Non-technical Summary
Animals first evolved more than 570 million years ago, during the Ediacaran time period, but it was not until well into the Cambrian time period, around 520 million years ago, that animal evolution really took off and most modern animal groups diversified. It is over this Ediacaran to Cambrian transition that we not only see animals first appear, but also the evolution of movement, the ability to burrow and to swim, and the very first reefs and macroscopic predators. There are likely many different factors that shaped this radiation of animal life, so in this review paper we discuss the ecology underlying this Ediacaran to Cambrian transition and place the individual specimens and taxa in the context of the environment in which they lived. After all, it is the interactions that organisms experienced in their daily lives with one another and their environment that led to the diversification of animal body plans, and the evolutionary patterns we observe over these crucial 75 million years. As early animals evolved, we see diversification in feeding and biological and environmental interactions. These ecological interactions started off relatively weak, with few interactions between organisms, but then increased throughout the Ediacaran and into the Cambrian. By 500 million years ago, the ecosystem structure was similar to that of marine systems today. However, there are time delays between the origins of structuring processes and the time when they have an observed impact on other organisms and their ecosystem. As such, while the key building blocks of ecosystem structure were in place by the end of the Cambrian, it takes evolutionary timescales for the impact of these innovationsto be realized.
Introduction
One of the most important evolutionary innovations in the history of life on Earth was the evolution of animals and the subsequent transformation of the biosphere. The appearance and diversity of body and trace fossils in the Cambrian is sufficiently quick that it was termed the Cambrian explosion in the 1970s (cf. Brasier Reference Brasier and House1979), with a rapid expansion of taxonomic and morphological diversity occurring in the Cambrian Epoch 2, where many animal phyla crown groups are first found (e.g., Erwin et al. Reference Erwin, Laflamme, Tweedt, Sperling, Pisani and Peterson2011; Zhang et al. Reference Zhang, Zhang, Ma, Taylor, Strotz, Jacquet, Skovsted, Chen, Han and Brock2021). These likely represent accurate timescales for animal origination and early diversification (Budd and Jensen Reference Budd and Jensen2017; Budd and Mann Reference Budd and Mann2023; Moody et al. Reference Moody, Álvarez-Carretero, Mahendrarajah, Clark, Betts, Dombrowski, Szánthó, Boyle, Daines, Chen and Lane2024). Since the 1970s, our understanding of the Cambrian Explosion and the origins and early evolution of metazoans have changed dramatically, with an appreciation that animals predate the Cambrian (Ford Reference Ford1958). Indeed it was only in the 1950s that complex, macroscopic fossils were established to have been found in rocks known to be pre-Cambrian (Ford Reference Ford1958), with six other localities known that are now dated as Ediacaran age (Billings and Billings Reference Billings and Billings1872; Gürich Reference Gürich1933; Sprigg Reference Sprigg1947; Misra Reference Misra1969; Sokolov Reference Sokolov1972; Fig. 1A–D). Yet it is only in the last few years that taxonomic affinities of some Ediacaran macrofossils have been resolved, with support from phylogenetic analyses, as total- and/or crown-group animals (Budd and Jensen Reference Budd and Jensen2017; Bobrovskiy et al. Reference Bobrovskiy, Hope, Ivantsov, Nettersheim, Hallmann and Brocks2018; Chen et al. Reference Chen, Zhou, Yuan and Xiao2019; Dunn et al. Reference Dunn, Liu, Grazhdankin, Vixseboxse, Flannery-Sutherland, Green, Harris, Wilby and Donoghue2021), supporting the diversification of early animals in the Ediacaran. The presence of Ediacaran animals (Bobrovskiy et al. Reference Bobrovskiy, Hope, Ivantsov, Nettersheim, Hallmann and Brocks2018; Chen et al. Reference Chen, Zhou, Yuan and Xiao2019; Dunn et al. Reference Dunn, Liu, Grazhdankin, Vixseboxse, Flannery-Sutherland, Green, Harris, Wilby and Donoghue2021), coupled to differences in when different groups radiated (Servais et al. Reference Servais, Cascales-Miñana, Harper, Lefebvre, Munnecke, Wang and Zhang2023), means that the term “Ediacaran/Cambrian transition” (ECT) (cf. Narbonne et al. Reference Narbonne, Myrow, Landing and Anderson1987) is a more appropriate term than Cambrian explosion for the radiation and diversification of early animals across the Ediacaran and Cambrian (e.g., Wood et al. Reference Wood, Liu, Bowyer, Wilby, Dunn, Kenchington, Cuthill, Mitchell and Penny2019).
