Introduction
Carbonatites are igneous rocks which contain more than 50 modal percent igneous carbonate and less than 20 wt.% silica content (Le Maitre, Reference Le Maitre2002). Mitchell (Reference Mitchell2005, Reference Mitchell2015) has redefined carbonatite as any igneous rock containing more than 30 vol.% primary carbonate, irrespective of its silica content. There are more than 500 carbonatite occurrences in world, ranging in age from Archean to Recent (Woolley and Kjarsgaard, Reference Woolley and Kjarsgaard2008). These commonly occur in the continental crust and are tectonically associated with extensional and post-collisional settings (e.g. Mitchell and Smith, Reference Mitchell and Smith2017; Boukirou et al., Reference Boukirou, Bouabdellah, Chakhmouradian, Mouttaqi, Reguir, Hauff, Cuney, J́ebrak, Yans and Hoernle2022). Calcite, dolomite, ferroan dolomite, magnesian siderite and siderite occur as the dominant carbonate minerals (Mitchell and Gittins, Reference Mitchell and Gittins2022) and can constitute up to 90 vol.% of the rock (Chudy, Reference Chudy2014). Accessory minerals include apatite, amphibole, phlogopite, magnetite, ilmenite, pyrite, baryte, fluorite and strontianite. Carbonatites are derived from mantle-derived magmas (e.g. Wallace and Green, Reference Wallace and Green1988; Bell and Simonetti, Reference Bell and Simonetti2010) and are known to host economic deposits of Nb (Mitchell, Reference Mitchell2015) and rare earth elements (REE) (Chakhmouradian and Zaitsev, Reference Chakhmouradian and Zaitsev2012).
The Newania carbonatite complex is one of the oldest carbonatite occurrences of more than 26 carbonatite localities in the Indian Peninsula. This is the only dolomite carbonatite-dominated occurrence with no associated calcite carbonatite and undersaturated silicate rocks (Viladkar, Reference Viladkar1980; Ray et al., Reference Ray, Pande, Bhutani, Shukla, Rai, Kumar, Awasthi, Smitha and Panda2013; Krishnamurthy, Reference Krishnamurthy2019; Tantkar, Reference Tantkar2019). Subsequent to its first report as Aravalli limestone (Gupta, Reference Gupta1934), followed by identification as a carbonatite (Dar, Reference Dar1964; Deans, unpublished report 1967), various petrologists have investigated the complex, mostly with respect to whole-rock geochemistry (Viladkar, Reference Viladkar1980; Viladkar and Wimmenauer, Reference Viladkar and Wimmenauer1986; Viladkar and Pawaskar, Reference Viladkar and Pawaskar1989; Pandit and Golani, Reference Pandit and Golani2001; Ray et al., Reference Ray, Shukla and Dewangan2010, 2013; Tantkar, Reference Tantkar2019). Mineralogical aspects of the complex have been documented in Viladkar and Wimmenauer (Reference Viladkar and Wimmenauer1986), Buckley and Woolley (Reference Buckley and Woolley1990), Woolley and Buckley (Reference Woolley and Buckley1993), Viladkar and Ghose (Reference Viladkar and Ghose2002), Doroshkevich et al. (Reference Doroshkevich, Ripp and Viladkar2010a), Ray et al. (Reference Ray, Pande, Bhutani, Shukla, Rai, Kumar, Awasthi, Smitha and Panda2013), Viladkar et al. (Reference Viladkar, Bismayer and Zietlow2017) and Sorokhtina et al. (Reference Sorokhtina, Belyatsky, Zaitsev, Viladkar, Kononkova and Ghatak2022). This study illustrates the petrographic and mineral compositional data for the constituent carbonate, silicate, phosphate and oxide minerals of the dolomite carbonatite to understand the magmatic and post-magmatic evolutionary history of the complex. The origin of the Newania carbonatites is briefly discussed as further data such as radiogenic isotopic studies are required.
Geological setting
Regional Geology
The Aravalli Craton is one of the five Precambrian cratons which constitute the Indian peninsula. This craton occupies the north-western region of India and is a part of the Aravalli orogen (Ramakrishnan and Vaidyanandhan, Reference Ramakrishnan and Vaidyanandhan2010). The Banded Gneissic Complex comprises the crystalline basement of the craton and has two subdivisions which lie south and north of Nathdwara, near Udaipur, respectively (Fig. 1a; Gupta, Reference Gupta1934; Heron, Reference Heron1953; Fareeduddin and Banerjee, Reference Fareeduddin and Banerjee2020). The older group occurs south of Nathdwara and is Palaeoarchean-to-early Palaeoproterozoic, forming the basement for Aravalli and Delhi supergroups (Gupta, Reference Gupta1934; Ahmad et al., Reference Ahmad, Mondal, Rahaman, Bhutani and Satyanarayanan2020), whereas the younger group, lying north of Nathdwara is dominantly late Proterozoic in age (~1.7 Ga; Fareeduddin and Kroner, Reference Fareeduddin, Kroner and Paliwal1998; Buick et al., Reference Buick, Allen, Pandit, Rubatto and Hermann2006; Dharma Rao et al., Reference Dharma Rao, Santosh, Purohit, Wang, Jiang and Kusky2011). The older complex is essentially undeformed and consists of granitic, metabasic, metasedimentary and ultrabasic rocks. The granites include orthogneisses and granitoids. The granitoids are younger than orthogneisses and are Neoarchean-to-Palaeoarchean in age. These intrude the older rocks in and around Gingla, Ahar River and Berach (Ahmad et al., Reference Ahmad, Mondal, Rahaman, Bhutani and Satyanarayanan2020). The Untala granite is one such undeformed granitoid body located near Untala (Vallabhnagar, Rajasthan). This is a lens-shaped intrusion which covers an area of ~300 km2 and is composed of grey diorite–tonalite–trondhjemite and pink-to-grey granite, gradational into granodiorite (Roy and Jakhar, Reference Roy and Jakhar2002; Ramakrishnan and Vaidyanandhan, Reference Ramakrishnan and Vaidyanandhan2010; GSI, 2011).

