facies is in turn typically overlain by meteorically derived glacier ice (englacial facies), although the contact between these facies is typically diffuse, leading several authors to propose a genetic relationship between the two (e.g. Reference LawsonLawson, 1979). It is also common to find debris bands (sometimes referred to as ‘banded facies’; Reference KnightKnight, 1987, Reference Knight1994) intercalated with dispersed facies or englacial facies. Debris bands have been suggested to be tectonically attenuated debris-rich ice derived from the stratified facies or from underlying subglacial sediments (e.g. Reference HartHart, 1995, Reference Hart1998; Reference Waller, Hart and KnightWaller and others, 2000). Though basal ice is important for transferring debris through the glacier system and for building till sequences and moraines (e.g. Reference LawsonLawson, 1979; Reference Knight, Waller, Patterson, Jones and RobinsonKnight and others, 2002), estimates of debris flux in basal ice layers are not common, and have largely been made for debris-rich stratified facies basal ice (e.g. Reference LarsonLarson and others, 2006; Reference Cook, Robinson, Fairchild, Knight, Waller and BoomerCook and others, 2010), with few measurements made of dispersed facies (e.g. Reference Knight, Waller, Patterson, Jones and RobinsonKnight and others, 2002).
Despite its potential importance and apparent ubiquity, the dispersed facies remains enigmatic, with several hypotheses proposed for its formation. Hypothesized mechanisms include those of a broadly sedimentological nature in which the dispersed facies is passively emplaced, including freezing-on of ice and sediment subglacially (Reference Christoffersen, Tulaczyk, Carsey and BeharChristoffersen and others, 2006), passive burial of sediment deposited supraglacially in the accumulation area (Reference KoernerKoerner, 1989), and transport of sediment through the intercrystalline vein network (Reference Knight and KnightKnight and Knight, 1994) by water generated by rigid-bed regelation (Reference KnightKnight, 1987; Reference SugdenSugden and others, 1987). Other mechanisms have been of a largely tectonic nature in which dispersed facies is the end-product of strain-induced metamorphism of another ice facies, including englacial ice (Reference Sharp, Jouzel, Hubbard and LawsonSharp and others, 1994; Reference Hubbard, Tison, Janssens and SpiroHubbard and others, 2000) or debris-rich stratified facies basal ice (Reference HartHart, 1995). Table 1 summarizes the key characteristics and proposed mechanisms of formation for the dispersed facies from a number of key studies.
The first aim of our study is to elucidate the origin of the dispersed facies at the temperate glacier Svínafellsjökull, southeast Iceland (Fig. 1). The presence of an icefall and a terminal subglacial overdeepening at Svínafellsjökull may contribute importantly to the origin of dispersed facies at this location in ways that have not been examined. Reference Goodsell, Hambrey and GlasserGoodsell and others (2002) demonstrated that basal materials could be elevated into englacial and even supraglacial positions in folds generated by intense compression at the base of an icefall, with dark ogive bands, which reflect areas of particularly dense foliation, forming planes of weakness along which strain can also be accommodated by thrusting on the