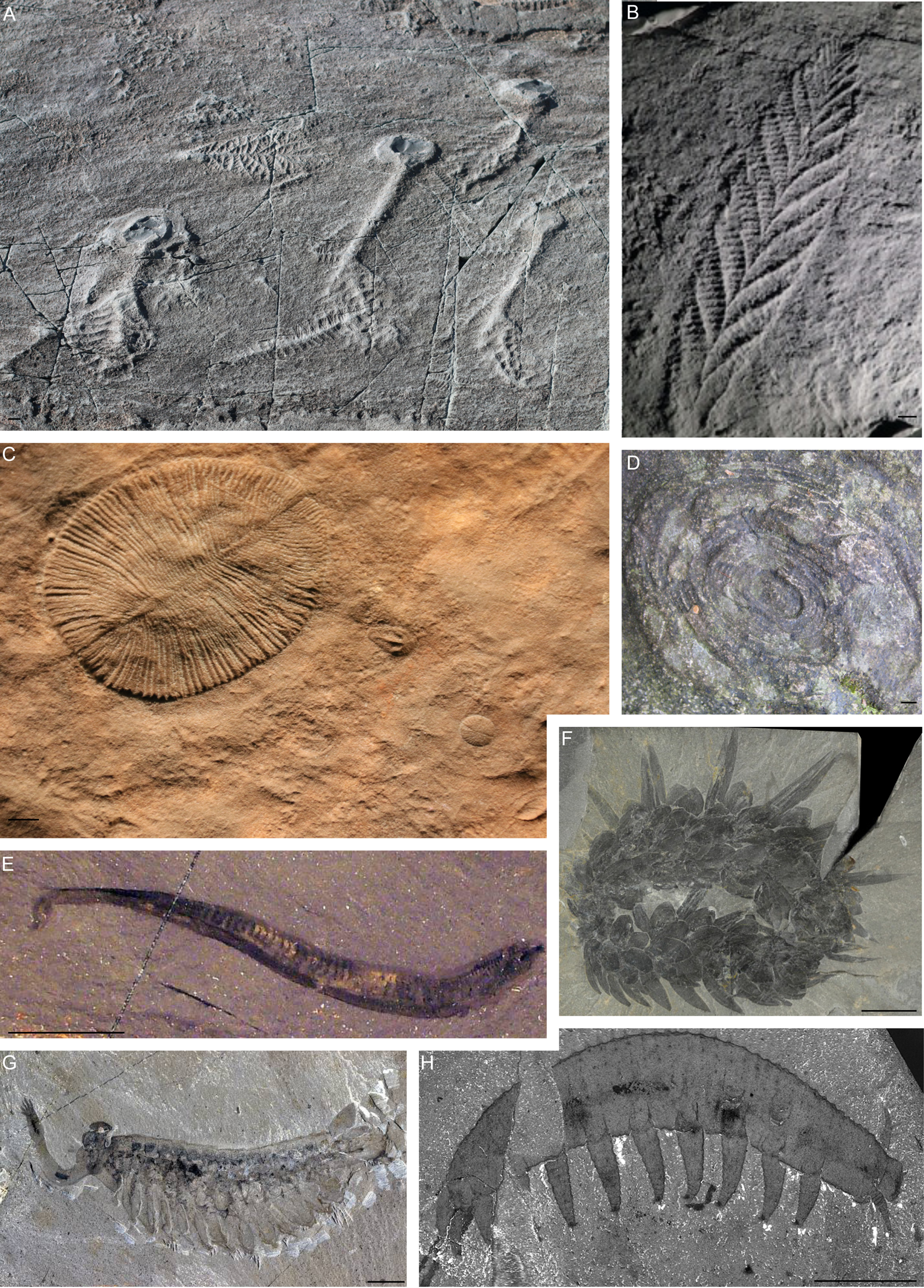
Figure 1. Iconic Ediacaran/Cambrian transition (ECT) organisms known in 1975. A, Mistaken Point E surface (~564 Ma), Newfoundland, Canada, showing Charniodiscus spinous, Charniodiscus arboreus, Beothukis mistakensis, and Fractofusus misrai. B, Charnia masoni holotype (LEIUG 2328), Bed B (~560 Ma) Charnwood Forest, U.K. Image credit: British Geological Survey. C, Dickinsonia costa (large specimen, left; and small specimen, right) and Parvancorina minchami (right middle) (~550 Ma), South Australia Museum. D, Cyclomedusa disk (~560 Ma), Charnwood Forest, U.K. A, C, D, Image credits: Emily G. Mitchell. E, Stem-group chordate Pikaia gracilens Walcott, 1911, Burgess Shale, Canada (Cambrian: Wuliuan) (Walcott Reference Walcott1911c). USNM PAL 83940B. Image courtesy of the Smithsonian Institution (CC0 license) (EZID:http://n2t.net/ark:/65665/m37ec4e117-c554-4a97-b352-5deb01b3081f). F, Stem-group mollusk Wiwaxia corrugata Walcott, 1911, Burgess Shale, Canada (Cambrian: Wuliuan) (Walcott Reference Walcott1911c). USNM PAL 198745. Image credit: Mark Florence. Image courtesy of the Smithsonian Institution (EZID: http://n2t.net/ark:/65665/m3038e2e32-c309-4da5-b28e-3f8cfdc8c941). G, Stem-group euarthropod Opabinia regalis Walcott, Reference Walcott1912, Burgess Shale, Canada (Cambrian: Wuliuan). USNM PAL 57683. Image credit: Han Zeng. Image courtesy of the Smithsonian Institution (EZID: http://n2t.net/ark:/65665/m31c224d68-28cb-465b-b42e-e565c31a44d1). H, Total-group ecdysozoan Aysheaia pedunculata Walcott, 1911, Burgess Shale, Canada (Cambrian: Wuliuan) (Walcott Reference Walcott1911c). USNM PAL365608. Image credit: Javier Ortega-Hernández. Scale bars for A–H, 10 mm.