Figure 1. (a) Regional geological setting of the Aravalli Craton (after Heron, Reference Heron1953; Roy and Jakhar, Reference Roy and Jakhar2002); (b) Geological setting of the Newania carbonatite complex (after Tantkar, Reference Tantkar2019).
Local Geology
The Newania carbonatite complex (24°39'2.98“N, 74°03'32.75”E) is located NE of Newania village, ~40 km from Udaipur city in Rajasthan. It is ~3 km in length, forming a NW–SE trending plutonic body which has intruded the Untala granite (Fig. 1b; Viladkar, Reference Viladkar1980; Viladkar and Pawaskar, Reference Viladkar and Pawaskar1989; Ray et al., Reference Ray, Pande, Bhutani, Shukla, Rai, Kumar, Awasthi, Smitha and Panda2013). The complex has been emplaced within structurally weak zones in the Aravalli rift (Viladkar and Wimmenauer, Reference Viladkar and Wimmenauer1986). Small-scale brecciation at the contact between carbonatite and country rock is present (Viladkar, Reference Viladkar1980). Undersaturated alkaline silicate rocks are not present in the complex or in the surrounding areas (Viladkar, Reference Viladkar1980; Viladkar and Wimmenauer, Reference Viladkar and Wimmenauer1986). The complex is surrounded by a fenitised aureole, extending up to a distance of 75 m from the contact between carbonatite and country rock (Viladkar and Wimmenauer, Reference Viladkar and Wimmenauer1986; Viladkar and Pawaskar, Reference Viladkar and Pawaskar1989).
The Newania carbonatite complex is a plutonic dolomite carbonatite with minor ferrocarbonatite, which occurs as bands, dykes and veins in the former. A laterite cap covers the complex. The weathered exposures of dolomite carbonatite are mostly brown whereas fresh outcrops are greyish-white in colour. In hand specimens, these are coarse-to-medium-grained rocks, composed dominantly of carbonate crystals, ranging in size up to 3 mm in diameter. Melanocratic bands composed of apatite, amphibole and magnetite are common. Apatite lenses up to a few cm in length are present. Ferrocarbonatite is dark maroon in colour and is exposed mainly in the north-western region of the complex. Bands and lenses of apatite, ranging from a few millimetres to metres are present in ferrocarbonatite. Laterite exposures are mostly present at the margins of the complex and have a sharp contact with the underlying carbonatite. Fresh exposures of laterite are yellowish brown in colour with visible crystals of mica. Fenite exposures around the complex are obscured by agricultural fields. A few exposures can be seen within the north-western region of the complex. These are fine-to-coarse, green-to-off-white, massive-to-banded rocks in which large crystals of amphibole, mica and feldspar can be recognised.
The Newania carbonatite complex is Proterozoic in age, although various ages have been determined by different investigations. The earliest attempts by Deans and Powell (Reference Deans and Powell1968) gave a K–Ar age of 959 Ma for alkali amphibole in fenite. Schleicher et al. (Reference Schleicher, Todt, Viladkar and Schmidt1997) and Sorokhtina et al. (Reference Sorokhtina, Belyatsky, Zaitsev, Viladkar, Kononkova and Ghatak2022) have reported the oldest emplacement ages of ~2.1–2.3 Ga for the dolomite carbonatite. Ankerite carbonatite, here termed ferrocarbonatite, is considered to have an age between ~1.4 to 1.5 Ga (Schleicher et al., Reference Schleicher, Todt, Viladkar and Schmidt1997; Ray et al., Reference Ray, Pande, Bhutani, Shukla, Rai, Kumar, Awasthi, Smitha and Panda2013). Younger ages ranging between 900 and 950 Ma are reported by Gruau et al. (Reference Gruau, Petibon, Viladkar, Fourcade, Bernard-Griffiths and Mace1995) and Ray et al. (Reference Ray, Pande, Bhutani, Shukla, Rai, Kumar, Awasthi, Smitha and Panda2013) and are considered to represent a high-temperature metamorphic event.
Analytical techniques
Petrographic studies of polished sections of dolomite carbonatite were carried out using an Olympus CX31 optical microscope at Panjab University, Chandigarh and Hitachi S-3400 N Scanning Electron Microscope at the CSIR-National Geophysical Research Institute, Hyderabad, India. Compositions of carbonate, silicate, phosphate and oxide minerals were determined using a five-channel CAMECA SX-5 electron probe micro-analyser (EPMA), installed at the Department of Earth Sciences, Indian Institute of Technology, Bombay. Details of the calibration settings of the instrument and standards used are given in Supplementary Table S1. The average lower limit of detection (LLD) for analysed elements is given in the tables in the Mineral composition section.
Petrography
Dolomite carbonatite is a coarse-to-fine-grained, equigranular rock, consisting dominantly of ferroan dolomite. Accessory minerals include apatite, amphibole, magnetite, graphite, monazite, pyrochlore and columbite which display intra-intrusion modal variations (Fig. 2a–h). Ferroan dolomite occurs as coarse-to-medium-grained subhedral crystals with a mosaic texture (Fig. 2a). Signatures of deformation are evident which include well-defined triple-junctions and thick twin lamellae. Grain boundaries are serrated and show bulging and creep (Fig. 2c,f,g). Magnesian siderite occurs as fine-to-medium-grained subhedral crystals, found at interstices of ferroan dolomite grains. These are found in textural equilibrium with apatite (Fig. 2e).

Figure 2. Photomicrographs of dolomite carbonatite in the Newania carbonatite complex, illustrating (a) crossed polars (xpl), ferroan dolomite set in mosaic fabric with triple junctions; (b) xpl, Ap-1 band and Mnz-1 crystals at grain boundaries, penetrating into the former; fractures and wavy extinction are visible; (c,d) xpl and back-scattered electron (BSE) image, respectively, textural relationships among ferroan dolomite, apatite and monazite; carbonate crystals show evidence of deformation; (e) BSE image, magnesian siderite in cluster with Ap-2 showing vein-like network; (f) plane polarised light (ppl), Amp-1 crystals at carbonate interstices; (g) ppl, single Amp-2 grain at interstices of ferroan dolomite; (h) BSE image, columbite–pyrochlore composite grain with baryte at boundary. Yellow arrows in (a) and (c) indicate signatures of deformation. (Abbreviations – Amp: amphibole, Ap: apatite, Brt: baryte, Clb: columbite, FDol: ferroan dolomite, Mg-Sd: magnesian siderite, Mnz: monazite, Pcl: pyrochlore).
Apatite is the most abundant non-carbonate phase, occurring as fine-to-medium-grained, subhedral-to-anhedral, finely fractured crystals. Two textural varieties of apatite are recognised, designated as Ap-1 and Ap-2. Variety Ap-1 occurs in monominerallic layers of equant crystals with triple junctions and mosaic texture (Fig. 2b). The apatite grains at boundaries of such layers have abraded margins. Variety Ap-2 occurs as discrete crystals or clusters of elliptical/pill-shaped crystals (Fig. 2c–e). Monazite is typically associated with apatite, occurring as fine-grained, subhedral-to-anhedral crystals, commonly penetrating the apatite grains. Two textural varieties of monazite are present, designated as Mnz-1 and Mnz-2. Variety Mnz-1 is associated with Ap-1 as subhedral-to-anhedral inclusions within apatite bands (Fig. 2b). These also occur as aggregates of >3 elliptical crystals, in direct contact with apatite. Mnz-2 occurs as discrete crystals, associated with Ap-2 (Fig. 2d). These are porous and have similar crystal habit as Ap-2. Patchy zoning is evident in a few monazite crystals. These are anhedral coarse-grained (up to 1000 μm in diameter) crystals. High and low AZ (average atomic number) regions are difficult to demarcate in back-scattered electron images; however, false-colour X-ray element-distribution maps show a core, enveloped with an intermediate zone and outermost rim (Fig. 3a–d). Both Mnz-1 and Mnz-2 are exclusively associated with Ap-1 and Ap-2, respectively.

Figure 3. False colour X-ray element-distribution map showing variation in (a) La; (b) Ce; (c) Nd; and (d) Sm contents in a zoned monazite in dolomite carbonatite.
Two amphibole types have been identified and designated as Amp-1 and Amp-2. Type Amp-1 occurs as medium-to-fine-grained, prismatic crystals generally confined to clusters showing pleochroism from greenish blue-to-light lavender blue-to-light bluish grey (Fig. 2f). The Amp-2 type forms fine-grained prismatic-to-bladed individual crystals and is pleochroic from light brown-to-nearly colourless (Fig. 2g). Both amphibole populations are present at carbonate interstices and are an early crystallised phase relative to the surrounding carbonates. Very fine-grained (>50 μm) inclusions of graphite are present within amphibole, apatite and ferroan dolomite grains.
Columbite and pyrochlore are the two Nb-bearing phases present. They have a wide variation in modal abundance, occasionally reaching up to 5 vol.%. Two textural varieties of columbite are identified: (1) Clb-1, which forms fine-grained (up to 150 μm), subhedral crystals with oval margins; intergrown with pyrochlore, forming composite grains; baryte is also present at crystal margins; and (2) Clb-2, occurring as fine-grained (up to 30 μm) subhedral-to-euhedral crystals (Fig. 2h). Both columbite varieties are commonly found at ferroan dolomite interstices. Magnetite are rare fine-grained euhedral-to-subhedral crystals, occurring as either individual grains or in layers with amphiboles.
Mineral compositions
Carbonates
Representative compositions of carbonates are given in Table 1. These are ferroan dolomite, containing 10.7–12.8 wt.% FeO, 13.9–15.4 wt.% MgO and 27.4–29.8 wt.% CaO. The contents of MnO and SrO vary up to 0.9 wt.%. These compositions partially overlap with carbonate compositions determined in earlier studies of the Newania carbonatite (Fig. 4a–c; Viladkar and Wimmenauer, Reference Viladkar and Wimmenauer1986; Doroshkevich et al., Reference Doroshkevich, Ripp and Viladkar2010a; Ray et al., Reference Ray, Pande, Bhutani, Shukla, Rai, Kumar, Awasthi, Smitha and Panda2013). They display compositional evolution by increasing Fe and decreasing Mg contents, in common with magmatic dolomite-to-ferroan dolomite in other carbonatite complexes (Fig. 4a–c; Viladkar and Subramanian, Reference Viladkar and Subramanian1995; Doroshkevich et al., Reference Doroshkevich, Wall and Ripp2007, Reference Doroshkevich, Ripp and Moore2010b; Chakhmouradian et al., Reference Chakhmouradian, Böhm, Demény, Reguir, Hegner, Creaser, Halden and Yang2009, Reference Chakhmouradian, Reguir and Zaitsev2016; Mitchell and Smith, Reference Mitchell and Smith2017; Rampilova et al., Reference Rampilova, Doroshkevich, Viladkar and Zubakova2021; Boukirou et al., Reference Boukirou, Bouabdellah, Chakhmouradian, Mouttaqi, Reguir, Hauff, Cuney, J́ebrak, Yans and Hoernle2022).
Table 1. Compositions of carbonates in dolomite carbonatite.

