Introduction
Increasing evidence indicates that sulfur amino acids, methionine and cysteine, are implicated in numerous biological functions and diseases, aside from their role in protein synthesis(Reference Stipanuk1–Reference Brosnan and Brosnan3). Methionine is an indispensable amino acid and is transmethylated intracellularly to homocysteine via S-adenosylmethionine (SAM), the principal biological methyl donor in mammalian cells and a precursor for polyamine synthesis(Reference Mato, Corrales and Lu4) (Fig. 1). Reduced SAM concentrations, as a consequence of low methionine intake or folate deficiency, mainly lead to a deregulation in DNA methylation and are implicated in various cancers, including colorectal cancer(Reference Kim5, Reference Giovannucci, Rimm and Ascherio6). Homocysteine is a sulfur-containing amino acid present in the blood and tissues but not incorporated into protein(Reference MacCoss, Fukagawa and Matthews7). Numerous clinical studies have shown that elevated plasma homocysteine levels are strongly associated with increased risk for several diseases, including CVD, stroke, Alzheimer's disease and osteoporosis(Reference Zhou and Austin8–Reference Herrmann, Peter and Umanskaya10). Homocysteine can be converted into cysteine via cystathionine through the trans-sulfuration pathway, an irreversible process(Reference Stipanuk1) (Fig. 1). Homocysteine can also be methylated back to methionine via the remethylation pathway (Fig. 1). The combination of transmethylation and remethylation pathways comprises the methionine cycle which occurs in most cells. However, the trans-sulfuration pathway has a limited tissue distribution and is restricted to the liver, kidney, intestine, pancreas and adrenals(Reference Brosnan and Brosnan3, Reference Zlotkin and Anderson11). Cysteine is considered a semi-indispensable amino acid whose availability is dependent upon methionine intake(Reference Stipanuk1). However, dietary cysteine can satisfy a proportion of the sulfur amino acid requirement, the so-called cysteine-sparing effect on dietary methionine requirement(Reference Ball, Courtney-Martin and Pencharz12). Cysteine is a constituent amino acid of the tripeptide glutathione (γ-Glu-Cys-Gly), the major cellular antioxidant in mammals, and serves also as a precursor for the synthesis of taurine, pyruvate, sulfate and hydrogen sulfide (H2S) (Fig. 1).
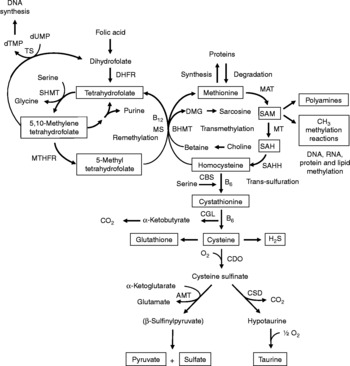
Fig. 1 Sulfur amino acid metabolism. AMT, aminotransferase; BHMT, betaine-homocysteine methyltransferase; CBS, cystathionine β-synthase; CDO, cysteine dioxygenase; CGL, cystathionine γ-lyase; CSD, cysteine sulfinate decarboxylase; DHFR, dihydrofolate reductase; DMG, dimethyl-glycine; dTMP, thymidylate; dUMP, deoxyuridylate; MAT, methionine adenosyltransferase; MS, methionine synthase; MT, methyl transferases; MTHFR, methylenetetrahydrofolate reductase, SAH, S-adenosylhomocysteine; SAHH, S-adenosylhomocysteine hydrolase; SAM, S-adenosylmethionine; SHMT, serine hydroxymethyltransferase; TS, thymidylate synthetase.
Although the liver is usually considered as one of the major organs in the body that metabolises sulfur amino acids, the gastrointestinal tract (GIT), and especially the intestine, appears to be also a significant site of dietary sulfur amino acid metabolism. Several reports indicate that intestinal sulfur amino acid metabolism is nutritionally important for normal gut function and intestinal mucosal growth. Dysregulation in the intestinal sulfur amino acid metabolism leads to numerous complications and diseases. In the present review, we will discuss the evidence of sulfur amino acid metabolism in the GIT and its functional and nutritional importance in health and disease.
Isotopic approaches to study sulfur amino acid metabolism
The introduction of stable-isotope tracer methodology has enabled the measurement of sulfur amino acid metabolism in vivo in human subjects, including adults and infants. Storch et al. (Reference Storch, Wagner and Burke13, Reference Storch, Wagner and Burke14) were the first to explore sulfur amino acid metabolism in human subjects using a stable-isotope approach. Their model was based on the intravenous infusion of a doubly-labelled methionine isotopomer [1-13C; methyl-2H3]methionine to determine the whole-body methionine carboxyl and methyl fluxes which provided estimates of transmethylation, trans-sulfuration and remethylation rates (Fig. 2). These studies demonstrated the role of feeding, fasting and the sparing effect of cysteine on kinetics of methionine catabolism in man and they defined the relative recycling of methionine through the remethylation of homocysteine(Reference Storch, Wagner and Burke14). In their methionine kinetic calculations, Storch et al. used an estimate of the intracellular methionine isotopic enrichment based on the intracellular dilution of leucine estimated with plasma α-ketoisocaproate enrichments observed by Matthews et al. (Reference Matthews, Schwarz and Yang15), yet this assumption does not reflect the true physiological effect of methionine metabolism. More recently, this model has been modified by MacCoss et al. (Reference MacCoss, Fukagawa and Matthews7) and others using plasma homocysteine enrichments to correct the intracellular methionine enrichments, since plasma homocysteine can be derived only from intracellular homocysteine formed by methionine transmethylation. Consistent with other reports(Reference Tessari, Kiwanuka and Coracina16, Reference Tessari, Coracina and Kiwanuka17), the rates of methionine kinetics were substantially higher after homocysteine correction than previous estimates.