scale of the glacier thickness. Reference Spedding and EvansSpedding and Evans (2002) suggested that the presence of a terminal over-deepening may further enhance the elevation of basal material as a result of ice flow against the adverse slope. Reference Swift, Evans and FallickSwift and others (2006) speculated that this might also reactivate thrusting along dark ogive bands to form prominent outcrops of very debris-rich ice in supraglacial locations down-glacier of the overdeepening. Similar processes may influence basal ice formation at Svínafellsjökull.
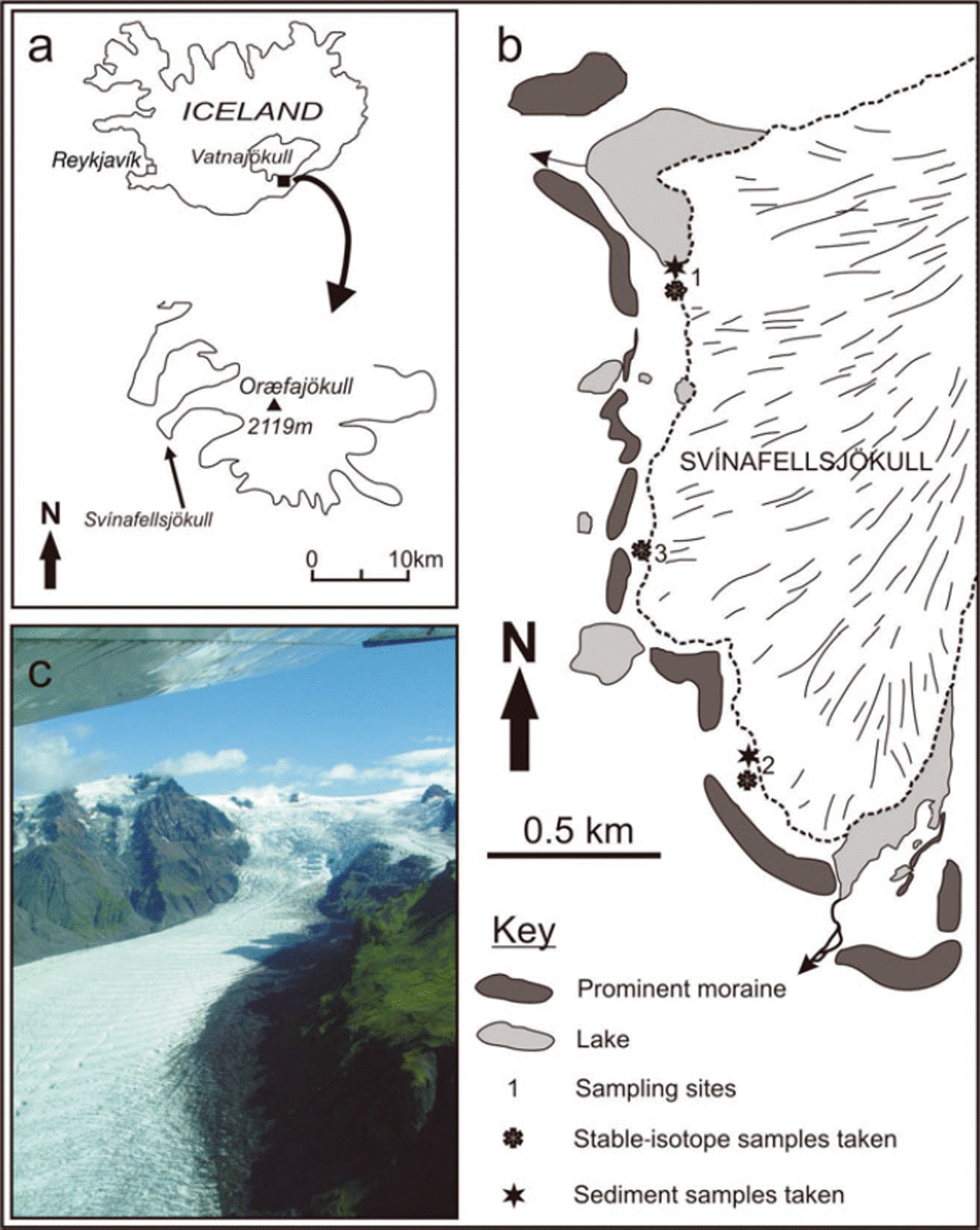
Fig. 1. (a) Regional context of Svínafellsjökull. (b) Map of Svínafellsjökull terminus including sampling locations for sediment particle size and stable-isotope analyses. (c) View of the icefall and ogives.
Our second aim is to assess the contribution of dispersed facies to sediment transfer at Svínafellsjökull following calculations made by Reference Cook, Robinson, Fairchild, Knight, Waller and BoomerCook and others (2010) of debris transfer by the more debris-rich stratified facies at the same glacier.
Study Site and Methods
Dispersed facies was examined at Svínafellsjökull (63°59′ N, 16°52′ W), a temperate outlet glacier of the Oræfajökull ice cap, southeast Iceland (Fig. 1). Typically the dispersed facies is found in metres-thick exposures at the terminus in direct contact with the substrate (glacial moraine/till), although it is occasionally found to be underlain by the more debris-rich and visibly layered stratified facies. The stratified facies has been examined in detail by Reference Cook, Knight, Waller, Robinson and AdamCook and others (2007, Reference Cook, Robinson, Fairchild, Knight, Waller and Boomer2010), who attributed its formation to the tectonic thickening of regelation ice and, in the southern part of the glacier terminus, to freeze-on of water and sediment as a result of glaciohydraulic supercooling.
Many glaciers in this region terminate in overdeepenings (Reference KnudsenKnudsen and others, 2001; Reference RobertsRoberts and others, 2002; Reference Spedding and EvansSpedding and Evans, 2002), and an overdeepening at Svínafellsjökull has been inferred from the distribution of features known to be associated with glaciohydraulic supercooling (Reference Cook, Knight, Waller, Robinson and AdamCook and others, 2007). These included vents of upwelling supercooled water, the presence of anchor-ice terraces and frazil-ice flocs in and adjacent to vents, and crevasses or fractures filled with platy ice (Reference Evenson, Mickelson and AttigEvenson and others, 1999). Also in common with other glaciers in the region is the prominent icefall located ∼6 km from the terminus, a position that coincides with the approximate altitude of the equilibrium line in this region (Reference Spedding and EvansSpedding and Evans, 2002). Ogives appear immediately down-glacier from the icefall spaced on average at a 35 m interval (Fig. 1c; Reference King and IvesKing and Ives, 1956), the arcuate dark ogive bands being composed of strongly foliated ice that occasionally contains subglacially worked clasts. The association of ogives with icefalls and subglacial debris is common to many glaciers in this region (Reference Ives and KingIves and King, 1954; Reference King and IvesKing and Ives, 1956; Reference Swift, Evans and FallickSwift and others, 2006).