LLD – lower limit of detection; bdl – below detection limit.

Figure 4. Compositional variation of carbonates in the Newania carbonatites. (a) Ca-Mg-Fe plot (after Mitchell and Smith, Reference Mitchell and Smith2017); (b) Mg/Fe vs. Ca (apfu); (c) Mn vs. Fe (apfu). Grey field: dolomite–ferroan dolomite compositions in global carbonatites; yellow field: magnesite–siderite carbonate compositions in global carbonatites; data for compositional fields of carbonates are taken from Viladkar and Subramanian (Reference Viladkar and Subramanian1995), Doroshkevich et al. (Reference Doroshkevich, Wall and Ripp2007, Reference Doroshkevich, Ripp and Moore2010b), Chakhmouradian et al. (Reference Chakhmouradian, Böhm, Demény, Reguir, Hegner, Creaser, Halden and Yang2009, Reference Chakhmouradian, Reguir and Zaitsev2016), Mitchell and Smith (Reference Mitchell and Smith2017), Rampilova et al. (Reference Rampilova, Doroshkevich, Viladkar and Zubakova2021), Boukirou et al. (Reference Boukirou, Bouabdellah, Chakhmouradian, Mouttaqi, Reguir, Hauff, Cuney, J́ebrak, Yans and Hoernle2022); previously published data for Newania carbonates are from Viladkar and Wimmenauer (Reference Viladkar and Wimmenauer1986), Buckley and Woolley (Reference Buckley and Woolley1990), Woolley and Buckley (Reference Woolley and Buckley1993), Doroshkevich et al. (Reference Doroshkevich, Ripp and Viladkar2010a), Ray et al. (Reference Ray, Pande, Bhutani, Shukla, Rai, Kumar, Awasthi, Smitha and Panda2013).
Magnesian siderite displays restricted compositions in comparison with previously reported compositions from Newania carbonatites (Fig. 4a–c; Buckley and Woolley, Reference Buckley and Woolley1990; Woolley and Buckley, Reference Woolley and Buckley1993; Doroshkevich et al., Reference Doroshkevich, Ripp and Viladkar2010a; Ray et al., Reference Ray, Pande, Bhutani, Shukla, Rai, Kumar, Awasthi, Smitha and Panda2013). These are characterised by 34.5–35.1 wt.% FeO, 19.2–19.5 wt.% MgO, 1.5–1.6 wt.% MnO and 0.8–0.9 wt.% CaO contents. These magnesian siderite fall well within the compositional ranges of magnesite–siderite solid solutions found in the Gleibat Lafhouda carbonatite, Morocco (Boukirou et al., Reference Boukirou, Bouabdellah, Chakhmouradian, Mouttaqi, Reguir, Hauff, Cuney, J́ebrak, Yans and Hoernle2022) and Ashram Zone carbonatite, Québec, Canada (Mitchell and Smith, Reference Mitchell and Smith2017; Fig. 4a–c).
Apatite
Compositions of Newania apatite are given in Table 2 which shows that they are fluorapatite, containing >3.2 wt.% F. Compositional zoning is absent. Ap-1 contains lower Sr (0.4–0.8 wt.% SrO) and light REE (0.2–1.0 wt.% ΣLREE2O3) contents than Ap-2 (1.3–2.0 wt.% SrO; 0.9–1.5 wt.% ΣLREE2O3). The contents of Na, Si and Fe are low (<0.4 wt.%). Newania apatite display compositional evolution from Ap-1 to Ap-2 indicated by Sr, Na and LREE enrichment and simultaneous Ca depletion (Fig. 5a,b).
Table 2. Compositions of Newania apatite.

FeO* – total iron expressed as FeO; Fe2+* – total iron as Fe2+; LLD – lower limit of detection; bdl – below detection limit.

Figure 5. Compositional variation of apatite (Ap-1 and Ap-2) from the Newania carbonatite complex: (a) Si4+ + REE3+ vs. Ca2+ + P5+ (apfu); (b) Na+ + REE3+ vs. Ca2+ (apfu); (c) Sr2+ vs. Ca2+ (apfu); and (d) Na+ vs. REE3+ (apfu). Data for carbonatitic apatite are from Viladkar and Wimmenauer (Reference Viladkar and Wimmenauer1992), Viladkar and Subramanian (Reference Viladkar and Subramanian1995), Doroshkevich et al. (Reference Doroshkevich, Wall and Ripp2007, 2010b), Melluso et al. (Reference Melluso, Srivastava, Guarino, Zanetti and Sinha2010), Burtseva et al. (Reference Burtseva, Ripp, Doroshkevich, Viladkar and Varadan2013), Sadiq et al. (Reference Sadiq, Ranjith and Umrao2014), Guarino et al. (Reference Guarino, Wu, Melluso, de Barros Gomes, Tassinari, Ruberti and Brilli2017); Fosu et al. (Reference Fosu, Ghosh, Chew and Viladkar2019), Rampilova et al. (Reference Rampilova, Doroshkevich, Viladkar and Zubakova2021), Boukirou et al. (Reference Boukirou, Bouabdellah, Chakhmouradian, Mouttaqi, Reguir, Hauff, Cuney, J́ebrak, Yans and Hoernle2022); previously published apatite compositions for Newania are from Viladkar and Wimmenauer (Reference Viladkar and Wimmenauer1986), Doroshkevich et al. (Reference Doroshkevich, Ripp and Viladkar2010a), Ray et al. (Reference Ray, Pande, Bhutani, Shukla, Rai, Kumar, Awasthi, Smitha and Panda2013).
Three major substitution mechanisms are evident in Newania apatite: (1) Si4+ + REE3+↔Ca2+ + P5+; (2) Na+ + REE3+↔2Ca2+; and (3) Sr2+ ↔ Ca2+ (Fig. 5a–c). Substitution mechanism (1) or britholite-type is shown by both Ap-1 and Ap-2 (Fig. 5a). Substitution mechanism (2) or belovite-type substitution is more pronounced in Ap-2 than Ap-1 apatite (Fig. 5b). These apatites display a positive correlation of 1:1 between Na and REE (Fig. 5d). Substitution of Ca for Sr varies from 1:1 to 2:1 ratio for Ap-1 to Ap-2 (Fig. 5c). Previously published data for Newania apatite (Viladkar and Wimmenauer, Reference Viladkar and Wimmenauer1986; Doroshkevich et al., Reference Doroshkevich, Ripp and Viladkar2010a; Ray et al., Reference Ray, Pande, Bhutani, Shukla, Rai, Kumar, Awasthi, Smitha and Panda2013) are similar to Ap-2 and display similar substitution trends whereas Ap-1 compositions have not been reported previously (Fig. 5a–d). The substitution mechanisms shown by Newania apatite are similar to those of other carbonatite apatites (Fig. 5a–c; Viladkar and Wimmenauer, Reference Viladkar and Wimmenauer1992; Viladkar and Subramanian, Reference Viladkar and Subramanian1995; Doroshkevich et al., Reference Doroshkevich, Wall and Ripp2007, Reference Doroshkevich, Ripp and Moore2010b; Melluso et al., Reference Melluso, Srivastava, Guarino, Zanetti and Sinha2010; Burtseva et al., Reference Burtseva, Ripp, Doroshkevich, Viladkar and Varadan2013; Sadiq et al., Reference Sadiq, Ranjith and Umrao2014; Guarino et al., Reference Guarino, Wu, Melluso, de Barros Gomes, Tassinari, Ruberti and Brilli2017; Fosu et al., Reference Fosu, Ghosh, Chew and Viladkar2019; Rampilova et al., Reference Rampilova, Doroshkevich, Viladkar and Zubakova2021; Boukirou et al., Reference Boukirou, Bouabdellah, Chakhmouradian, Mouttaqi, Reguir, Hauff, Cuney, J́ebrak, Yans and Hoernle2022).
Monazite
The compositions of zonation-free and zoned monazite are given in Table 3. The two textural varieties (Mnz-1 and Mnz-2) show significant compositional contrast in LREE (La, Pr, Nd and Sm) contents except for Ce which is the dominant REE in both the varieties, ranging from 31.3 to 35.4 wt.% Ce2O3 (Fig. 6a,b). Mnz-1 are characterised by lower La (13.6–17.4 wt.% La2O3) and higher Pr (3.8–4.5 wt.% Pr2O3), Nd (11.0–15.4 wt.% Nd2O3) and Sm (1.1–2.2 wt.% Sm2O3) contents as compared to Mnz-2 (24.3–25.4 wt.% La2O3; 2.6–3.0 wt.% Pr2O3; 6.6–7.9 wt.% Nd2O3; 0.4–0.5 wt.% Sm2O3). Uranium is below the detection limit and Th content reaches up to 1.2 wt.% in Mnz-2.
Table 3. Compositions of Newania monazite.