Our understanding of the evolutionary relationships of Cambrian organisms has similarly shifted since the 1970s, greatly influenced by research effort on exceptionally preserved fossils. Detailed redescriptions of Burgess Shale animals first described by Walcott in the 1910s–1920s (e.g., Walcott Reference Walcott1911a,Reference Walcottb,Reference Walcottc, Reference Walcott1912, Reference Walcott1918, Reference Walcott1920), by Whittington and colleagues, alongside descriptions of new taxa, highlighted the unusual morphology of many Cambrian soft-bodied animals. While these fossil taxa were recognizable as metazoans, they were not always straightforward to place within extant classes or even phyla (Whittington Reference Whittington1971, Reference Whittington1975; Hughes Reference Hughes1975; Briggs Reference Briggs1976; Conway Morris Reference Conway Morris1976, Reference Conway Morris1977; Conway Morris and Robison Reference Conway Morris and Robison1982; Bruton and Whittington Reference Bruton and Whittington1983; Whittington and Briggs Reference Whittington and Briggs1985). Over the last 30 yr, the integration of these previously problematic Cambrian animals into cladograms (Briggs and Fortey Reference Briggs and Fortey1989; Budd Reference Budd1993, Reference Budd, Fortey and Thomas1998) and phylogenetic analyses including extant groups allowed these and other soft-bodied fossil taxa to be recognized as stem-group representatives of modern classes and phyla (Daley et al. Reference Daley, Budd, Caron, Edgecombe and Collins2009; Legg et al. Reference Legg, Sutton and Edgecombe2013; Smith and Caron Reference Smith and Caron2015; Park et al. Reference Park, Nielsen, Parry, Sørensen, Lee, Kihm and Ahn2024; Rahman and Zamora Reference Rahman and Zamora2024). These exceptional fossils have provided critical data for understanding the evolution of disparate body plans (Fig. 1E–H), although not always without controversy (Daley et al. Reference Daley, Antcliffe, Drage and Pates2018; Nanglu and Caron Reference Nanglu and Caron2018; Zhao et al. Reference Zhao, Vinther, Parry, Wei, Green, Pisani, Hou, Edgecombe and Cong2019; Nanglu et al. Reference Nanglu, Caron and Cameron2020a, Reference Nanglu, Lerosey-Aubril, Weaver and Ortega-Hernández2023; Kihm et al. Reference Kihm, Smith, Kim, Rho, Zhang, Liu and Park2023; Park et al. Reference Park, Nielsen, Parry, Sørensen, Lee, Kihm and Ahn2024).
Other substantial insights into the ECT since 1975 have been gained through the large increase in terms of number of localities and sites (Fig. 2), geographic distribution of these sites, and new data-collection strategies. These data have been leveraged to further our understanding in terms not only of ECT evolutionary relationships, but also their evolutionary drivers through interrogating the ecology and ecological relationships. Whereas specimens were historically often collected in isolation, so taken out of their population and community context, now this information is commonly kept intact, enabling us to reconstruct the interactions and ecology of these organisms on ecological, not only geological, timescales. These data are further leveraged by the vast improvements in computational and imaging power since 1975, enabling more detailed studies of organisms’ anatomy through photographic and scanning methods (Y. Liu et al. Reference Liu, Scholtz and Hou2015) and complex ecological statistical analyses (e.g., Clapham et al. Reference Clapham, Narbonne and Gehling2003; Mitchell et al. Reference Mitchell, Kenchington, Liu, Matthews and Butterfield2015), as well as new computationally intensive analyses, such as computational fluid dynamics (CFD; e.g., Rahman et al. Reference Rahman, Zamora, Falkingham and Phillips2015b) and finite element analysis (FEA; Bicknell et al. Reference Bicknell, Ledogar, Wroe, Gutzler, Watson and Paterson2018, Reference Bicknell, Holmes, Edgecombe, Losso, Ortega-Hernández, Wroe and Paterson2021) to investigate ECT organisms. This change in ECT paleontology is also mirrored in extant ecology, with a shift from largely descriptive and observational work to that which is more hypothesis testing–based and data driven (Anderson et al. Reference Anderson, Elsen, Hughes, Tonietto, Bletz, Gill and Holgerson2021).