Dispersed facies was characterized and logged following Reference LawsonLawson (1979), with description of facies thickness, physical appearance, mean crystal diameter, debris distribution and debris concentration. Measurements of crystal size were facilitated by thin-sectioning blocks of ice to ∼1–2 mm thickness on a hot plate and viewing the thin section through cross-polarizing lenses. Decimetre-scale samples of dispersed facies were removed using an ice axe, after removing the top 20–30 cm of rotted ice. These were taken from sections with a massive structureless appearance with no debris bands present. Sediment samples were extracted from melted ice blocks by filtering. Sediment size distribution was characterized by wet sieving (−4 to 0Φ)) and laser granulometry (0–10Φ)). Descriptive statistics (e.g. Φ) is defined as log2 d, where d is particle diameter (mm)) and sediment classifications for particle size distributions were produced using the GRADISTAT package (Reference Blott and PyeBlott and Pye, 2001).
Stable-isotope analysis of dispersed facies followed similar methods to previous research (e.g. Reference Cook, Robinson, Fairchild, Knight, Waller and BoomerCook and others, 2010). Before sampling the ice, the top 20–30 cm of ice was removed to extract ice unaffected by surficial melting and contamination resulting from the movement of surface meltwater along crystal boundaries. Blocks of ice of ∼30–100 mL were removed (i.e. typically decimetre-scale samples, although occasionally centimetre-scale) and allowed to melt in sealed sample bags. Samples were then filtered through 0.45 μm nitrocellulose filter papers and subsequently stored in airtight bottles. Stable-isotope analysis was undertaken on a GV Instruments Isoprime continuous-flow mass spectrometer. Standardization was carried out by calibration against laboratory and International Atomic Energy Agency (IAEA) reference standards. All analyses were duplicated and internal precision was 0.4% for hydrogen and 0.08% for oxygen, with external precision estimated to be approximately twice these values.
Results
Physical attributes and facies classification
Dispersed facies ice was characterized by a low mean debris concentration of 1.6% by volume (std dev. 2.0%; n = 48) dispersed throughout a clean ice matrix (Fig. 2a and b). Measured debris concentrations ranged from 0.2% to 9.7% by volume. Debris occurred either as individual particles or as irregular-shaped aggregates (typically less than ∼5 mm in diameter) and generally had a polymodal grain-size distribution (see below). Thin sections of the ice revealed that debris was typically found within the ice crystals (i.e. intracrystalline) rather than at crystal boundaries (Fig. 2a). Debris was occasionally concentrated along debris planes separated by the more typical arrangement of uniformly distributed debris (Fig. 2c). Viewed at the centimetre to decimetre scale, dispersed facies appeared massive and structureless, although at the decimetre to metre scale it sometimes exhibited weak layering (Fig. 2d). Bubbles were rare, although where they did exist they were deformed and flattened, and generally less than ∼3 mm in length. Crystal diameters ranged from 0.8 to 6.8 cm, with a mean of 2.9 cm (std dev. 1.5 cm; n = 37).

Fig. 2. Typical characteristics of the dispersed facies including (a, b) dispersed debris aggregates and particles (a) in thin section and (b) in situ, (c) occasional debris-rich planes and (d) the crudely layered structure of the ice.
The underlying stratified facies was generally more debris-rich (3–30% sediment by volume), equally bubble-poor and generally had smaller crystal sizes (0.4–1.8 cm). For detailed comparison with the characteristics of the stratified facies, we refer the reader to Reference Cook, Knight, Waller, Robinson and AdamCook and others (2007, Reference Cook, Robinson, Fairchild, Knight, Waller and Boomer2010). Overlying glacier ice (englacial facies) was characteristically blue or white, with a high concentration of bubbles and low debris content (0.04% by volume; n = 4). Glacier ice crystal diameters were larger on average than dispersed facies crystals, 1.8–7.5 cm with a mean diameter of 3.8 cm (std dev. 1.5 cm; n = 20). Debris was similarly polymodal, although insufficient debris was recovered from glacier ice to allow detailed particle size analysis.
Exposures of dispersed facies were typically sub-vertical at the glacier margin, with exposures of 0.5–15 m in height. In most cases, the base of the dispersed facies could be seen, except in the southern portion of the glacier where it is receding into an overdeepening. Dispersed facies could be traced up-glacier across the glacier surface for several tens of metres. Foliation planes within the dispersed facies and overlying glacier ice typically dipped up-glacier at 10–20°, so true thicknesses in this area were up to ∼25 m. Dispersed facies ice was also observed within laterally discontinuous bands that outcropped up to ∼400 m up-glacier of the main ice-marginal exposures. These bands contained aggregates or angular clasts of yellow or red tephra or rock, and were not sampled for sediment or isotope analyses.
The naming of different basal ice facies has been inconsistent between studies (Reference Hubbard, Cook and CoulsonHubbard and others, 2009). We have adopted the term ‘dispersed facies’ of Reference LawsonLawson (1979) because it is the most widely used. However, to facilitate future comparisons between similar ice facies at other glaciers, we classify dispersed facies basal ice at Svinafellsjökull according to the unified basal ice facies classification scheme of Reference Hubbard, Cook and CoulsonHubbard and others (2009). As such, dispersed facies at Svínafellsjökull includes Dispersed (D) (where no layering is visible), Dispersed Stratified (DSt) (where layering is on the scale of metres) and Dispersed Banded (DB) (where layering is on the scale of decimetres) basal cryofacies.