LLD – lower limit of detection; bdl – below detection limit.
* Zoned samples: 11,12 = core; 13–18 = intermediate rim; 19 = outermost rim

Figure 6. Compositional variation of monazite (Mnz-1 and Mnz-2) from Newania carbonatites: (a) Nd2O3–La2O3–Ce2O3 ternary plot (wt.%); (b) Ce2O3 vs. La2O3 (wt.%); (c) (Th+U)4+ + Si4+ vs. ΣREE3+ + P5+(apfu); (d) (Th+U)4+ + Ca2+ vs. ΣREE3+ (apfu). Compositional fields of carbonatite-hosted monazites in Fig. 6a are shown in grey colour and are from Chen et al. (Reference Chen, Honghui, Bai and Jiang2017). The data points of worldwide carbonatite monazites in Fig. 6b–d are from Doroshkevich et al. (Reference Doroshkevich, Wall and Ripp2007), Burtseva et al. (Reference Burtseva, Ripp, Doroshkevich, Viladkar and Varadan2013), Basu and Bhattacharya (Reference Basu and Bhattacharyya2014), Sadiq et al. (Reference Sadiq, Ranjith and Umrao2014), Dalsin et al. (Reference Dalsin, Groat, Creighton and Evans2015), Trofanenko et al. (Reference Trofanenko, Williams-Jones, Simandl and Migdisov2016), Rampilova et al. (Reference Rampilova, Doroshkevich, Viladkar and Zubakova2021); previously published Newania monazite composition are from Sorokhtina et al. (Reference Sorokhtina, Belyatsky, Zaitsev, Viladkar, Kononkova and Ghatak2022).
False colour X-ray elemental maps of zoned monazites show a La–Ce-rich core, enveloped by a discontinuous La–Ce-poor and Nd-rich intermediate zone; an outermost partial rim compositionally resembling the core is also present (Fig. 3). Compositionally, these overlap with unzoned Mnz-1. The core contains lower La (14.9–15.0 wt.% La2O3) and Ce (31.9–32.2 wt.% Ce2O3) and higher Pr (4.2–4.3 wt.% Pr2O3), Nd (15.6–15.8 wt.% Nd2O3) and Sm (1.8–2.1 wt.% Sm2O3) than intermediate zone (16.5–17.3 wt.% La2O3, 33.1–34.2 wt.% Ce2O3, 3.8–4.2 wt.% Pr2O3, 12.5–13.7 wt.% Nd2O3 and 1.3–1.5 wt.% Sm2O3) and outermost rim (17.2 wt.% La2O3, 33.1 wt.% Ce2O3, 4.2 wt.% Pr2O3, 13.4 wt.% Nd2O3 and 1.4 wt.% Sm2O3).
Newania monazite fall well within the compositional field of worldwide carbonatite monazites (Fig. 6a; Chen et al., Reference Chen, Honghui, Bai and Jiang2017) and are classified as monazite-(Ce). Compositional trends of La enrichment and depletion in Pr, Nd and Sm contents are evident from Mnz-1 to Mnz-2. Cerium displays two distinct compositional evolutionary trends where Ce content increases in Mnz-1 and decreases in Mnz-2 as La content increases (Fig. 6b). The two substitution mechanisms shown by Newania monazite are huttonite {(Th,U)4+ + Si4+ ↔ ΣREE3+ + P5+} and brabantite, now called cheralite {(Th,U)4+ + Ca2+ ↔ 2ΣREE3+}; the former being shown by Mnz-1 with ΣREE3+ + P5+ > 1.994 atoms per formula unit (apfu), whereas the latter substitution is shown by Mnz-2 and Mnz-1 with ΣREE3+ + P5+ < 1.994 apfu (Fig. 6c–d). Similar composition and substitution trends are displayed by other carbonatite monazites (Fig. 6b–d; Burtseva et al., Reference Burtseva, Ripp, Doroshkevich, Viladkar and Varadan2013; Basu and Bhattacharya, Reference Basu and Bhattacharyya2014; Sadiq et al., Reference Sadiq, Ranjith and Umrao2014; Dalsin et al., Reference Dalsin, Groat, Creighton and Evans2015; Trofanenko et al., Reference Trofanenko, Williams-Jones, Simandl and Migdisov2016; Chen et al., Reference Chen, Honghui, Bai and Jiang2017 and references cited therein; Rampilova et al., Reference Rampilova, Doroshkevich, Viladkar and Zubakova2021).
Amphibole
Both types of Newania amphibole display restricted compositional variation (Table 4). Amp-1 is characterised by 12.2–12.7 wt.% total FeO, 15.8–16.6 wt.% MgO and 4.1–5.1 wt.% CaO contents. The contents of Na and K range from 5.3–6.3 wt.% and 0.5–0.6 wt.%, respectively. These contain up to 0.4 wt.% Al2O3, 0.1 wt.% TiO2 and 0.2 wt.% MnO. Compositionally, Amp-1 belongs to the sodium-calcium subgroup of the amphibole supergroup and is ferri-winchite (Fig. 7a). Amp-2 contains 26.3–26.4 wt.% total FeO, 14.5–14.6 wt.% MgO and 0.8–1.1 wt. % Na2O contents. The content of Ca, Mn and F varies from 0.4 to 0.6 wt.%. Potassium, Al and Ti contents reach up to 0.1 wt.%. Amp-2 belongs to the magnesium-iron-manganese subgroup amphiboles and is compositionally equivalent to cummingtonite (Fig. 7b).
Table 4. Compositions of amphiboles in dolomite carbonatite, calculated using the amphibole classification spreadsheet of Locock (Reference Locock2014).