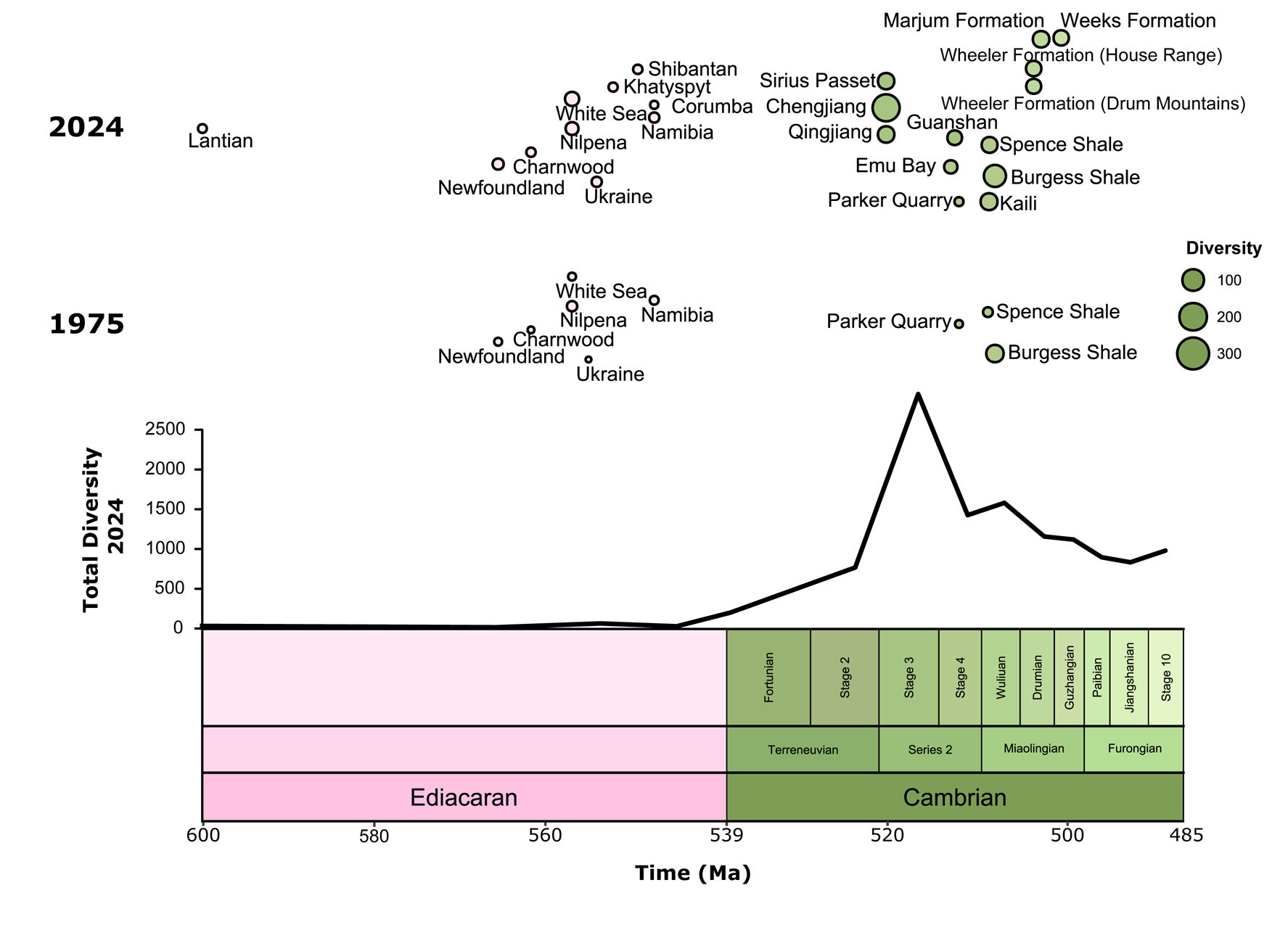
Figure 2. Key Ediacaran (pink) and Cambrian (green) Lagerstätten with their generic diversity in 1975 and 2024, and the total diversity from the Paleobiology Database (Na and Kiessling Reference Na and Kiessling2015) (black line).
Diversity Patterns over the ECT
The term “Cambrian explosion” conjures up images of low (or no) animal life before the Cambrian, followed by a rapid/exponential radiation in terms of taxonomic diversity. This pattern was visualized first in Sepkoski (Reference Sepkoski1978), who showed that the number of orders from the Ediacaran time period (then known as the Vendian) through the Cambrian followed an exponential curve that plateaued in the Cambrian Epoch 2. This historical pattern is seen across multiple groups, including trace fossils and commonly preserved fossil groups such as trilobites and brachiopods (e.g., Z. Zhang et al. Reference Zhang, Robson, Emig and Shu2008; Mángano and Buatois Reference Mángano and Buatois2017; Paterson et al. Reference Paterson, Edgecombe and Lee2019). Almost 50 yr later, diversity patterns appear much more complex, with an increase and subsequent decrease in the Ediacaran, followed by a large increase in the Cambrian at the base of Stage 3, with the rapid diversity increase that we associate with the Cambrian Explosion, which then plateaus (Na and Kiessling Reference Na and Kiessling2015). Yet these patterns are not robust across all taxa, environments, or metrics. Ichnodiversity follows the classic ECT increase (Mángano and Buatois Reference Mángano and Buatois2014), while some groups show a pulsed radiation through the ECT (Zhuravlev and Wood Reference Zhuravlev and Wood2018), and others begin to radiate at different times, some even starting their radiation in the Ordovician (Servais et al. Reference Servais, Cascales-Miñana, Harper, Lefebvre, Munnecke, Wang and Zhang2023).