Spatial distribution
Dispersed facies occurred almost ubiquitously around the glacier terminus. It was found either in direct contact with the substrate or was separated from it by a layer of debris-rich stratified facies. Where they were found together, dispersed facies and stratified facies had a sharp, unconformable contact. Bubble-foliated glacier ice derived from meteoric sources lay stratigraphically above the dispersed facies, and the boundary between the two ice types was diffuse. Fractures filled with platy ice were common in the southern reaches of the glacier (Reference Cook, Knight, Waller, Robinson and AdamCook and others, 2007) and represent the injection of supercooled water along with sediment into crevasses and fractures where the glacier enters an overdeepening. These structures have been injected into and cross-cut the dispersed facies, suggesting that the dispersed facies developed up-glacier of the terminal overdeepening.
Sediment characteristics
Figure 3 presents sediment particle size distributions for dispersed facies from two locations. There was significant variability between individual particle size distributions, which were generally polymodal. Samples at site 1 (location in Fig. 1b) both contained high proportions of pebbles, granules and coarse sand, and one of the two samples contained a high proportion of silt (Fig. 3a). At site 2 (Fig. 3b), there was a much lower proportion of pebbles, granules and coarse sand, with samples generally dominated by fine sand and silt. The mean particle size for the dispersed facies at Svínafellsjökull was 2.95 ± 3.62Φ) (fine sand; n = 9). Figure 4 demonstrates that debris volumes measured through the thickness of dispersed facies at the glacier margin did not vary systematically with height above the glacier bed over scales of metres (Fig. 4a–e) to tens of metres (Fig. 4f).

Fig. 3. Particle size distributions for (a) site 1 and (b) site 2 (see Fig. 1b for site locations).

Fig. 4. Change in debris volume within dispersed facies with height above the glacier bed, from site 1 (a–c), site 3 (d, e) and site 2 (f).
Stable-isotope analysis
Figure 5 presents stable-isotope plots of δ18O against δD for dispersed facies, and results are summarized in Table 2. A local meteoric waterline (LMWL) is plotted on each graph in Figure 5 based on the data of Reference RobinsonRobinson (2003) and Reference Robinson, Fairchild and ArrowsmithRobinson and others (2009) from nearby Skeiðarárjökull (∼10.5 km west of Svínafellsjökull). This was also used as a reference water composition for Svínafellsjökull by Reference Cook, Robinson, Fairchild, Knight, Waller and BoomerCook and others (2010). This LMWL has a slope of 8.46 and is plotted through the lightest values in the dataset to facilitate comparison with dispersed facies ice compositions (i.e. it is an adjusted LMWL). An envelope encapsulating the isotope compositions of stratified facies samples is plotted for comparison based on the data of Reference Cook, Robinson, Fairchild, Knight, Waller and BoomerCook and others (2010).

Fig. 5. Stable-isotope composition of dispersed facies: (a) composition of all dispersed facies, glacier ice and stratified facies samples; (b–d) composition of dispersed facies samples at (b) site 1, (c) site 2 and (d) site 3.
Table 2. Summary of the stable-isotope compositions of dispersed facies and glacier ice samples

Figure 5a shows all stable-isotope data for dispersed facies and glacier ice. Dispersed facies compositions plot with a regression slope of 4.7 (r 2 = 0.92; Table 2). Glacier ice compositions plot with a regression slope of 8.6 (r 2 = 0.74), similar to the adjusted LMWL and typical of ice derived directly from meteoric sources (e.g. Reference Jouzel and SouchezJouzel and Souchez, 1982). Dispersed facies samples generally have a lighter composition than stratified facies samples, although there is overlap between compositions. Comparison between Figure 5b, c and d (see also Table 2) shows that there was some compositional variability of dispersed facies between sites 1, 2 and 3, respectively. However, at all sites, data plot along regression slopes of 5.0–6.5, with high r 2 values (0.91–0.99). The isotope composition of dispersed facies, plotting along a line of lower slope than meteorically derived glacier ice samples, is therefore consistent with isotope fractionation during closed-system freezing (Reference Jouzel and SouchezJouzel and Souchez, 1982).
Discussion
Evaluation of existing theories for dispersed facies formation at Svínafellsjökull
Any theory for the origin of dispersed facies basal ice at Svínafellsjökull must account for the development of its key characteristics. Dispersed facies: (1) is massive (i.e. structureless), with low debris concentrations (mean 1.6% by volume), low bubble content, and crystal size intermediate between that of overlying glacier ice and underlying stratified facies; (2) is almost ubiquitous around the glacier margin and in thicknesses of metres to tens of metres; (3) contains sediment sizes ranging from clay to pebble; (4) shows no systematic decrease or increase in sediment concentration with height above the bed, although it does have a diffuse contact with overlying glacier ice; and (5) has a stable-isotope composition consistent with closed-system freezing. Overall, our results indicate that no single existing theory of dispersed facies formation can fully account for the characteristics of dispersed facies basal ice at Svínafellsjökull. We consider each existing hypothesis in turn, highlighting the reasons for these hypotheses being discounted.