Fe2O3 and FeO calculated on stoichiometric basis; n.a. – not analysed; LLD – lower limit of detection; bdl – below detection limit.

Figure 7. (a,b) Classification diagram for amphiboles in the Newania carbonatites (after Hawthorne et al., Reference Hawthorne, Oberti, Harlow, Maresch, Martin, Schumacher and Welch2012).
Previously published amphibole compositions of Newania carbonatites when recalculated using the spreadsheet of Locock (Reference Locock2014) suggest the presence of magnesio-arfvedsonite, magnesio-riebeckite and ferri-katophorite (Viladkar and Wimmenauer, Reference Viladkar and Wimmenauer1986; Doroshkevich et al., Reference Doroshkevich, Ripp and Viladkar2010a; Ray et al., Reference Ray, Pande, Bhutani, Shukla, Rai, Kumar, Awasthi, Smitha and Panda2013). The compositions display an evolutionary trend from ferri-katophorite to magnesio-arfvedsonite and magnesio-riebeckite, which is similar to the evolution of other carbonatite amphiboles (Fig. 8a; Samoylov, Reference Samoylov1977; Martin, Reference Martin, Hawthorne, Oberti, Della Ventura and Mottana2007; Reguir et al., Reference Reguir, Chakhmouradian, Pisiak, Halden, Yang, Xu, Kynický and Couëslan2012). The amphibole compositions obtained in this investigation differ markedly from the earlier published compositions (Fig. 8a,b), although similar amphiboles have been reported previously from a few carbonatite occurrences i.e. Veseloe, North Transbaikalia, Russia (Doroshkevich et al., Reference Doroshkevich, Wall and Ripp2007), Fir, British Columbia, Canada (Chudy, Reference Chudy2014) and Huayangchuan carbonatites, Central China (Reguir et al., Reference Reguir, Chakhmouradian, Pisiak, Halden, Yang, Xu, Kynický and Couëslan2012; Fig. 8a–c).

Figure 8. Compositional variation of amphiboles in dolomite carbonatite: (a) Total Fe vs. Total Mg (apfu); (b) Total Na + K vs. Total Ca (apfu); (c) Total Ti vs. Total Al (apfu). Data for worldwide carbonatitic amphiboles are taken from Secher and Larsen (Reference Secher and Larsen1980), Viladkar and Subramanian (Reference Viladkar and Subramanian1995), Doroshkevich et al. (Reference Doroshkevich, Wall and Ripp2007, Reference Doroshkevich, Ripp and Moore2010b), Reguir et al. (Reference Reguir, Chakhmouradian, Pisiak, Halden, Yang, Xu, Kynický and Couëslan2012), Chudy (Reference Chudy2014), Rampilova et al. (Reference Rampilova, Doroshkevich, Viladkar and Zubakova2021); previously published data for Newania amphiboles are taken from Viladkar and Wimmenauer (Reference Viladkar and Wimmenauer1986); Doroshkevich et al. (Reference Doroshkevich, Ripp and Viladkar2010a) and Ray et al. (Reference Ray, Pande, Bhutani, Shukla, Rai, Kumar, Awasthi, Smitha and Panda2013).
Columbite
The compositions of columbite are given in Table 5. Clb-1 contains 67.9–77.3 wt.% Nb2O5, 1.6–3.4 wt.% Ta2O5, 7.7–18.7 wt.% FeO and 1.0–4.1 wt.% TiO2. Clb-2 is characterised by similar Nb (68.4–73.9 wt.% Nb2O5), higher Ta (4.5–10.0 wt.% Ta2O5) and Fe (18.4–19.1 wt.% FeO) and lower Ti (0.3–0.8 wt.% TiO2) contents than Clb-1. The manganese content is below the detection limit (800 ppm). Compositionally, Clb-1 and Clb-2 are iron-rich members of the columbite supergroup i.e. columbite-(Fe) (Chukanov et al., Reference Chukanov, Pasero, Aksenov, Britvin, Zubkova, Yike and Witzke2023). Previously published columbite compositions from Newania carbonatites resemble those of Clb-2 (Fig. 9; Viladkar et al., Reference Viladkar, Bismayer and Zietlow2017). An increase in Ta content from Clb-1 to Clb-2 is similar to the compositional evolution of columbite from Fir carbonatites (Chudy, Reference Chudy2014). Overall, the compositions of Newania columbite-(Fe) are similar to those found in Fir (Chudy, Reference Chudy2014) and Gleibat Lafhouda (Boukirou et al., Reference Boukirou, Bouabdellah, Chakhmouradian, Mouttaqi, Reguir, Hauff, Cuney, J́ebrak, Yans and Hoernle2022) whereas those from Aley, British Columbia, Canada (Chakhmouradian et al., Reference Chakhmouradian, Reguir, Kressall, Crozier, Pisiak, Sidhu and Yang2015) and Ashram (Mitchell and Smith, Reference Mitchell and Smith2017) are relatively depleted in Ta (Fig. 9).
Table 5. Compositions of columbite-(Fe) in dolomite carbonatite.
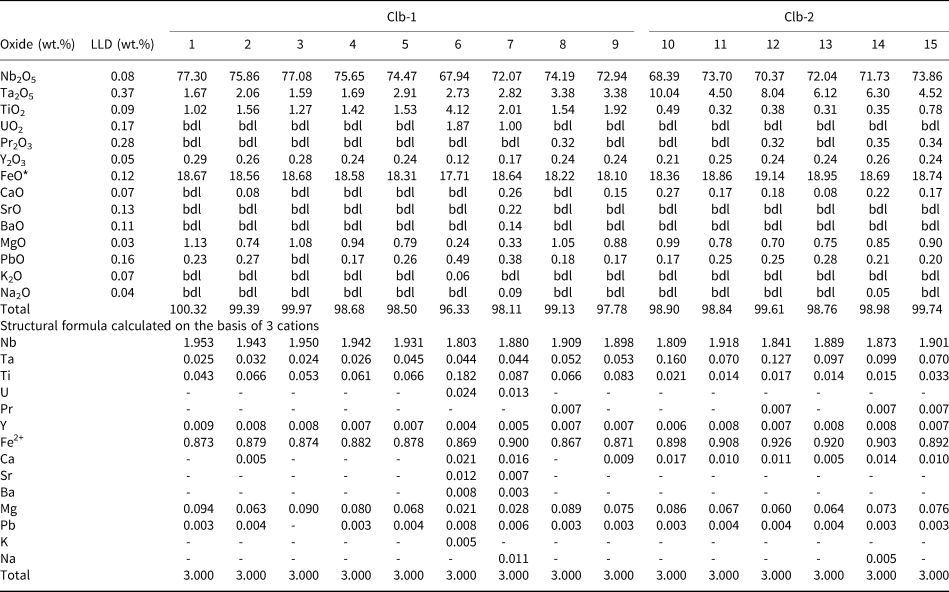
FeO* is total iron oxide; LLD – lower limit of detection; bdl – below detection limit.