Fig. 2 Stable isotopic tracer model used by Storch et al. (Reference Storch, Wagner and Burke14). This model is based on the intravenous infusion of a methionine isotopomer [1-13C; methyl-2H3]methionine [M+4]. The [2H3]methyl group is lost during transmethylation from S-adenosylmethionine (SAM) to S-adenosylhomocysteine (SAH), whereas the [13C]carbon group is transferred to [1-13C]homocysteine [M+1], [1-13C]cystathionine [M+1] and finally, oxidised to 13CO2 via α-ketobutyrate in the tricarboxylic acid cycle.
Few other studies have used different isotopomers to study whole-body methionine metabolism. A complication of the measurement of homocysteine remethylation rate is that the reaction can be catalysed by two enzymic pathways. Homocysteine can be methylated back to methionine by the ubiquitously distributed methionine synthase using 5-methyltetrahydrofolate as a methyl donor, and also in a folate-independent pathway by betaine-homocysteine methyltransferase (Fig. 1), which is expressed only in the liver and the kidney(Reference Brosnan and Brosnan3). To distinguish between the methyl groups transferred by the two homocysteine methyltransferases, Davis et al. (Reference Davis, Stacpoole and Williamson18) used a combined isotopic approach of [U-13C]methionine and [3-13C]serine. With this tracer combination, the remethylated methionine tracer, i.e. [13C4]methionine, was measured separately from the [13C1]methionine generated by the folate-dependent homocysteine remethylation pathway from [3-13C]serine. Using this tracer model, they determined that serine is the predominant one-carbon donor for homocysteine remethylation in healthy females with adequate folate, vitamin B12 and vitamin B6 nutritional status. The steady-state model of Storch et al. was also discussed by Hoffer(Reference Hoffer19) who concluded that this model can not provide information about the type and the severity of metabolic blocks that cause, for instance, hyperhomocysteinaemia. Based on this appreciation, Shinohara et al. (Reference Shinohara, Hasegawa and Ogawa20) examined the remethylation of homocysteine after a single administration of [2H7]methionine in rats under folate and/or choline (a precursor of betaine) deficiency. With a 2H label in the methyl position, this model has the advantage to recognise directly the elimination of methionine and to determine the extent of homocysteine remethylation by measuring [2H7]methionine, [2H4]homocysteine, [2H4]methionine and unlabelled methionine by GC-MS. Their results showed that plasma levels of remethylated methionine were influenced by choline deficiency rather than folate deficiency.
Important contributions to our knowledge of the dietary requirement for sulfur amino acids and the effect of cysteine on methionine requirement in man have resulted from recent studies using tracer isotopic approaches(Reference Ball, Courtney-Martin and Pencharz12, Reference Di Buono, Wykes and Cole21–Reference Fukagawa23). The ability of cysteine to provide a proportion of the total sulfur amino acid requirements in man, hence providing a sparing effect on methionine requirement, has been extensively debated(Reference Ball, Courtney-Martin and Pencharz12, Reference Di Buono, Wykes and Cole21, Reference Fukagawa23). Many studies performed in animals have shown that more than 40 % of the sulfur amino acid requirement can be met by dietary cysteine(Reference Ball, Courtney-Martin and Pencharz12, Reference Baker, Clausing and Harmon24–Reference Baker28). However, the cysteine-sparing effect on methionine requirement in adult human subjects was not found in a set of studies using methionine and cysteine tracers(Reference Storch, Wagner and Burke13, Reference Hiramatsu, Fukagawa and Marchini29–Reference Raguso, Regan and Young32). In these human studies, the methionine and cysteine intake was based on the 1985 FAO, WHO and United Nations University recommendation of 13 mg/kg per d, which has been recently found to be deficient for humans(Reference Di Buono, Wykes and Ball33, Reference Kurpad, Regan and Varalakshmi34). The failure to detect a cysteine-sparing effect in those studies(Reference Storch, Wagner and Burke13, Reference Hiramatsu, Fukagawa and Marchini29–Reference Raguso, Regan and Young32) has been discussed by Ball et al. (Reference Ball, Courtney-Martin and Pencharz12) and appears to be related to the methionine intake below the minimum obligatory requirement, which has not been defined yet. More recent stable-isotope studies in human subjects, using indicator amino acid oxidation(Reference Di Buono, Wykes and Ball35) and 24 h indicator amino acid oxidation and indicator amino acid balance(Reference Kurpad, Regan and Varalakshmi22) techniques to determine the sulfur amino acid requirement, have found a sparing effect of methionine requirement by cysteine with graded levels of methionine in the absence and presence of excess cysteine in the diet. These studies(Reference Kurpad, Regan and Varalakshmi22, Reference Di Buono, Wykes and Ball35) combined with earlier N balance studies(Reference Albanese, Holt and Davis36–Reference Reynolds, Steel and Jones38) provide evidence of a cysteine-sparing effect in man that ranges from 17 to 90 %. The sparing effect of cysteine on dietary methionine requirement appears to occur by reducing methionine catabolism through trans-sulfuration to cystathionine, which is related to a decrease in the concentration of cystathionase β-synthase (CBS), the enzyme that synthesises cystathionine from homocysteine (Fig. 1), and SAM (Fig. 1), a positive effector of the CBS enzyme(Reference Finkelstein, Martin and Harris39). Most recently, it has been shown that when total sulfur amino acid needs are provided as methionine at 14 mg/kg per d in the presence of an adequate protein intake of 1 g/kg per d(Reference Humayun, Elango and Ball40), extra cysteine does not increase the synthesis of erythrocyte glutathione, the major cellular antioxidant, in healthy adult humans(Reference Courtney-Martin, Rafii and Wykes41). Moreover, the cysteine-sparing effect on methionine requirement has been also estimated in parenterally and enterally fed piglets and the safe methionine intake with an excess of cysteine was determined to be 0·27 and 0·36 g/kg per d in parenteral and enteral feeding, respectively(Reference Shoveller, Brunton and House27). The significant difference in methionine requirement between parenteral and enteral feeding indicates that the gut utilises about 28 % of the total sulfur amino acid requirement(Reference Shoveller, Brunton and House27), suggesting an active metabolic role of the gut in the whole-body sulfur amino acid metabolism, although the cysteine-sparing effect is not dependent on first-pass metabolism in the gut.