With the variation in timings, and distribution of radiations across different groups, it is likely that a range of different processes drove the ECT. Indeed, the exponential increase in the diversity of a group follows a null model, with background rates of origination and extinction leading to crown-group radiation (Budd and Mann Reference Budd and Mann2020), so arguably requires no drivers. However, to describe the processes behind these patterns, we need to resolve which key mechanisms were operating, and even if the ECT diversification was driven purely by abiotic factors, we still need to understand how animals interacted with these abiotic factors, enabling diversification.
Ecology
Ecology is a very broad field that includes a range of different subtopics that consider organisms’ interactions on a variety of different scales. On an individual specimen level, organismal ecology describes aspects of the individual that reflect aspects of an organism's behavior, functional morphology, and/or life style. In a paleontological context, functional morphology, ichnology, and ecospace studies fall within organismal ecology.
Organismal ecology is the product of how different organisms interact with their local environment and other taxa, and these interactions and associations build up to form the network of interactions that form the ecosystem, the community ecology. Community ecology includes interactions such as competition and facilitation, as well as trophic interactions between organisms, that is, food webs. Community ecology can also include the interactions of organisms with their local habitat, and how that then impacts the organisms themselves. Population ecology also acts at a community level (communities are after all just collections of interacting populations), so includes analyses of population size distributions, for example, and can also include behavioral ecology, such as the interactions between predators and prey (e.g., the landscape of fear; Laundré et al. Reference Laundré, Hernández and Altendorf2001). In a paleontological context, community ecology can be inferred from organisms’ functional morphology (Haug et al. Reference Haug, Walosek, Maas, Liu and Haug2012), with hypotheses now often interrogated with computational methods (Rahman et al. Reference Rahman, Darroch, Racicot and Laflamme2015a; Bicknell et al. Reference Bicknell, Holmes, Edgecombe, Losso, Ortega-Hernández, Wroe and Paterson2021); gut contents and morphology (trophic ecology and food webs) (Butterfield Reference Butterfield2002; Vannier and Chen Reference Vannier and Chen2005; Vannier Reference Vannier2012; Vannier et al. Reference Vannier, Liu, Lerosey-Aubril, Vinther and Daley2014; Strang et al. Reference Strang, Armstrong and Harper2016; Peel Reference Peel2017; Park et al. Reference Park, Nielsen, Parry, Sørensen, Lee, Kihm and Ahn2024); trace fossils, including predation traces (Seilacher et al. Reference Seilacher, Buatois and Gabriela Mángano2005; Jensen et al. Reference Jensen, Droser, Gehling, Xiao and Kaufman2006; Chen et al. Reference Chen, Zhou, Meyer, Xiang, Schiffbauer, Yuan and Xiao2013; Bicknell and Paterson Reference Bicknell and Paterson2018; Mángano and Buatois Reference Mángano and Buatois2020; Bicknell et al. Reference Bicknell, Holmes, Pates, García-Bellido and Paterson2022); and with exceptional preservation, from quantitative analyses of census populations or communities (Wood et al. Reference Wood, Dalrymple, Narbonne, Gehling and Clapham2003; Darroch et al. Reference Darroch, Laflamme and Clapham2013; Zhao et al. Reference Zhao, Caron, Bottjer, Hu, Yin and Zhu2014; O'Brien and Caron Reference O'Brien and Caron2016; Mitchell et al. Reference Mitchell, Harris, Kenchington, Vixseboxse, Roberts, Clark, Dennis, Liu and Wilby2019; Nanglu et al. Reference Nanglu, Caron and Gaines2020b).
The ecological mechanisms that shape and drive evolutionary patterns act at and within the community scale, over time leading to changes in organismal ecology and to broad patterns across space and time (between-community ecology, or macroecology). Comparisons between different communities encompasses metacommunity (and metapopulation) ecology, landscape ecology, biogeography, global ecology, movement ecology, and macroecology. As such, diversity trends over geological and evolutionary timescales are the result of organism, community, and global ecological interactions of organisms with one another and their environment.