The model of clotted facies formation at Russell Glacier, West Greenland, proposed by Reference SugdenSugden and others (1987), involving alteration of glacier ice by regelation in the interior of the ice mass (Table 1) can explain a number of features of the dispersed facies at Svínafellsjökull. For example, Svínafellsjökull is a temperate glacier that likely moves in part through basal sliding around bedrock obstacles, so the basal layer should be expected to have been affected by regelation (Reference Cook, Robinson, Fairchild, Knight, Waller and BoomerCook and others, 2010). The low debris concentration, diffuse contact with glacier ice, polymodal sediment size distribution and closed freezing system isotope composition are all consistent with an origin through regelation. It is difficult, however, to explain the distribution and full suite of characteristics of dispersed facies through regelation alone. Regelation typically only produces basal ice layers on the order of millimetres to decimetres thickness (e.g. Reference Kamb and LaChapelleKamb and LaChapelle, 1964; Reference Hubbard and SharpHubbard and Sharp, 1993), whereas dispersed facies at Svínafellsjökull is typically several metres to tens of metres thick. Thus, to explain the thickness of dispersed facies through regelation requires regelation ice to have been thickened tectonically.
Tectonic thickening can happen in one of two ways. Firstly, Reference Knight, Sugden and MintyKnight and others (1994) suggested that variation in the spatial distribution of clotted ice at Russell Glacier may represent thickening of a regelation layer by plastic flow around large bedrock obstacles close to the ice-sheet margin. Such obstacles are not evident at the margin of Svínafellsjökull. Likewise, the variability in distribution and thickness of the clotted facies at Russell Glacier is not observed for the dispersed facies at Svínafellsjökull. Secondly, tectonic thickening could occur against the adverse slope of the overdeepening. Regelation layers up to 2 m thick were observed within the stratified facies by Reference Cook, Knight, Waller, Robinson and AdamCook and others (2007, Reference Cook, Robinson, Fairchild, Knight, Waller and Boomer2010), with some regelation layers displaying evidence of having been thickened (i.e. the presence of folds and faults). This, however, was limited to the stratified facies and to a scale that could not explain the observed thicknesses of dispersed facies, and fracture-fills that crosscut the dispersed facies do not show evidence of deformation. It therefore seems unlikely that regelation and tectonic thickening in the terminal region of the glacier are sufficient to explain the thickness of dispersed facies at Svínafellsjökull.
Another possible formation mechanism that is closely related to the rigid-bed regelation hypothesis is the generation of clots as a result of water and sediment being squeezed away from bedrock obstacles into the intercrystalline vein network (Table 1). This leads to the accumulation of fine-grained sediments between crystal boundaries (Reference Knight and KnightKnight and Knight, 1994). Reference Spedding and EvansSpedding and Evans (2002) suggested that basal ice at nearby Kvíárjökull was formed in this manner, with the additional effect of tectonic thickening as a consequence of compressive flow near the terminus. This hypothesis is refuted because: (1) dispersed facies debris at Svínafellsjökull includes pebbles and coarse sand, which are too coarse to be transported through the intercrystalline vein network (Fig. 3); (2) this debris is often found within crystals, rather than at triple-grain boundaries (Fig. 2); and (3) there is no systematic reduction in sediment concentration with height above the bed (Fig. 4), as would be expected if water had been squeezed upward from an underlying sediment source or debris-rich ice layer.
Reference HartHart (1995) suggested an alternative model of dispersed facies formation involving the tectonic attenuation of debris from a lowermost debris-rich basal ice or subglacial sediment layer into the overlying glacier ice (Table 1). This hypothesis is also rejected at Svínafellsjökull. Firstly, Reference HartHart (1995) suggested that debris concentration and debris aggregate size should reduce with height above the bed, which is inconsistent with the irregular changes in debris concentration with height above the bed observed at Svínafellsjökull (Fig. 4). Secondly, this model presumes that the host ice facies into which debris-rich basal ice or subglacial sediment has been entrained is meteorically derived englacial facies glacier ice, the isotope composition of which should plot along a line of similar slope to that of the LMWL. At Svínafellsjökull the samples of this ice plot along a freezing slope of lower gradient (Fig. 5; Table 2), implying a different origin.
Another tectonic mechanism has been proposed by Reference Hubbard, Tison, Janssens and SpiroHubbard and others (2000) for the ‘clear facies’ at Tsanfleuron, Switzerland, in which meteorically derived glacier ice is metamorphosed by internal deformation close to the ice/bedrock interface (Table 1). Comparison of the characteristics of dispersed facies at Svínafellsjökull with the clear facies at Tsanfleuron indicates that this model does indeed account for many of the characteristics of the ice, including the low bubble concentrations, occasionally deformed and flattened bubbles, metre thicknesses of massive structureless basal ice, and the diffuse boundary with overlying glacier ice. However, it would be expected that this process would produce ice with an isotope composition similar to that of the LMWL, whereas dispersed facies samples from Svínafellsjökull plot instead along a closed-system freezing slope (Fig. 5; Table 2), and so this hypothesis is also rejected.