Figure 9. Nb2O5–Ta2O5–TiO2 ternary diagram showing variation in the compositions of columbite-(Fe) in the Newania carbonatites. Data for columbite in worldwide carbonatites are from Chudy (Reference Chudy2014), Chakhmouradian et al. (Reference Chakhmouradian, Reguir, Kressall, Crozier, Pisiak, Sidhu and Yang2015), Mitchell and Smith (Reference Mitchell and Smith2017) and Boukirou et al. (Reference Boukirou, Bouabdellah, Chakhmouradian, Mouttaqi, Reguir, Hauff, Cuney, J́ebrak, Yans and Hoernle2022); previously published data for Newania columbite-(Fe) are from Viladkar et al. (Reference Viladkar, Bismayer and Zietlow2017).
Pyrochlore
The compositions of pyrochlore in the dolomite carbonatite are given in Table 6. The nomenclature used for the pyrochlore supergroup follows Atencio et al. (Reference Atencio, Andrade, Christy, Gieré and Kartashov2010) and Bhattacharjee et al. (Reference Bhattacharjee, Dey, Chakrabarty, Mitchell and Ren2022). The Newania pyrochlores are enriched in niobium with Nb>Ti>>>Ta, and hence, belong to the pyrochlore-group (Fig. 10a) and are Sr-bearing U-rich kenocalciopyrochlore and Sr-, Ca-bearing U-rich kenopyrochlore. They are characterised by 26.0–28.5 wt.% Nb2O5, 12.9–16.0 wt.% Ta2O5 and 5.3–6.8 wt.% TiO2. A-site cations are dominated by Ca (3.9–4.6 wt.% CaO) and U (17.1–19.6 wt.% UO2). Strontium and Ba contents range up to 4.7 wt.% and 0.9 wt.%, respectively. The contents of Na and REE are <0.5 wt.%.
Table 6. Compositions of pyrochlore in dolomite carbonatite.

Fe2O3# is total iron, calculated from FeO; lower limit of detection for FeO is 0.12 wt. %; LLD – lower limit of detection; bdl – below detection limit.