To understand the long-term diversity and evolutionary patterns over the ECT, this review outlines our current knowledge in ECT organismal ecology, community ecology, and macroecology, and how these different scales of interactions feed into and influence the broad-scale diversity patterns we observe over the ECT. Of course, preservational biases can have huge impacts on paleoecological studies (Nanglu and Cullen Reference Nanglu and Cullen2023). However, the exceptional preservation for many fossil localities spanning the ECT means that analytical techniques normally only applicable for extant systems can be used. The preservation of Ediacaran macrofossil communities is commonly in-situ, creating near-census preservation, with burial under volcanic ash flow (Benus et al. Reference Benus, Landing, Narbonne and Myrow1988; Wood et al. Reference Wood, Dalrymple, Narbonne, Gehling and Clapham2003; Noble et al. Reference Noble, Condon, Carney, Wilby, Pharaoh and Ford2015) and pervasive microbial mat coverage of the seafloor leading to widespread in situ preservation of benthic communities between 572 and 550 Ma (Gehling Reference Gehling1999; Grazhdankin Reference Grazhdankin2004; Fedonkin et al. Reference Fedonkin, Simonetta and Ivantsov2007; Laflamme et al. Reference Laflamme, Schiffbauer, Narbonne and Briggs2011; Darroch et al. Reference Darroch, Laflamme, Schiffbauer and Briggs2012; Tarhan et al. Reference Tarhan, Droser and Gehling2015, Reference Tarhan, Droser, Gehling and Dzaugis2017). The terminal Ediacaran (550–539 Ma) also has exceptional soft-bodied preservation, but many of these sites contained specimens that were transported in mass flow events (Hall et al. Reference Hall, Kaufman, Vickers-Rich, Ivantsov, Trusler, Linnemann and Hofmann2013; Meyer et al. Reference Meyer, Elliott, Schiffbauer, Hall, Hoffman, Schneider, Vickers-Rich and Xiao2014), as well as biomineralized reefs preserved within carbonate platforms (Hofmann and Mountjoy Reference Hofmann and Mountjoy2001; Grotzinger et al. Reference Grotzinger, Adams and Schroder2005; Warren et al. Reference Warren, Fairchild, Gaucher, Boggiani, Poiré, Anelli and Inchausti2011).
Dozens of deposits with exceptional soft-bodied preservation are known from the Cambrian (Gaines Reference Gaines2014; Muscente et al. Reference Muscente, Schiffbauer, Broce, Laflamme, O'Donnell, Boag, Meyer and et al2017). These provide census-level data for deposits that have not undergone significant transport, as well as a rich record of the anatomy of animals completely lacking hard parts, as well as soft tissues such as appendages, nervous tissues, and guts not usually preserved in the shelly fossil record (Gaines Reference Gaines2014), just as for Ediacaran deposits with similar preservation. However the oldest of these Cambrian deposits are known from Stage 3 (Fig. 2), leaving a large gap in the soft-bodied fossil record spanning the entire Terreneuvian. Insights into the organismal ecology of Terreneuvian animals can be gleaned from trace fossils, for which a continuous record exists (Seilacher et al. Reference Seilacher, Buatois and Gabriela Mángano2005; Mángano and Buatois Reference Mángano and Buatois2017; Buatois et al. Reference Buatois, Almond, Mángano, Jensen and Germs2018, Reference Buatois, Mángano, Minter, Zhou, Wisshak, Wilson and Olea2020), as well as the small shelly and small carbonaceous fossil records (Butterfield and Harvey Reference Butterfield and Harvey2012; Kouchinsky et al. Reference Kouchinsky, Bengtson, Runnegar, Skovsted, Steiner and Vendrasco2012); however, the lack of exceptional deposits precludes community ecology studies. Compared with the Ediacaran, exceptional soft-bodied deposits from the Cambrian Series 2 and 3 provide a more heavily sampled interval, including multiple deposits of similar age from geographically constrained areas (e.g., Burgess Shale and nearby localities [Collins et al. Reference Collins, Briggs and Conway Morris1983; Caron et al. Reference Caron, Gaines, Mángano, Streng and Daley2010; Nanglu et al. Reference Nanglu, Caron and Gaines2020b]; the Great Basin of the United States [Lieberman Reference Lieberman2003; Lerosey-Aubril et al. Reference Lerosey-Aubril, Gaines, Hegna, Ortega-Hernández, Van Roy, Kier and Bonino2018, Reference Lerosey-Aubril, Kimmig, Pates, Skabelund, Weug and Ortega-Hernández2020; Kimmig et al. Reference Kimmig, Strotz, Kimmig, Egenhoff and Lieberman2019]; and South China [Steiner et al. Reference Steiner, Zhu, Zhao and Erdtmann2005; X. Zhang et al. Reference Zhang, Liu and Zhao2008; Hou et al. Reference Hou, Siveter, Siveter, Aldridge, Cong, Gabbot, Ma, Purnell and Williams2017; Fu et al. Reference Fu, Tong, Dai, Liu, Yang, Zhang, Cui, Li, Yun, Wu, Sun, Liu, Pei, Gaines and Zhang2019]). Just as for soft-bodied preservation in the Ediacaran and post-Cambrian, these deposits provide a rich record important for our understanding of organismal ecology. Compared with Ediacaran macrofossils, Cambrian macrofossils are generally more readily identifiable to the phylum or class level, facilitating a more straightforward comparison with modern analogues to infer aspects of their ecology (see “Organismal Ecology”). Importantly, alongside the temporal gap in the Terreneuvian, there are geographic limitations to our understanding of soft-bodied Cambrian animals. Most pressingly, there is a paucity of deposits preserving soft tissues from high latitudes, and those that are known do not have the same fidelity or abundance of fossils as the best low-latitude Lagerstätten (they are Tier 3, rather than Tier 1; sensu Gaines Reference Gaines2014). Thus comparable preservation modes and types facilitate comparison of organism, community, and macroecology across the ECT, albeit with some temporal and geographic gaps and differences in sampling intensity.