The interpretation by Reference KoernerKoerner (1989) of basal ice in cores from Canadian Arctic islands and from Greenland as buried superimposed ice containing wind-blown sediment raises another potential mechanism: the incorporation of surficial sediment. Some englacial debris at Svínafellsjökull undoubtedly enters the glacier via crevasses, especially those formed at the icefall, some of which may penetrate to considerable depth. This is supported by the presence of laterally discontinuous bands of dispersed facies at Svínafellsjökull containing red and yellow angular tephra/rock that presumably reflect individual rockfall events from the valley side-walls. The critical test of this mechanism is that the isotope composition of the host ice must be consistent with that of meteorically derived englacial facies glacier ice, which is not the case (Fig. 5; Table 2). Further, it is not clear how debris derived from rockfall or aeolian deposition could form such a homogeneous and discrete layer close to the glacier bed.
Finally, processes of basal adfreezing must also be considered, as these have been invoked in other studies to explain the origin of facies similar to dispersed facies. Reference Christoffersen, Tulaczyk, Carsey and BeharChristoffersen and others (2006) showed that freeze-on of pore-water from subglacial sediments can produce ‘clean’ or ‘clear’ facies (the authors use the terms interchangeably) beneath Antarctic ice streams. However, it is unlikely that such processes operate to produce metres to tens of metres of basal ice beneath temperate valley glaciers where environmental conditions are quite different to those beneath Antarctic ice streams. Further, since Reference Souchez, Samyn, Lorrain, Pattyn and FitzsimonsSouchez and others (2004) have predicted that the stable-isotope composition of basal ice produced by adfreezing would not show isotope fractionation, isotopic composition again provides a critical test which leads us to refute this hypothesis, specifically because samples from Svínafellsjökull plot along a freezing slope (Fig. 5; Table 2).
Though not considered to be an established mechanism of dispersed facies formation, glaciohydraulic supercooling has previously been linked to the formation of metres thicknesses of basal ice (e.g. Reference Lawson, Strasser, Evenson, Alley, Larson and ArconeLawson and others, 1998; Reference Cook, Robinson, Fairchild, Knight, Waller and BoomerCook and others, 2010), and it is therefore important to carefully exclude this as a potential mechanism. Firstly, features associated with supercooling (e.g. fracture-fills) always cross-cut dispersed facies or underlie dispersed facies with an unconformable contact (e.g. stratified facies), indicating that dispersed facies is formed by a separate process that operates up-glacier of the overdeepening. Secondly, the isotope composition of dispersed facies ice is different from basal ice types formed by supercooling, in that it plots along a freezing slope of lower gradient than the LMWL (Fig. 5; Table 2) (cf. Reference Cook, Robinson, Fairchild, Knight, Waller and BoomerCook and others, 2010). It is of course possible that supercool ice forms a small component of the dispersed facies, especially if there are multiple overdeepenings along the glacier length, and that subsequent strain and/or regelation is sufficient to alter significantly the debris distribution and isotope composition. Nevertheless, supercooling is typically only locally significant (e.g. Reference Spedding and EvansSpedding and Evans, 2002; Reference Tweed, Roberts and RussellTweed and others, 2005; Reference Cook, Knight, Waller, Robinson and AdamCook and others, 2007, Reference Cook, Robinson, Fairchild, Knight, Waller and Boomer2010), whereas dispersed facies at Svínafellsjökull is ubiquitous, making it difficult to envisage supercooling as the primary source.
A model of dispersed facies formation at Svínafellsjökull
None of the aforementioned theories of dispersed facies formation satisfactorily explains the origin of dispersed facies at Svínafellsjökull. Perhaps the most striking observation at Svínafellsjökull is that the dispersed facies is almost ubiquitous around the glacier margin and occurs in metres-thick sequences. Hence, the mechanism(s) responsible for its formation must operate pervasively across the glacier width, and up to tens of metres at the glacier base. We therefore illustrate a new model for dispersed facies formation that is partly dependent on the glacier-wide elevation of basal debris throughout the glacier thickness by processes operating at the base of the icefall (Fig. 6) (cf. Reference Goodsell, Hambrey and GlasserGoodsell and others, 2002; Reference Swift, Evans and FallickSwift and others, 2006).

Fig. 6. Conceptual model of dispersed facies formation through entrainment of sediment at the base of an icefall followed by ice metamorphism. (a) Sediment entrainment at the base of an icefall through shearing and folding to form ogives. (b) Basal part of ogive is metamorphosed through regelation and strain to form dispersed facies basal ice. (c) Final stratigraphy at glacier terminus includes stratified facies accreted from supercooled water in the overdeepening, the possible tectonic thickening of basal ice as the glacier flows against the reverse slope of the overdeepening, and the possible effect of inefficient subglacial drainage across the overdeepening, allowing basal ice to survive in metres thicknesses.