Figure 10. Ternary compositional diagrams for pyrochlores in dolomite carbonatite at Newania: (a) Nb–Ti–Ta (apfu); (b) Ca – A-site-vacancy – Na (apfu); (c) Na+Ca – A-site-vacancy – U+Th (apfu); (d) Na+Ca – Sr+Ba – F (apfu). Fields in (a) are from Atencio et al. (Reference Atencio, Andrade, Christy, Gieré and Kartashov2010), dotted-line and yellow fields in (b) are from Zurevinski and Mitchell (Reference Zurevinski and Mitchell2004) and Bhattacharjee et al. (Reference Bhattacharjee, Dey, Chakrabarty, Mitchell and Ren2022), respectively and grey field in (c) is taken from Chakhmouradian and Mitchell (Reference Chakhmouradian and Mitchell2002); pyrochlore compositional data are taken from Chakhmouradian and Mitchell (Reference Chakhmouradian and Mitchell1998), Bambi et al. (Reference Bambi, Costanzo, Gonçalves and Melgarejo2012), Chudy (Reference Chudy2014), Viladkar and Bismayer (Reference Viladkar and Bismayer2014), Chakhmouradian et al. (Reference Chakhmouradian, Reguir, Kressall, Crozier, Pisiak, Sidhu and Yang2015) and Mitchell et al. (Reference Mitchell, Wahl and Cohen2020); previously published data for Newania pyrochlores are taken from Viladkar and Ghose (Reference Viladkar and Ghose2002) and Viladkar et al. (Reference Viladkar, Bismayer and Zietlow2017).
Pyrochlore in the dolomite carbonatite fall within the hydrothermal to supergene field on a ternary Na – A-site-vacancy – Ca plot and partially overlap with previously published Newania pyrochlore compositions (Fig. 10b; Viladkar and Ghose, Reference Viladkar and Ghose2002; Viladkar et al., Reference Viladkar, Bismayer and Zietlow2017). Overall, the Newania pyrochlore compositions are in good agreement with the carbonatite pyrochlore compositions, worldwide (Fig. 10c). Similar pyrochlore compositions with significant A-site vacancy, Sr, Ba and U are described from carbonatites at Angola (Bambi et al., Reference Bambi, Costanzo, Gonçalves and Melgarejo2012), Lesnaya Varaka, Kola Peninsula, Russia (Chakhmouradian and Mitchell, Reference Chakhmouradian and Mitchell1998), Good Hope, Canada (Mitchell et al., Reference Mitchell, Wahl and Cohen2020), Catalão I and II, Brazil (Guarino et al., Reference Guarino, Wu, Melluso, de Barros Gomes, Tassinari, Ruberti and Brilli2017) and Sevathur, Tamil Nadu, India (Viladkar and Bismayer, Reference Viladkar and Bismayer2014; Dey et al., Reference Dey, Bhattacharjee, Chakrabarty, Mitchell, Pal, Pal and Sen2021; Fig. 10d). Newania pyrochlores analysed in this work and previous studies, together with those from Sevathur and Lesnaya Varaka show an evolutionary trend towards increased Sr and Ba. This is in contrast with the magmatic pyrochlores from Aley (Chakhmouradian et al., Reference Chakhmouradian, Reguir, Kressall, Crozier, Pisiak, Sidhu and Yang2015), Angolan (Bambi et al., Reference Bambi, Costanzo, Gonçalves and Melgarejo2012), Catalão I and II (Guarino et al., Reference Guarino, Wu, Melluso, de Barros Gomes, Tassinari, Ruberti and Brilli2017) and Fir (Chudy, Reference Chudy2014) carbonatites which trend towards F (Fig. 10d).
Discussion
Carbonatites are readily susceptible to textural and chemical re-equilibration during subsolidus, deformational and late-stage hydrothermal conditions (e.g. Chudy, Reference Chudy2014; Chakhmouradian et al., Reference Chakhmouradian, Reguir and Zaitsev2016; Mitchell and Smith, Reference Mitchell and Smith2017). The Newania carbonatite complex presents a similar example where primary textures and mineral compositions are modified and new minerals are crystallised due to post-magmatic processes.
Textural modification
Ferroan dolomite is the principal constituent of the dolomite carbonatite at Newania and illustrates variable degrees of textural re-equilibration. Variation of textures from fractured, euhedral crystals to serrated grain boundaries and thick twinning lamellae to grain boundary bulging, creep and re-crystallisation of very fine carbonates at crystal boundaries attest to the inhomogeneous deformational history of the complex. The conditions of deformation range from brittle to ductile deformation which is accompanied by re-crystallisation at relatively high temperatures (Chakhmouradian et al., Reference Chakhmouradian, Reguir and Zaitsev2016). Chudy (Reference Chudy2014) has investigated the fabric of Fir carbonatites at megascopic and microscopic levels, and suggested that the observed textures are due to variable degrees of deformation, varying from brittle to plastic–ductile regimes.
Rarely, apatite in dolomite carbonatite displays effects of deformation, such as fractures in Ap-1 and wavy extinction in Ap-2 crystals. However, magmatic textures of apatite are preserved better than those of carbonates which have been modified post-crystallisation. Magmatic textures of apatite include the oval morphology of the crystals and abraded margins at the contact between apatite and ferroan dolomite grains. These are typical of apatite in plutonic carbonatites, produced via erosion/abrasion due to surrounding magma and crystals and/or by unusual growth mechanisms (Chakhmouradian et al., Reference Chakhmouradian, Reguir, Zaitsev, Couëslan, Xu, Kynický, Hamid Mumin and Yang2017). Such apatite morphologies are significantly different from experimentally produced prismatic apatite with well developed crystal faces (Hammouda et al., Reference Hammouda, Chantel and Devidal2010; Anenburg et al., Reference Anenburg, Mavrogenes, Frigo and Wall2020). The monominerallic layers of Ap-1 grains are magmatic in origin, formed by the separation of apatite grains from parent magma due to crystal setting (Wyllie and Biggar, Reference Wyllie and Biggar1966).
The textural characteristics of Newania monazite are more similar to hydrothermal monazite than magmatic carbonatite monazites, such as having localised aggregates of more than four grains, high relief and birefringence and weak pleochroism (Schandl and Gorton, Reference Schandl and Gorton2004; Kim et al., Reference Kim, Lee, Yin and Park2005; Xu et al., Reference Xu, Kynicky, Chakhmouradian, Campbell and Allen2010; Giebel et al., Reference Giebel, Gauert, Marks, Costin and Markl2017; Slezak and Spandler, Reference Slezak and Spandler2019). The dissolution of precursor mineral(s) and re-precipitation of new or altered phases result in porosity development. The mechanism of porosity development is discussed in Harlov et al. (Reference Harlov, Andersson, Förster, Nyström, Dulski and Broman2002), Putnis (Reference Putnis2002) and Harlov (Reference Harlov2011, Reference Harlov2015). Porous textures are evident in monazite in Newania carbonatite and indicate a non-magmatic origin. Inhomogeneous intra-intrusion distribution and exclusive occurrence with apatite as anhedral inclusions at grain boundaries of Ap-1, commonly penetrating the grains or as pseudomorphs after the Ap-2 attest to the post-magmatic origin of monazite. The presence of hydrothermal monazite (Mnz-1) with deformed Ap-1 grains indicates the involvement of a fluid phase during or after the deformation. Experimental studies have shown that monazite can crystallise over a range of temperature and pressure varying from 300 to 900°C and 500 to 1000 MPa, respectively; and, the fluid facilitating monazite crystallisation is characterised by pure H2O to diluted solutions with CO2 or KCl and can be REE-rich (Harlov and Förster, Reference Harlov and Förster2003; Trofanenko et al., Reference Trofanenko, Williams-Jones, Simandl and Migdisov2016).
The columbite–pyrochlore composite grains exhibit variable proportions and intergrowth patterns but no core–rim structure and fine veinlet network; hence, they are not replacing each other (Chudy, Reference Chudy2014) and are altered during late-to-post magmatic stages. However, the effects of alteration are much more prominent for pyrochlore than columbite-(Fe), as indicated by the porous texture and composition of pyrochlore (discussed below). Unlike carbonates and apatite, columbite-(Fe) and pyrochlore are relatively more resistant to deformation. Baryte is exclusively present at peripheral regions of composite grains, constituting post-magmatic mineral assemblage with monazite.
Mineralogical evolution
Carbonatitic apatite are characterised by Cl-poor fluorapatite or F-rich hydroxylapatite compositions, containing 0.2–2.7 wt.% SrO, up to 4.5 wt.% REE2O3 and 0.8 wt.% Na2O (Chakhmouradian et al., Reference Chakhmouradian, Reguir, Zaitsev, Couëslan, Xu, Kynický, Hamid Mumin and Yang2017). Newania apatite fall well within the compositional range of magmatic apatite in carbonatites except for depletion in REE. Secondary apatite in carbonatites can crystallise by solution and reprecipitation of primary fluorapatite (Basu and Bhattacharya, Reference Basu and Bhattacharyya2014) and show enrichment in heavy REE contents, such as in the case of the Songwe Hill, Tundulu and Kangankunde carbonatite complexes in Malawi (Broom-Fendley et al., Reference Broom-Fendley, Styles, Appleton, Gunn and Wall2016, Reference Broom-Fendley, Brady, Wall, Gunn and Dawes2017). However, no such compositional features are evident in Newania apatite, indicating negligible effects of deformation on composition.
Amphiboles in the Newania carbonatites are of both magmatic and metamorphic origin. Ferri-winchite, together with previously published amphibole compositions viz. ferri-katophorite, magnesio-arfvedsonite and magnesio-riebeckite (Viladkar and Wimmenauer, Reference Viladkar and Wimmenauer1986; Doroshkevich et al., Reference Doroshkevich, Ripp and Viladkar2010a; Ray et al., Reference Ray, Pande, Bhutani, Shukla, Rai, Kumar, Awasthi, Smitha and Panda2013) are magmatic amphiboles and represent the middle-to-late stage of amphibole crystallisation from parent carbonatite-forming melt (Samoylov, Reference Samoylov1977; Martin, Reference Martin, Hawthorne, Oberti, Della Ventura and Mottana2007). The low contents of Al and Ti indicate no assimilation and contamination of parent carbonatite magma by crustal material (Chakhmouradian and Zaitsev, Reference Chakhmouradian and Zaitsev2002; Chudy, Reference Chudy2014). Cummingtonite is a metamorphic amphibole, rare among igneous rocks and crystallises under regional metamorphic conditions in low-Ca amphibolites (Deer et al., Reference Deer, Howie and Zussman2013). There is no textural evidence to verify if the early crystallised magmatic amphiboles are later replaced by cummingtonite. Examples of metamorphic amphiboles in carbonatites are not common. Lastochkin et al. (Reference Lastochkin, Ripp and Doroshkevich2011) and Chudy (Reference Chudy2014) have reported metamorphic amphiboles, compositionally equivalent to tremolite–actinolite and anthophyllite. Chudy (Reference Chudy2014) postulated the origin of tremolite–actinolite in the presence of ferroan dolomite during metamorphic growth.
The Newania pyrochlores have U–Ta–Ti-rich compositions and significant A- and Y-site vacancies which show that the pyrochlores are magmatic in nature and have undergone alteration during late-to-post magmatic conditions. The U–Ta–Ti-rich compositions are indicative of early-stage crystallisation from the parent carbonatite magma (Chakhmouradian and Mitchell, Reference Chakhmouradian and Mitchell1998) as the contents of U and B-site cations remain constant during alteration (Lumpkin and Ewing, Reference Lumpkin and Ewing1995). The development of vacancies at A- and Y-sites is a consequence of secondary alteration indicated by the leaching of cations and anions, cation exchange and hydration (Lumpkin and Ewing, Reference Lumpkin and Ewing1995; Chakhmouradian and Mitchell, Reference Chakhmouradian and Mitchell1998). Nasraoui and Bilal (Reference Nasraoui and Bilal2000) have described similar supergene alteration of carbonatitic pyrochlore in the Lueshe carbonatite complex (Democratic Republic of Congo). The source for U, Ta and Nb must be the parental carbonatite-forming magma as no other U-bearing phases are present. Hence, it is unlikely that the U-enrichment in Newania pyrochlores is secondary in nature.
The compositional data for columbite–tantalite-group minerals from carbonatites are available for only a few occurrences (Chudy, Reference Chudy2014; Chakhmouradian et al., Reference Chakhmouradian, Reguir, Kressall, Crozier, Pisiak, Sidhu and Yang2015; Mitchell and Smith, Reference Mitchell and Smith2017; Boukirou et al., Reference Boukirou, Bouabdellah, Chakhmouradian, Mouttaqi, Reguir, Hauff, Cuney, J́ebrak, Yans and Hoernle2022), and data on co-crystallisation of columbite and pyrochlore in carbonatite is even rarer. Experimental work of Lumpkin and Ewing (Reference Lumpkin and Ewing1995) shows that pyrochlore can co-exist with columbite-(Fe) under moderate a Fe2+ and a Ca2+ and low-to-moderate a Na+ conditions. Chudy (Reference Chudy2014) has postulated that under decreasing a Na+ / a Ca2+ conditions, columbite-(Fe) and pyrochlore can become stable.
Crystallisation sequence
The crystallisation sequence of minerals in Newania carbonatites as interpreted from textural and compositional data is given in Fig. 11. The earliest crystallising phase in dolomite carbonatite is Ap-1 which formed bands/layers in the north-western region of the complex. This was followed by the crystallisation of Amp-1 and columbite–pyrochlore composite grains. Ap-2 and magnesian siderite are co-crystallised. Clb-2, which occurs as individual grains of columbite is formed later than Clb-1. Ferroan dolomite displays an extended crystallising history and crystallised always after the non-carbonate phases. Monazite is a hydrothermal phase which crystallised during the post-magmatic stage of carbonatite evolution. These monazite grains are not deformed whereas the surrounding Ap-1 and ferroan dolomite grains show deformation features, and hence are crystallised later than the deformation event. Baryte is a late-stage phase which crystallised as a rim on columbite–pyrochlore composite grains.