Evidence of intestinal sulfur amino acid metabolism
In 1965, Mudd et al. (Reference Mudd, Finkelstein and Irreverre42) were the first to show significant levels of three enzymes involved in the trans-sulfuration pathway, i.e. methionine adenosyltransferase (MAT), CBS and cystathionine γ-lyase, in rat small intestine mucosa, but at lower activities for one or more enzymes compared with the liver, pancreas and kidney. In 1972, Stegink & Den Besten(Reference Stegink and Den Besten43) then showed that splanchnic organs are an important site of transmethylation and trans-sulfuration of dietary methionine for the synthesis of cysteine in human adults. They found a twofold higher plasma cystine concentration in adults given a continuous cyst(e)ine-free nutrient solution for 2 weeks via the nasogastric route compared with the intravenous route. Since then, others studies have shown that total parenteral nutrition without cysteine results in low circulating levels of cystine in newborn infants(Reference Zlotkin, Bryan and Anderson44) and neonatal piglets(Reference Shoveller, Brunton and Pencharz45). In agreement with the study from Stegink & Den Besten(Reference Stegink and Den Besten43), plasma cystine concentrations were higher in enterally than parenterally fed piglets administered methionine as the sole sulfur amino acid source(Reference Shoveller, Brunton and Pencharz45). These data suggest that the first-pass splanchnic metabolism is important for the synthesis of cysteine in neonates as well as adults.
Significant advancements in our understanding of splanchnic amino acid metabolism were derived from in vivo measurements of splanchnic organ balance(Reference Stoll and Burrin46). Pig models have been extensively utilised to study human nutrition since they have similar physiology to man, especially gut physiology. Our studies in piglets showed that the net portal absorption of several indispensable amino acids, including methionine, was significantly less than 100 % of the dietary intake, ranging from 40 to 70 %(Reference Stoll, Henry and Reeds47). The importance of the gut was also demonstrated in studies where the whole-body methionine requirement was 30 % greater in enterally fed than parenterally fed piglets(Reference Shoveller, Brunton and Pencharz45, Reference Shoveller, House and Brunton48). Our recent study in infant piglets using the Storch et al. isotopic model indicates that the GIT metabolises 20 % of dietary methionine intake which is mainly transmethylated to homocysteine and trans-sulfurated to cysteine(Reference Riedijk, Stoll and Chacko49). The GIT accounts for about 25 % of whole-body transmethylation and trans-sulfuration(Reference Riedijk, Stoll and Chacko49). More direct evidence of intestinal sulfur amino acid metabolism was found in studies in rat by Finkelstein(Reference Finkelstein50, Reference Finkelstein51), demonstrating that the small intestine possesses the enzymes necessary to metabolise methionine to cysteine, albeit at significantly lower activities than the liver. In our most recent piglet study, we also found significant enzymic activities of MAT, CBS and methionine synthase in the small intestine (jejunum and ileum)(Reference Bauchart-Thevret, Stoll and Chacko52), enzymes that are involved in the methionine transmethylation, trans-sulfuration and remethylation pathways, respectively. All these studies provide compelling evidence of intestinal sulfur amino acid metabolism. However, recent studies in pigs found negligible catabolism of methionine in enterocytes(Reference Chen, Yin and Jobgen53, Reference Chen, Li and Wang54), suggesting that the metabolism of methionine in the intestine may result from the action of non-epithelial cells or luminal microbes in the intestinal mucosa.
Homocysteine, an intermediate metabolite formed during the metabolism of methionine to cysteine (Fig. 1), plays an important physiological role, since a dysregulation in homocysteine metabolism, leading to an elevated homocysteine plasma concentration, has been associated with an increased risk of CVD, stroke, Alzheimer's disease and osteoporosis(Reference Zhou and Austin8–Reference Herrmann, Peter and Umanskaya10). In our study in infant piglets, we demonstrated that the GIT is a site of net homocysteine release into the body(Reference Riedijk, Stoll and Chacko49). A recent study performed on female rats investigated tissue homocysteine metabolism using stable-isotope approaches and tried to assess the contribution of individual tissues to plasma homocysteine by measuring the isotopic enrichment(Reference Wilson, van den Borne and Calder55). Interestingly, in contrast with previous studies(Reference Stead, Brosnan and Brosnan56, Reference Mudd, Brosnan and Brosnan57), they did not find a dominant role for the liver as a source of plasma homocysteine. Other tissues with higher intracellular homocysteine enrichments than in the plasma, including the small intestine, may also contribute to the homocysteinaemia(Reference Wilson, van den Borne and Calder55).