The processes of entrainment envisaged in this model have previously been inferred by Reference Goodsell, Hambrey and GlasserGoodsell and others (2002) from observations of ogive formation at Bas Glacier d’Arolla, Switzerland (Fig. 6a). Notably, Reference Goodsell, Hambrey and GlasserGoodsell and others (2002) found that folding at the base of the icefall generated areas of particularly dense foliation (i.e. dark ogive bands) that formed planes of weakness along which folding and thrusting had entrained both basal ice and debris. Reference Swift, Evans and FallickSwift and others (2006) later adapted this model to explain the formation of englacial debris-rich basal ice bands at Kvíárjökull. They argued that initial folding at the base of an icefall could be followed by shearing of the fold structures and the direct entrainment of basal ice and debris along the shear zone. This results in a series of parallel arcuate glacier-wide folds containing basal ice and debris, with central debris-rich thrust planes, that are relatively broad at the base and thin towards the surface (Reference Swift, Evans and FallickSwift and others, 2006, fig. 7b). The presence of ogives immediately down-glacier of the icefall implies similar mechanisms of debris elevation at Svínafellsjökull (Fig. 1c). Debris from surficial sources (tephra, wind-blown dust, rockfall from valley sides) may also be incorporated into the ice via open crevasses in the region of the icefall.
Following initial entrainment at or near the icefall, our model envisages metamorphosis and mixing of the englacially elevated basal ice and adjacent englacial ice as a result of regelation and strain experienced during the ∼6 km of ice flow between the icefall and the terminus. The closed-system freezing origin, indicated by the stable-isotope composition, is consistent with the influence of regelation (e.g. Reference Hubbard and SharpHubbard and Sharp, 1993), while occasional deformed and flattened bubbles indicate the influence of strain. Both these processes have been invoked separately to explain tens of metres of dispersed-type ice (e.g. Reference SugdenSugden and others, 1987; Reference Hubbard, Tison, Janssens and SpiroHubbard and others, 2000). In combination they will allow the mixing and metamorphosis of ice to several metres or even tens of metres above the bed, producing the uniform massive structure of the dispersed facies and characteristic diffuse interface with the overlying glacier ice (Fig. 6b), and with the surface expression of the ogives remaining largely unchanged. Other studies too have considered dispersed facies to have been generated by high strain following initial sediment entrainment into englacial ice (e.g. Reference HartHart, 1995, Reference Hart1998; Reference Waller, Hart and KnightWaller and others, 2000).
It is also possible that dispersed facies characteristics consistent with regelation (e.g. closed-system isotope composition) are inherited from the entrainment of regelation basal ice into thrusts and folds at the icefall. Furthermore, between the thrust planes that penetrate to the glacier surface to form ogives, there are likely to be a number of minor thrusts and folds. These will not reach the glacier surface, but may elevate basal ice and sediment to several metres or tens of metres above the glacier bed. These smaller tectonic features generated at the base of the icefall may also be important in the generation of dispersed facies, and will also experience strain and regelation with transport to the terminus.
The terminal overdeepening may also play a role in the development of dispersed facies at Svínafellsjökull (Reference Swift, Evans and FallickSwift and others, 2006), although the evidence for such an influence is less convincing (Fig. 6c). It is possible that glacier flow against the adverse slope of the overdeepening has caused tectonic thickening of the dispersed facies (or has done in the recent past). Notably, tectonic features such as shear planes and overturned folds (nappes) have been observed in the stratified facies (Reference Cook, Robinson, Fairchild, Knight, Waller and BoomerCook and others, 2010), and seem to explain the thickening of centimetre- and decimetre-scale regelation layers to metre-scale layers. However, there is little direct evidence for the thickening of such layers on the scale of many metres. Furthermore, the dispersed facies is cross-cut by features associated with the operation of glaciohydraulic supercooling in the over-deepening (e.g. fractures filled with platy ice; Reference Cook, Knight, Waller, Robinson and AdamCook and others, 2007) that do not display any tectonic disturbance. This suggests that dispersed facies develop to their full extent before reaching the terminal overdeepening.
A further factor likely to favour thick dispersed facies is the possible lack of efficient subglacial drainage in the region of the overdeepening, which should allow basal ice to survive in greater thicknesses (e.g. Reference SpeddingSpedding, 2000; Reference Spedding and EvansSpedding and Evans, 2002). The nature of the subglacial drainage system at Svínafellsjökull remains unknown, but it is likely that the presence of an inefficient subglacial drainage system is important in enabling the development of the significant thicknesses of dispersed facies at this location.
Significance of dispersed facies at Svínafellsjökull
Relatively few studies have quantified sediment fluxes from the basal ice layer, and fewer still have quantified this for individual basal ice facies. We use the steady-state sediment flux equation of Reference Cook, Robinson, Fairchild, Knight, Waller and BoomerCook and others (2010) to quantify sediment flux for the dispersed facies. In our calculation we use a mean dispersed facies thickness of 3.5 m across the 2 km of the glacier margin, a mean sediment content of 1.6% by volume, and measured glacier terminus velocity of 14.6–29.2 m a−1. This gives a sediment flux of 0.81–1.62 m3 m−1 a−1. This value is lower than that calculated by Reference Cook, Robinson, Fairchild, Knight, Waller and BoomerCook and others (2010) for the stratified basal ice produced by supercooling and by regelation which has sediment fluxes of 4.8–9.6 and 1.0– 2.0 m3 m−1 a−1 respectively. Therefore, on a local scale, dispersed facies contributes much less sediment to the proglacial environment during melt-out than does stratified facies. Reference Knight, Waller, Patterson, Jones and RobinsonKnight and others (2002) measured a debris flux of 7.2 m3 m−1 a−1 for the dispersed facies of Russell Glacier. This value is higher than our calculated sediment flux for dispersed facies at Svínafellsjökull largely because of the higher mean thickness (15 m) of dispersed facies measured by Reference Knight, Waller, Patterson, Jones and RobinsonKnight and others (2002) at Russell Glacier.