Figure 11. Schematic diagram showing crystallisation sequence of constituent minerals in dolomite carbonatite at Newania.
Origin of Newania carbonatites
The origin of carbonatites from mantle-derived magmas might be explained by three processes: (1) direct low-degree partial melting of carbonated peridotite at mantle depths exceeding 70 kms (e.g. Wyllie and Huang, Reference Wyllie and Huang1975; Wallace and Green, Reference Wallace and Green1988); (2) separation of conjugate silicate and carbonate magma from parent carbonated silicate magma by liquid immiscibility (e.g. Kjarsgaard and Peterson, Reference Kjarsgaard and Peterson1991; Mitchell, Reference Mitchell2009; Weidendorfer et al., Reference Weidendorfer, Schmidt and Mattsson2017); and (3) generation of carbonatitic residual magma by fractional crystallisation of carbonated silicate magma (e.g. Watkinson and Wyllie, Reference Watkinson and Wyllie1971; Mitchell, Reference Mitchell2005). The nature of the parental magma of the Newania carbonatites is indicated by the dominance of dolomite carbonatite and the absence of genetically-related silicate rock(s). This observation suggests derivation of a parent magma directly by melting of mantle peridotite.
Experimental studies (Wyllie and Huang, Reference Wyllie and Huang1975; Wallace and Green, Reference Wallace and Green1988) have shown that primary carbonate-bearing magmas can be generated by low-degree partial melting of carbonate-amphibole-bearing lherzolite, containing 0.3% H2O and 0.5–2.5% CO2 at pressures between 2.1 to 3 GPa and temperatures ranging from 930 to 1080°C. Such carbonatite magma has a dolomitic composition, with high contents of Ca, Mg, Na and Fe (decreasing abundance) and minor K and P, which crystallises dolomite (FeO = 4.4 wt.%) and Na–Mg carbonates. The presence of H2O and CO2 as volatile species in the experiments represent fairly oxidising conditions in the mantle source. Brey et al. (Reference Brey, Brice, Ellis, Green, Harris and Ryabchikov1983) concluded that the carbonatite magma generated from phlogopite-bearing mantle peridotite becomes more magnesian as the carbonate phase changes from dolomite to magnesite at ~3.2 GPa. Falloon and Green (Reference Falloon and Green1990) and Jago and Gittins (Reference Jago and Gittins1991) have demonstrated that F plays an important role in lowering of liquidus temperature of carbonates whereas H2O has little effect on peridotite solidus, thus indicating low solubility of H2O in carbonatite magma.
The mineralogy of dolomite carbonatite contains significant modal abundances of F-bearing non-carbonate phases such as apatite and amphibole, indicating presence of F, P, Si and alkalis (Na>K) in the parent magma. These observations are consistent with the experimentally produced carbonatite magma compositions of Wallace and Green (Reference Wallace and Green1988). The crystallisation of magmatic magnesian siderite is possible only from a carbonatite magma with high magnesian content. Such magma might be produced from magnesite–phlogopite-bearing mantle peridotite at depths exceeding 3.2 GPa (Brey et al., Reference Brey, Brice, Ellis, Green, Harris and Ryabchikov1983; Buckley and Woolley, Reference Buckley and Woolley1990; Woolley and Buckley, Reference Woolley and Buckley1993). The occurrence of Ap-1 and pyrochlore as early-crystallised phases suggest that the parent magma inherently contains a few percent of phosphate and fluorine (Wyllie and Biggar, Reference Wyllie and Biggar1966; Jago and Gittins, Reference Jago and Gittins1993; Mitchell and Kjarsgaard, Reference Mitchell and Kjarsgaard2004). On the basis of mineralogical studies and previously published literature for the Newania carbonatites, it is concluded that the parent magma for Newania carbonatites is produced by low-degree partial melting of magnesite–phlogopite-bearing peridotite at pressures reaching up to 3 GPa. The magma generated is dolomitic in composition, possibly more magnesian in composition and contains significant contents of Si, F, P and alkalis (Na and K).
The dolomite carbonatite occurrences at Gleibat Lafhouda (~1.85 Ga; Montero et al., Reference Montero, Haissen, Mouttaqi, Molina, Errami, Sadki, Cambeses and Bea2016) and Veseloe (~593 Ga; Doroshkevich et al., Reference Doroshkevich, Wall and Ripp2007) are similar to the Newania carbonatites. Both of these carbonatite occurrences are exclusively dolomite carbonatite-dominated complexes, devoid of genetically-related silicate rock(s) and emplaced in an extensional environment (Doroshkevich et al., Reference Doroshkevich, Wall and Ripp2007; Boukirou et al., Reference Boukirou, Bouabdellah, Chakhmouradian, Mouttaqi, Reguir, Hauff, Cuney, J́ebrak, Yans and Hoernle2022). Doroshkevich et al. (Reference Doroshkevich, Wall and Ripp2007) have postulated that the calcite-bearing dolomite carbonatite at Veseloe has crystallised from a rapidly transported dolomitic magma that originated within the mantle. Boukirou et al. (Reference Boukirou, Bouabdellah, Chakhmouradian, Mouttaqi, Reguir, Hauff, Cuney, J́ebrak, Yans and Hoernle2022) have suggested a similar origin for the Gleibat Lafhouda dolomite carbonatites, stating crystallisation from an Mg-rich magma, originated by very low-degree partial melting of mantle containing garnet and phlogopite. Furthermore, the emplacement coincides with the plume-initiated breakup of the Columbia supercontinent (ca. 1.85 Ga). Laiwu-Zibo carbonatites (China) are another example of such mantle-derived carbonatites. These consist of a complete carbonatite series of calcio-, magnesio- and ferrocarbonatites which are suggested to be generated directly from partial melting of Mesozoic enriched lithosphere (Ying et al., Reference Ying, Zhou and Zhang2004).
Conclusions
The Newania carbonatite complex is a plutonic carbonatite occurrence consisting principally of ferroan dolomite carbonatite with lesser ferrocarbonatite. The complex has undergone post-emplacement deformation which has affected the original textures and compositions of constituent minerals and resulted in the crystallisation of new minerals. Ferroan dolomite has undergone a maximum degree of deformation, ranging from brittle-to-ductile deformation regimes and subsequent re-crystallisation. Two types of apatite, Ap-1 and Ap-2 crystallised during the early- and late-stages of carbonatite evolution, respectively. Two new compositions of amphiboles viz. ferri-winchite and cummingtonite are found where the former has a magmatic origin and the latter probably crystallised during deformation. Pyrochlore is a magmatic phase, crystallised during early stages of evolution, which has undergone supergene alteration during late- to post-magmatic stages. However, the U–Ta–Ti-rich signature of magmatic origin remained unchanged during alteration. Monazite and baryte constitute a post-magmatic mineral assemblage. The parent melt for Newania carbonatites is considered to be a silica-bearing magnesian carbonate magma derived from low degree partial melting of magnesite–phlogopite-bearing peridotite at pressures equivalent to 3 GPa.
Acknowledgements
AS is thankful to the Council of Scientific and Industrial Research, Government of India for providing fellowship for doctoral work. The authors express their gratitude to Prof. Suresh C. Patel of IIT, Bombay for generating EPMA data. AS and DSS acknowledge the Director, CSIR-NGRI, Hyderabad for providing permission to use analytical facilities at NGRI. AS thanks Parminder Kaur, Sanchit Garg, Jaspreet Saini and Praveen Tantkar for help and guidance during fieldwork. Two anonymous Journal reviewers, Associate Editor Leone Melluso and Production Editor Helen Kerbey are thanked for providing comments and suggestions. Principal Editor Stuart Mills is thanked for editorial handling.
Supplementary material
The supplementary material for this article can be found at https://doi.org/10.1180/mgm.2023.61.
Competing interests
The authors declare none.