As regards cysteine in the GIT, studies in pigs indicate that less than 100 % of dietary cysteine appears in the portal blood, suggesting intestinal utilisation of cysteine(Reference Stoll, Henry and Reeds47, Reference Bos, Stoll and Fouillet58). The first step in cysteine catabolism is its conversion to cysteine sulfinate via the enzyme cysteine dioxygenase (CDO) (Fig. 1). The cysteine sulfinate is then either decarboxylated via cysteine sulfinate decarboxylase to produce hypotaurine, which is further oxidised to taurine via a poorly understood mechanism, or transaminated to the putitative intermediate β-sulfinylpyruvate that spontaneously decomposes to pyruvate and sulfate(Reference Stipanuk, Ueki and Dominy59) (Fig. 1). Rodent studies with 14C-labelled cysteine showed significantly higher oxidation when given via the intragastric (70 %) than the intraperitoneal (41 %) route, suggesting that nearly half of the whole-body cysteine oxidation occurs in splanchnic tissues(Reference Stipanuk and Rotter60). More importantly, subsequent work demonstrated that rat enterocytes extensively metabolise cysteine via CDO to cysteine sulfinate(Reference Coloso and Stipanuk61). This is in accordance with the expression of the murine CDO gene in the small intestine(Reference Hirschberger, Daval and Stover62). In contrast to rodent studies, our recent studies in infant pigs showed that the fractional whole-body oxidation of enteral cysteine (22 %) is lower than parenteral cysteine (35 %)(Reference Cottrell, Stoll and Burrin63). We also found that splanchnic tissues (liver and gut) utilise about 40 % of dietary cysteine intake in first-pass metabolism(Reference Cottrell, Stoll and Burrin63). Intestinal absorption was the major metabolic fate of dietary cysteine, representing 75 % intake, indicating that the gut utilises 25 % of the dietary cysteine intake(Reference Cottrell, Stoll and Burrin63). We also determined that cysteine gut uptake represents about 65 % of the splanchnic first-pass uptake(Reference Cottrell, Stoll and Burrin63). In addition, our results in pigs suggest that gut tissues consume a substantial proportion of dietary splanchnic cysteine metabolism via non-oxidative pathways(Reference Cottrell, Stoll and Burrin63). We postulate that glutathione synthesis is a major non-oxidative metabolic fate for cysteine in the gut. Consistent with this, in vivo rodent studies with intravenous infusion of Reference Matthews, Schwarz and Yang[15N]cysteine indicate that an important metabolic fate of cysteine in the gut is incorporation into glutathione(Reference Malmezat, Breuille and Capitan64).
Taurine is also another endproduct of cellular cysteine catabolism(Reference Stipanuk1) (Fig. 1) and we recently found that taurine is produced by the small intestine in piglets(Reference Bauchart-Thevret, Stoll and Chacko52). Along with glutathione and cysteine, taurine plays an important role in the antioxidant function in the body(Reference Atmaca65), especially in the intestine which is constantly exposed to endogenous and exogenous diet-derived oxidants. Moreover, among the numerous other physiological functions of taurine (i.e. osmoregulation, retinal and cardiac function, diabetes modulator, hepatoprotection, neuroprotection(Reference Huxtable66, Reference Bouckenooghe, Remacle and Reusens67)), taurine is also involved in bile acid conjugation(Reference Bouckenooghe, Remacle and Reusens67). Bile acids are essential for intestinal digestion and absorption of lipids. In a recent study in adult patients with short-bowel syndrome(Reference Schneider, Joly and Gehrardt68), long-term parenteral nutrition was associated with an impaired tauro-conjugation of bile acids (enterohepatic pool) and a low biliary taurine concentration despite the long-term taurine intravenous supplementation, suggesting a partition between the systemic and the enterohepatic taurine pools. This finding suggests that the intestine plays an important role in providing taurine to the liver. Besides the fact that the majority of the intestinal taurine pool is derived from the diet and absorbed by the gut, some taurine might also come from endogenous synthesis in the gut from cysteine since CDO expression has been found in rodent small intestine(Reference Stipanuk, Ueki and Dominy59, Reference Hirschberger, Daval and Stover62). Moreover, we also found a decrease in taurine concentration in the ileum of piglets enterally fed a sulfur amino acid-free diet compared with a complete diet(Reference Bauchart-Thevret, Stoll and Chacko52), suggesting an intestinal synthesis of taurine from methionine and cysteine.
Catabolism of cysteine can also occur by desulfuration reactions that cleave the sulfur from cysteine to produce H2S (Fig. 1) via multiple pathways, mainly through CBS and cystathionine γ-lyase activity(Reference Stipanuk1, Reference Li, Bazer and Gao69). H2S has been found to be produced by the ileum(Reference Zhao, Ndisang and Wang70) and the colon(Reference Linden, Sha and Mazzone71) in rodents. H2S is a gas that has been reported to exert anti-inflammatory(Reference Wallace72), relaxant(Reference Teague, Asiedu and Moore73, Reference Gallego, Clave and Donovan74), prosecretory(Reference Schicho, Krueger and Zeller75) and antinociceptive(Reference Distrutti, Sediari and Mencarelli76) effects in the GIT. Factors that influence sulfur amino acid metabolism can also affect the production of H2S. Sulfur amino acid metabolism is regulated through the balance of production and disposal of cellular homocysteine and cysteine. Therefore, the release of homocysteine and H2S by the gut depends on the methionine utilisation by the gut, which then may have an impact on peripheral physiology. For example, a recent study in rats demonstrated that liver endothelial dysfunction caused by hyperhomocysteinaemia was reversed by exogenous H2S(Reference Distrutti, Mencarelli and Santucci77).