Organismal Ecology
Organismal ecology, or autecology, focuses on the adaptations that an individual organism has in response to its biotic and abiotic environment. These changes include morphological, physiological, and behavioral adaptations. Insights into autecology can be gained through functional ecology analyses such as CFD and FEA to infer trophic relationships (e.g., Gibson et al. Reference Gibson, Furbish, Rahman, Schmeeckle, Laflamme and Darroch2020; Bicknell et al. Reference Bicknell, Holmes, Edgecombe, Losso, Ortega-Hernández, Wroe and Paterson2021) and trace fossil analyses to understand habitat and feeding modes (Seilacher et al. Reference Seilacher, Buatois and Gabriela Mángano2005; Jensen et al. Reference Jensen, Droser, Gehling, Xiao and Kaufman2006; Mángano and Buatois Reference Mángano and Buatois2020). These analyses can also be visualized in ecospace, whereby different axes are used to quantify the different niches in which organisms can live and how this niche or ecospace occupation changes through time (Bambach Reference Bambach, Tevesz and McCall1983). For marine organisms, the axes used are normally: extent of mobility, position relative to the substrate, and feeding modes (Bambach et al. Reference Bambach, Bush and Erwin2007). Body and trace fossil data are used to determine whether an area of ecospace (or “Bambach cube”) is occupied for a particular time slice. During the ECT, ecospace occupation changed dramatically between the Ediacaran and Cambrian, with the advent of animals, mobility, and many new feeding modes. In the Ediacaran, 12 Bambach cubes were occupied, with 30 in the Cambrian, and 92 in the modern (Bambach et al. Reference Bambach, Bush and Erwin2007; Bush et al. Reference Bush, Bambach, Erwin, Laflamme, Schiffbauer and Dornbos2011).
Marine Animal Forests: Lantian and Avalon Assemblages (Pre-558 Ma)
The first putative animals were sessile, benthic organisms that lived attached to the seafloor. The oldest are putative cnidarians from the Lantian biota, China (Wan et al. Reference Wan, Yuan, Chen, Guan, Pang, Tang and Xiao2016) (up to 600 Ma), and widespread, abundant, large, complex fossils found predominantly in Newfoundland, Canada, and Charnwood Forest, U.K. (572–560 Ma), known as the Avalon assemblage (Waggoner Reference Waggoner2003; Noble et al. Reference Noble, Condon, Carney, Wilby, Pharaoh and Ford2015; Matthews et al. Reference Matthews, Liu, Yang, McIlroy, Levell and Condon2021). Avalon communities are dominated by rangeomorphs, a group of fractally branching organisms unique to the Ediacaran (Narbonne Reference Narbonne2004; Brasier et al. Reference Brasier, Antcliffe and Liu2012; Hoyal Cuthill and Conway Morris Reference Hoyal Cuthill and Conway Morris2014), with large numbers of arboreomorphs (Laflamme and Narbonne Reference Laflamme and Narbonne2008) also present (Clapham et al. Reference Clapham, Narbonne and Gehling2003; Wilby et al. Reference Wilby, Carney and Howe2011). Phylogenetic analyses have resolved rangeomorphs as eumetazoans (Dunn et al. Reference Dunn, Liu, Grazhdankin, Vixseboxse, Flannery-Sutherland, Green, Harris, Wilby and Donoghue2021) with other taxonomically resolved taxa, including crown-group cnidarians (Liu et al. Reference Liu, Matthews, Menon, McIlroy and Brasier2014; Dunn et al. Reference Dunn, Kenchington, Parry, Clark, Kendall and Wilby2022) and putative sponges (Sperling et al. Reference Sperling, Peterson and Laflamme2011; Suarez and Leys Reference Suarez and Leys2022). In these early Ediacaran assemblages, namely the Lantian and Avalon, there is no evidence for mobility within animal communities. Putative cnidarian trails have been found in nearby strata (Liu et al. Reference Liu, Mcllroy and Brasier2010), but these may instead be microbial aggregates (Mariotti et al. Reference Mariotti, Pruss, Ai, Perron and Bosak2016; Warren et al. Reference Warren, Buatois, Mángano, Simões, Santos, Poiré, Riccomini and Assine2020).