Using the above parameters, we calculate that dispersed facies has a total sediment discharge of 1635–3270 m3 a−1. Using the data in Reference Cook, Robinson, Fairchild, Knight, Waller and BoomerCook and others (2010), we calculate that the total sediment discharge for supercool stratified facies is 207–414 m3 a−1 and for regelation stratified facies is 42–84 m3 a−1 (i.e. a total of 249–498 m3 a−1 for stratified facies). Thus, despite its low sediment content, dispersed facies transports ∼6.5 times as much sediment to the proglacial environment as does stratified facies, because of its consistently higher thickness and ubiquity around the glacier margin.
Tectonic processes are typically thought not to be significant for glacier and ice-sheet sediment budgets, except for surging, advancing and polythermal glaciers (e.g. Reference Alley, Cuffey, Evenson, Strasser, Lawson and LarsonAlley and others, 1997). However, our results indicate that thrusting and folding on glacier-thickness scales, generated at the base of an icefall, serve to significantly enhance sediment entrainment and transport even at temperate glaciers, in accordance with other recent work (e.g. Reference Goodsell, Hambrey and GlasserGoodsell and others, 2002; Reference Swift, Evans and FallickSwift and others, 2006). The effect of tectonic processes on the development of basal ice may have been overlooked in previous studies because the basal ice visible at the glacier margin may not show much (or any) clear evidence for tectonic entrainment of sediment. Such evidence would be removed from the basal ice or overprinted by other processes (e.g. regelation, melting, refreezing, water percolation, strain metamorphism, etc.). We suggest, therefore, that tectonic entrainment of material in the deep interior of glaciers and ice sheets may be more widespread than previously envisaged, but that the influence of such processes in the development of basal ice is not observable, nor is there sufficient detail on bed topography beneath many ice masses to be able to identify areas where such processes may operate (i.e. situations similar to an icefall or overdeepening, but beneath several hundred metres or several kilometres of ice).
Conclusions
At Svínafellsjökull we have tested existing hypotheses for the origin of dispersed facies basal ice which is found almost ubiquitously around the glacier margin in thicknesses of up to ∼25 m. These existing theories included regelation around bedrock obstacles with associated flow of sediment-laden water through the intercrystalline vein network, tectonic attenuation of underlying sediment or sediment-rich basal ice, strain-induced metamorphism of firnified glacier ice, basal adfreezing of subglacial water, and inputs from surface ice and sediment sources. Analysis of physical, sedimentological and stable-isotope characteristics of the dispersed facies at Svínafellsjökull reveals that none of these existing hypotheses can fully account for the origin of dispersed facies here. Instead we propose that dispersed facies forms by regelation and strain-induced metamorphism of the lowermost parts of band ogives. These ogives form at the base of a prominent icefall where compression leads to the elevation of basal sediment through the glacier thickness and across the glacier width by folding and thrusting. Minor folds and thrusts at the glacier base between ogive bands may also entrain significant amounts of sediment, and will also be subject to regelation and strain during transport to the glacier terminus. The presence of a terminal over-deepening beneath the glacier may also promote the thickening of dispersed facies by (1) allowing ice flow against a reverse bed slope, thereby enhancing tectonic thickening of the basal ice, and (2) preventing significant basal melt as a consequence of the operation of an inefficient subglacial drainage system.
Dispersed facies is calculated to have a sediment flux of 0.81–1.62 m3 m−1 a−1 (dependent on glacier velocity) which is lower than that measured for the underlying debris-rich stratified facies by Reference Cook, Robinson, Fairchild, Knight, Waller and BoomerCook and others (2010). However, because the dispersed facies is thick (∼3.5 m on average) and almost ubiquitous around the glacier margin, it has a total sediment discharge of 1635–3270 m3 a−1. This value is ∼6.5 times greater than that of the stratified facies. The dispersed facies owes its origin and sediment content to tectonic entrainment of sediment at the base of an icefall. Tectonic processes of sediment entrainment have often been overlooked or considered not to significantly influence the sediment budgets of glaciers and ice sheets. Our results, however, indicate that tectonic processes significantly enhance sediment entrainment and the development of basal ice.
Acknowledgements
We thank I. Fairchild and I. Boomer (University of Birmingham) for isotope analyses. S.J.C. gratefully acknowledges funding from the Royal Geographical Society. D.A.S. acknowledges the support of a BP Royal Society of Edinburgh personal research fellowship and of the Carnegie Trust for the Universities of Scotland. We thank two anonymous reviewers for constructive reviews.