Studies on ruminants also showed kinetic evidence of methionine metabolism, i.e. transmethylation, remethylation and trans-sulfuration in the GIT(Reference Lobley, Connell and Revell78, Reference Lobley, Shen and Le79). Recent studies in lambs using stable-isotopic approaches demonstrated that the GIT is capable of methionine synthesis from the dietary methionine hydroxy analogue, namely 2-hydroxy-(4-methylthio)butanoic acid (HMB)(Reference Lobley, Wester and Calder80, Reference Lobley, Wester and Holtrop81). The methionine hydroxy analogue HMB is a synthetic source of methionine that is commonly added to commercial animal diets to ensure that the nutritional requirement for this essential amino acid is satisfied. The biochemical conversion of HMB to methionine involves two enzymic steps (Fig. 3). The first reaction is a stereospecific oxidation involving two enzymes: a peroxisomal l-2-hydroxy acid oxidase (l-HAOX) and a mitochondrial d-2-hydroxy acid dehydrogenase (d-HADH), which catalyses the oxidation of l-HMB and d-HMB, respectively, into 2-keto-(4-methylthio)butanoic acid(Reference Dibner and Knight82). The second reaction is the transamination of 2-keto-(4-methylthio)butanoic acid to l-methionine. This reaction is ubiquitous and does not constitute a limiting step in the metabolic conversion of dl-HMB to l-methionine(Reference Rangel-Lugo and Austic83). However, the specific l-HAOX activity has been found in chicken liver and kidney(Reference Dibner and Knight82, Reference Ferjancic-Biagini, Dupuis and De Caro84), rat liver(Reference Langer85), pig kidney(Reference Robinson, Keay and Molinari86) and also in liver, kidney and ruminal epithelia in sheep(Reference McCollum, Vazquez-Anon and Dibner87), whereas the d-HADH has been detected in numerous tissues in chickens, including liver, kidney, skeletal muscle, intestine, pancreas, spleen and brain(Reference Dibner and Knight82), and in liver, kidney and ruminal epithelia in sheep(Reference McCollum, Vazquez-Anon and Dibner87). Studies in lambs showed that more than 85 % of the [1-13C]HMB infused directly into the abomasum was absorbed into the portal vein and substantial enrichment of [1-13C]methionine occurred in the small- and large-intestinal tissues(Reference Lobley, Wester and Calder80, Reference Lobley, Wester and Holtrop81). Moreover, recent studies in chickens have also demonstrated the capacity of the small intestine for significant HMB conversion to methionine(Reference Martin-Venegas, Soriano-Garcia and Vinardell88, Reference Martin-Venegas, Geraert and Ferrer89). It is now well established that HMB is a safe and efficacious precursor of methionine in chicks(Reference Dilger, Kobler and Weckbecker90, Reference Stipanuk91). Although it still remains unknown whether the human GIT is capable of methionine synthesis from HMB, dl-HMB is currently used as an enteral product (Ketosteril®, Fresenius Kabi), a mixture of ketoacids and amino acids, in conjunction with a low-protein diet, for patients suffering from renal insufficiency(Reference Stipanuk91, Reference Feiten, Draibe and Watanabe92).

Fig. 3 Metabolic pathway for the conversion of 2-hydroxy-(4-methylthio)butanoic acid (HMB) to l-methionine. KMB, 2-keto-(4-methylthio)butanoic acid.
Sulfur amino acids and intestinal mucosal growth and function
Methionine, cysteine and glutathione
The intestinal epithelium is one of the most dynamic sites of cell turnover in mammals(Reference Johnson93). Its growth status can be modulated by various stimuli, accompanied by corresponding changes in subepithelial mucosa. Total parenteral nutrition is commonly associated with a decrease in crypt cell proliferation and epithelial renewal rate(Reference Burrin, Stoll and Jiang94). Our results in piglets indicate that the minimal enteral nutrient intake necessary to increase mucosal mass was 40 % of total nutrient intake, whereas 60 % of enteral nutrition was necessary to sustain normal mucosal proliferation and growth(Reference Burrin, Stoll and Jiang95). We recently demonstrated that a sulfur amino acid-free diet administered enterally to piglets for 7 d led to a reduced intestinal mucosal growth associated with villus atrophy, reduced epithelial cell proliferation, lower goblet cell number and diminished small-intestinal redox capacity(Reference Bauchart-Thevret, Stoll and Chacko52). Moreover, using [1-13C-methyl-2H3]methionine and [15N]cysteine tracer approaches, we also found an alteration in methionine metabolism under sulfur amino acid-deficient conditions at both whole-body and intestinal levels, where the methionine pool was preserved for protein synthesis by up-regulation of homocysteine remethylation and suppression of trans-sulfuration(Reference Bauchart-Thevret, Stoll and Chacko52). The suppression of trans-sulfuration contributed to the diminished cellular cysteine and glutathione concentrations and increased oxidant stress, which seemed to preferentially affect intestinal growth, especially in the jejunum(Reference Bauchart-Thevret, Stoll and Chacko52).
Evidence suggests that intracellular redox status plays an important role in the control of intestinal epithelial cell proliferation and apoptosis where increased oxidant stress and redox imbalance suppress cell proliferation and induce apoptosis(Reference Pias and Aw96–Reference Aw99). Glutathione (γ-Glu-Cys-Gly) is the major low-molecular-weight thiol in the cells that controls cellular thiol/disulfide redox state(Reference Jones100) and serves as a major reservoir for cysteine(Reference Stipanuk1). Reduced glutathione (GSH) is a ubiquitous tripeptide present in high concentration in tissue, especially in the intestine(Reference Aw101). Cellular GSH homeostasis is maintained through de novo synthesis from precursor sulfur amino acids methionine and cysteine, regeneration from its oxidised form glutathione disulfide (GSSG), and uptake of extracellular intact GSH via Na+-dependent transport systems(Reference Aw101). Maintaining normal GSH concentration is essential to most tissues, especially the intestine, which is constantly challenged by luminal toxins and oxidants derived from the diet as well as endogenous generated reactive oxygen species. Indeed, a marked depletion of GSH, induced by buthionine sulfoximine, was shown to result in severe degeneration of jejunal and colonic epithelial cells in mice and this intestinal damage seemed to be prevented by concomitant GSH administration(Reference Martensson, Jain and Meister102). However, the notion of an essential need of GSH for cell growth appeared to be controversial(Reference Tian, Washizawa and Gu103). Changes in the extracellular cysteine/cystine redox status per se have been shown to mediate proliferative signalling that is independent of the intracellular GSH/GSSG in colon cancer cells(Reference Noda, Iwakiri and Fujimoto104, Reference Anderson, Iyer and Ziegler105). Thus, all these findings strongly suggest that sulfur amino acids methionine and cysteine play a key role in intestinal mucosal growth associated with a regulated redox status, and intestinal epithelial cell function.
S-adenosylmethionine in methylation reactions and polyamine synthesis
SAM has emerged as a key regulator of metabolism, proliferation, differentiation, apoptosis and cell death(Reference Martinez-Lopez, Varela-Rey and Ariz106). SAM is synthesised from methionine and ATP only by MAT (Fig. 4), which exists through three distinct isoforms (i.e. MAT I, MAT II and MAT III)(Reference Mato, Corrales and Lu4) that are the products of two different genes (i.e. MAT1A and MAT2A)(Reference Kotb, Mudd and Mato107). The gene MAT1A encodes the α1 catalytic subunit, which organises into dimers (MAT III) or tetramers (MAT I). The gene MAT2A encodes for the α2 catalytic subunit in the MAT II isoform. A third gene, MAT2β, encodes for a β regulatory subunit that regulates the activity of MAT II by lowering the inhibition constant (K i) for SAM and the Michaelis constant (K m) for methionine(Reference Halim, LeGros and Geller108). MAT1A is expressed mostly in adult liver, whereas MAT2A is widely distributed. MAT2A is also expressed by fetal liver but is replaced by MAT1A during development(Reference Lu and Mato109). Although MAT isoenzymes catalyse the same reaction, they are differentially regulated by their product, SAM. SAM maintains MAT1A expression in hepatocytes, but inhibits MAT2A expression(Reference Mato, Corrales and Lu4). In mammalian liver, MAT1A is a marker for the differentiated or mature liver phenotype, while MAT2A is a marker for rapid growth and dedifferentiation(Reference Lu and Mato109). The properties of mammalian MAT isoforms are summarised in Table 1. Few studies have examined the expression of MAT in intestinal tissues, yet the expression of the MAT2A gene and MAT II protein has been confirmed in human and mouse colonic tissue(Reference Ito, Ikeda and Kojima110, Reference Chen, Xia and Lin111). We recently confirmed MAT activity in piglet small intestine(Reference Bauchart-Thevret, Stoll and Chacko52). MAT2A expression has been shown to be up-regulated by growth factors, such as epidermal growth factor, insulin-like growth factor-1 and leptin, in colorectal cancer cells HT-29 and RKO and its inhibition using RNAi led to cell death(Reference Chen, Xia and Lin111). In addition, Chen et al. (Reference Chen, Xia and Lin111) also demonstrated that SAM and its metabolite methylthioadenosine (MTA) (Fig. 4) are able to down-regulate MAT2A gene expression at high doses. These findings clearly indicate that MAT2A gene expression is critical for cell survival where SAM appears as a key role in the regulation of intestinal cell proliferation.

Fig. 4 S-adenosylmethionine (SAM) metabolic pathways: transmethylation and polyamine synthesis. dcSAM, decarboxylated S-adenosylmethionine; MAT, methionine adenosyltransferase; MTA, methylthioadenosine; SAH, S-adenosylhomocysteine; SAMDC, S-adenosylmethionine decarboxylase; ODC, ornithine decarboxylase; PAO, polyamine oxidase; SSAT, spermidine/spermine N1-acetyltransferase; , methionine salvage pathway.
Table 1 Properties of mammalian methionine adenosyltransferase (MAT) isoforms (from Lu & Mato(Reference Lu and Mato145))

SAM, S-adenosylmethionine; HCC, hepatocellular carcinoma.
* K m values differ depending on the purity of the enzyme.
Besides its role as a constitutive precursor for protein synthesis, the role of methionine as a precursor for SAM synthesis may have greater regulatory importance for cell function and survival given its singular role in methylation reactions and control of gene expression. Once SAM is produced, it can be processed via two pathways, namely transmethylation or polyamine synthesis (Fig. 4). SAM is the major biological methyl group donor for a variety of methyltransferases, resulting in the methylation of substrates such as nucleic acids (DNA, RNA), proteins and lipids(Reference Chiang, Gordon and Tal112), as well as the synthesis of small molecules (for example, creatine, phosphatidylcholine, adrenaline), modification of xenobiotics (for example, thiols, arsenite) and inactivation of neurotransmitters (for example, adrenaline, noradrenaline, dopamine)(Reference Brosnan, da Silva and Brosnan113). DNA methylation is a major epigenetic modification of the genome that regulates crucial aspects of its function during development and in adults, including imprinting and X-chromosome inactivation(Reference Reik and Dean114). In mammalian cells, DNA methylation occurs mainly on cytosine residues at the C5 position within CpG dinucleotides to form 5-methylcytosine and this reaction is carried out by two important classes of DNA methyltransferases (DNMT). DNMT1 is essential for maintaining DNA-methylation patterns in proliferating cells, whereas DNMT3a and DNMT3b, two members of the second class of methyltransferases, are required for de novo methylation during embryonic development(Reference Patra, Patra and Rizzi115). Few studies have examined the epigenetic mechanisms underlying normal gut development. Most of our knowledge on epigenetic involvement, especially methylation reactions, in intestinal metabolism and physiology comes from epigenetic dysregulation in gastrointestinal carcinogenesis, such as in colorectal cancer. The role of sulfur amino acid metabolism in colorectal cancer will be discussed further in the present review.
In addition to its role in methylation, SAM is also involved in the synthesis of polyamines (Fig. 4) which are known to play a key role in cell proliferation by regulating the expression of various growth-related genes(Reference Moinard, Cynober and de Bandt116, Reference Bettuzzi, Davalli and Astancolle117). The intestinal epithelium is one of the most rapidly proliferating tissues in the body and, therefore, has a high demand for polyamines(Reference McCormack and Johnson118). Several studies have demonstrated that polyamines stimulate normal intestinal epithelial cell proliferation and are required for the maintenance of mucosal integrity(Reference McCormack and Johnson118, Reference Wang119). Very few studies have investigated the role of SAM in intestinal cell growth related to polyamine synthesis. SAM has been widely studied in hepatocytes since the liver is the major site of SAM synthesis and degradation(Reference Mato, Corrales and Lu4). A physiological increase in SAM has been reported to stimulate growth in liver cancer cells whereas a pharmalogical dose of SAM exerted a growth-inhibitory effect(Reference Ansorena, Garcia-Trevijano and Martinez-Chantar120). The effect of SAM at pharmalogical doses may be mediated by its metabolite MTA. SAM is very unstable and converts spontaneously to MTA in the polyamine synthesis (Fig. 4). In contrast to SAM, MTA has been shown to inhibit transmethylation and polyamine synthesis(Reference Clarke, Clarke and Tamanoi121). The removal of MTA by MTA phosphorylase is necessary both for the resynthesis of methionine (Fig. 5) and for polyamine synthesis, since MTA is a strong inhibitor of both spermine and spermidine synthases(Reference Grillo and Colombatto122). In colon cancer cells, 6 h of MTA treatment (1 mm) was shown to reduce polyamine level(Reference Chen, Xia and Lin111). In addition, it was recently reported that SAM and MTA are able to induce apoptosis in colon cancer cell lines but not in normal colon epithelial cells(Reference Li, Zhang and Oh123). These opposing effects of SAM and MTA on apoptosis in normal v. cancer cells have been also previously reported in hepatocytes(Reference Ansorena, Garcia-Trevijano and Martinez-Chantar120). However, the molecular mechanism of SAM and MTA on cell growth between colonocytes and hepatocytes may be different due to the presence of the MAT1A gene in hepatocytes that is absent in intestinal cells and which is differently regulated by SAM (Table 1)(Reference Mato, Corrales and Lu4). Interestingly, the proapototic effect of MTA was not blocked by increased doses of polyamines in colon cancer cells RKO(Reference Li, Zhang and Oh123). Polyamine depletion has been shown to both enhance and protect against apoptosis in a cell line- and stimulus-dependent manner(Reference Seiler and Raul124). Thus, all these data suggest that the concentration of SAM plays an important role in intestinal cell growth and apoptosis. A decrease in methionine intake or a deficiency in folate may disrupt methionine metabolism and have an impact on intestinal SAM pool and hence polyamine synthesis, which clearly emphasises the importance of dietary sulfur amino acid intake to maintain a normal intestinal growth.

Fig. 5 Methionine salvage pathway from 5′-methylthioadenosine (MTA). MTOB, 4-methylthio-2-oxobutanoic acid; MTR-1P, 5′-methylthioribose-1-P.
Sulfur amino acid metabolism and gastrointestinal diseases
Considerable epidemiological evidence suggests that a low-folate diet is associated with an increased risk of colorectal cancer(Reference Sanderson, Stone and Kim125). Like folate, choline and its oxidation product betaine are methyl-group donors that are involved in the remethylation of homocysteine to methionine (Fig. 1). Because homocysteine methylation by betaine via betaine-homocysteine methyltransferase activity is confined to the liver and the kidney(Reference Brosnan and Brosnan3), choline and betaine dietary intake may have less impact on the methylation of homocysteine in the colon than folate since methionine synthase that methylates homocysteine to methionine using 5-methyltetrahydrofolate as a methyl donor is ubiquitously distributed(Reference Brosnan and Brosnan3). One of the consequences of folate deficiency is SAM deficiency since folate is involved in the remethylation of homocysteine to methionine, the precursor of SAM (Fig. 1). However, folate supplementation can either prevent or exacerbate intestinal tumorigenesis, depending on the timing and dose of folate intervention(Reference Kim126). This may be explained by the function of folate in nucleotide synthesis (Fig. 1) where rapidly proliferating tissues, including tumours, have an increased requirement for nucleotides. At present, based on lack of compelling supportive evidence on the potential tumour-promoting effect and presence of some adverse effects(Reference Ulrich127, Reference Smith, Kim and Refsum128), a recent review concludes that folate supplementation should not be recommended as a chemopreventive measure against colorectal cancer(Reference Kim129). Interestingly, a low methionine intake, which decreases SAM levels and increases SAH levels, stimulates methylenetretrahydrofolate reductase (MTHFR) to irreversibly convert 5,10-methylenetetrahydrofolate to 5-methyltetrahydrofolate (Fig. 1)(Reference Giovannucci130). DNA methylation is an important epigenetic determinant in gene expression, in the maintenance of DNA integrity and stability, in chromatin modifications and in the development of mutations(Reference Jones and Baylin131). Aberrant DNA methylation, i.e. global genomic hypomethylation and hypermethylation of the promoter region of certain tumour-suppressor genes, has been implicated in colorectal carcinogenesis(Reference Duthie, Narayanan and Sharp132). MTHFR is an important regulatory enzyme in the metabolism of folate and the genetic polymorphism of MTHFR can affect DNA methylation and influence the risk of colorectal cancer, particularly in the presence of a low-folate diet(Reference Kim133). One of the most common functional polymorphisms of the MTHFR gene is the C677T polymorphism which results in an alanine-to-valine substitution at codon 222(Reference Frosst, Blom and Milos134). Individuals with the 677TT genotype (variant homozygotes) have no more than 30 % of normal enzyme activity, while heterozygotes (CT) have 65 % of normal enzyme activity(Reference Frosst, Blom and Milos134). A recent meta-analysis demonstrated that individuals with a homozygous variant of the MTHFR gene (677TT genotype) have a reduced risk of colorectal cancer(Reference Hubner and Houlston135). However, the beneficial effect of the 677TT genotype was reported to be abolished when folate or methionine intake is low and/or with a high alcohol intake(Reference Chen, Giovannucci and Kelsey136, Reference Ma, Stampfer and Giovannucci137).
Although there is an abundant literature on the role of folate in cancer risk, few studies have examined the role of SAM in the pathogenesis of colon cancer. One study examined MAT protein expression and activity in human colorectal cancer and found that MAT II activity is well correlated with the stage of colorectal tumour compared with normal tissue(Reference Ito, Ikeda and Kojima110). More recently, Chen et al. (Reference Chen, Xia and Lin111) found higher SAM levels in human colon cancer specimens and polyps from Min (multiple intestinal neoplasia) mice, associated with an increase in MAT2A gene expression and MAT II protein level. In addition, they demonstrated that SAM and its metabolite MTA, at pharmacological doses, are able to down-regulate MAT2A expression and to block the ability of growth factors (leptin, insulin-like growth factor-1 and epidermal growth factor) to induce MAT2A expression and exert their mitogenic response in colorectal cancer cells HT-29 and RKO(Reference Chen, Xia and Lin111). They also observed similar results in vivo in wild-type mice where SAM and MTA supplementation in drinking water for 6 d decreased MAT2A expression in proximal small intestine by 20 %(Reference Chen, Xia and Lin111). The increased levels of intracellular SAM in cancerous colonocytes are likely to support polyamine synthesis required for growth and differentiation. However, high-dose SAM treatment was shown to inhibit growth and the effect is mediated by MTA(Reference Chen, Xia and Lin111). Indeed, in contrast to SAM, MTA inhibits methylation and polyamine synthesis(Reference Avila, Garcia-Trevijano and Lu138). Therefore, like for folate, the dose of SAM supplementation may be a critical determinant in colorectal cancer susceptibility. In addition, due to their proapoptotic effect in colon cancer cells(Reference Li, Zhang and Oh123), SAM and MTA may be attractive agents in the chemoprevention and treatment of colon cancer.
Homocysteine has been associated with the pathophysiology of inflammatory bowel disease (IBD). The two major forms of IBD, Crohn's disease and ulcerative colitis, represent chronic inflammatory disorders characterised by progressive destructive inflammation in the GIT. Hyperhomocysteinaemia has been consistently found in patients with IBD, as well as an increased level of homocysteine in colonic mucosa(Reference Morgenstern, Raijmakers and Peters139, Reference Danese, Sgambato and Papa140). Elevated plasma and intestinal mucosal homocysteine concentration can result from either genetic defects affecting either the trans-sulfuration or remethylation pathways of homocysteine such as CBS deficiency or a polymorphism in MTHFR, or nutritional deficiencies of the vitamin cofactors (folate, vitamin B12 and vitamin B6) required for homocysteine metabolism (Fig. 1)(Reference Morgenstern, Raijmakers and Peters139, Reference Danese, Sgambato and Papa140). However, no direct link has been established between homocysteine and IBD. Several studies suggest that homocysteine may promote inflammatory processes through oxidative stress(Reference Papatheodorou and Weiss141) and contribute to the inflammatory state of the mucosal endothelium through endoplasmic reticulum stress(Reference Zhang, Cai and Adachi142). Although the aetiology of Crohn's disease and ulcerative colitis remains unknown, gut tissue injury is the result of an abnormal immune response and involves multiple non-immune cellular systems, including intestinal microvascular endothelial cells(Reference Papa, Scaldaferri and Danese143, Reference Deban, Correale and Vetrano144). Whether increased circulating or local homocysteine is involved in these phenomena warrants further study.
Conclusion
An increasing body of evidence supports the metabolic significance of the GIT in sulfur amino acid metabolism. Recent in vivo studies show that the intestinal metabolism of dietary sulfur amino acids methionine and cysteine is nutritionally important for normal intestinal mucosal growth. However, it still remains unknown how sulfur amino acid metabolism regulates gut growth beyond its role as a precursor of protein biosynthesis. Methionine and cysteine also are the precursors of important molecules that may contribute to intestinal mucosal growth and gut function. SAM appears to be a key molecule that links three important metabolic pathways, i.e. transmethylation, trans-sulfuration and polyamine synthesis with MTA production. Epigenetic regulation of gene expression via modulation of DNA methylation may be the critical role of SAM. Further studies are warranted to determine the role of SAM and its metabolite MTA in methylation reactions in intestinal epithelial cells and how the regulation of their synthesis can affect intestinal cell survival and proliferation. Also, glutathione plays an important role in intestinal gut function due to, in part, its role in redox status. Thus, the availability of cysteine, the rate-limiting amino acid in glutathione synthesis, appears to be important for the maintenance of epithelial cell glutathione concentration and hence in the regulation of intestinal cell redox status. However, further investigation needs to be done to determine whether the increase in dietary cysteine intake may be an effective strategy to treat diseases related to oxidant injury in the GIT. Another potentially important metabolite of methionine cycle activity is homocysteine. As regards to IBD, further studies are warranted to establish whether the association between homocysteine and IBD is a causal link or a consequence of a poor nutritional status, mainly vitamin deficiencies (folate, vitamin B12 and B6). In the case of SAM and MTA, these metabolites may have important roles in both colonic epithelial cell survival and inflammation that could also be relevant to IBD and cancer.
Acknowledgements
The present review is a publication of the US Department of Agriculture, Agricultural Research Service (USDA/ARS) Children's Nutrition Research Center, Department of Pediatrics, Baylor College of Medicine and Texas Children's Hospital, Houston, TX. It was supported by federal funds from the USDA/ARS under cooperative agreement number 58-6250-6-001. The contents of this publication do not necessarily reflect the views or policies of the USDA, nor does mention of trade names, commercial products, or organisations imply endorsement by the US Government. C. B.-T. was supported by grants from Association pour le Développement de la Recherche en Nutrition (ADREN)/Société Francophone Nutrition Clinique et Métabolisme (SFNEP), Nutricia Research Foundation, and Société Française de Nutrition (SFN).
We acknowledge the contribution of all authors in the writing of the present review.
The authors declare that they have no conflicts of interest.