Feeding modes for these sessile, benthic organisms are hard to establish, because comparisons with extant sessile benthic organisms such as sponges and sea pens are limited (Antcliffe and Brasier Reference Antcliffe and Brasier2007; Laflamme et al. Reference Laflamme, Xiao and Kowalewski2009). The widespread presence of rangeomorphs in deep-water strata below the photic zone demonstrates these sessile organisms were not algae or photosynthetic (Benus et al. Reference Benus, Landing, Narbonne and Myrow1988; Wood et al. Reference Wood, Dalrymple, Narbonne, Gehling and Clapham2003). Instead, the fractal-style branching of rangeomorphs (Hoyal Cuthill and Conway Morris Reference Hoyal Cuthill and Conway Morris2014) suggests utilization of resources from the water column (Narbonne Reference Narbonne2004, Reference Narbonne2005). This feeding could be through suspension or filter feeding, as is seen with extant sessile benthic animals, or osmotrophy like giant bacteria and other microorganisms. As rangeomorphs exhibit surface area to volume ratios similar to those of extant osmotrophs, and no feeding apparatus for suspension or filter feeding is preserved at a micron-level scale, this unusual mode of feeding was thought likely (Laflamme et al. Reference Laflamme, Xiao and Kowalewski2009). However, suspension feeding is now considered the most likely mode for rangeomorphs, as osmotrophy could not occur at the high Raleigh numbers and advective fluid dynamics that operate at macroscopic scales (Butterfield Reference Butterfield2022). Furthermore, if the filter/suspension-feeding apparatuses were sufficiently small (<8 microns), they might not have been preserved (Riisgård and Manríquez Reference Riisgård and Manríquez1997), especially due to the preferential loss of labile tissue (Gibson et al. Reference Gibson, Schiffbauer and Darroch2018). Finally, rangeomorphs display growth patterns that put them within Eumetazoans (Dunn et al. Reference Dunn, Liu, Grazhdankin, Vixseboxse, Flannery-Sutherland, Green, Harris, Wilby and Donoghue2021), where osmotrophy is unknown. CFD simulations have lent further support for suspension feeding in Ediacaran organisms. Tribrachidium and Ernietta are now interpreted as a suspension feeders (Rahman et al. Reference Rahman, Darroch, Racicot and Laflamme2015a; Gibson et al. Reference Gibson, Darroch, Maloney and Laflamme2021), whereas the rangeomorph Pectinifrons shows feeding modes inconsistent with suspension feeding, osmotrophy, and carnivory (e.g., the carnivorous sponges Lyra; Darroch et al. Reference Darroch, Gutarra, Masaki, Olaru, Gibson, Dunn, Mitchell, Racicot, Burzynski and Rahman2023a).
The passive uptake of food from the water (by osmotrophy or passive suspension feeding) can be supplemented by making the most of canopy flow (Ghisalberti et al. Reference Ghisalberti, Gold, Laflamme, Clapham, Narbonne, Summons, Johnston and Jacobs2014), but these are not active feeding modes, as they do not expend energy in order to gain further energy. This contrasts with filter feeding, the active pumping of water through feeding apparatuses. Before 559 Ma, the putative sponge Thectardis (572 Ma) is the only organism for which filter feeding has been proposed. Support for this feeding mode is based on length/width ratios and comparisons of the morphology of Cambrian and extant sponge oscula and surface area of flattened specimens with those of Thectardis (Sperling et al. Reference Sperling, Peterson and Laflamme2011). As such, before 559 Ma, known life modes are limited to nonmobile, passive feeders attached to the substrate (Bush et al. Reference Bush, Bambach, Erwin, Laflamme, Schiffbauer and Dornbos2011).
Origins of Mobility and Morphological Diversification: White Sea Assemblage (558–550 Ma)
Taxonomic, morphological, and functional diversity increases dramatically ~555 Ma during the Avalon explosion or Ediacaran second wave (Shen et al. Reference Shen, Dong, Xiao and Kowalewski2008; Droser and Gehling Reference Droser and Gehling2015; Evans et al. Reference Evans, Diamond, Droser and Lyons2018), with key localities for this time period in South Australia and the White Sea Region, Russia (Boag et al. Reference Boag, Darroch and Laflamme2016). In contrast to the deep-water Avalon assemblage, the White Sea assemblage records a shallow-water environment (Grazhdankin Reference Grazhdankin2004; Tarhan et al. Reference Tarhan, Droser and Gehling2015). Phototrophs in the form of macroalgae are present within some communities (Wang et al. Reference Wang, Wang, Du and Wang2014; Droser and Gehling Reference Droser and Gehling2015; Xiao et al. Reference Xiao, Gehling, Evans, Hughes and Droser2020), as are the dominant Avalon taxa, rangeomorphs and arboreomorphs, but in reduced diversity and abundance (Evans et al. Reference Evans, Diamond, Droser and Lyons2018). Communities of the tubular organism Funisia reach extremely high densities—more than 6000 specimens/m2 (Suprenaut et al. Reference Suprenaut, Gehling and Droser2020), likely due to reproductive spawning akin to that of corals (Droser and Gehling Reference Droser and Gehling2008). Besides these frondose organisms, the White Sea assemblage includes mat-sticking organisms with a range of symmetries not present today, such as triradial and tetraradial (Xiao and Laflamme Reference Xiao and Laflamme2009), as well as facultative mobile organisms in their colonization life stage (Darroch et al. Reference Darroch, Rahman, Gibson, Racicot and Laflamme2017; Coutts et al. Reference Coutts, Bradshaw, García-Bellido and Gehling2018). Tissues diversified, with hard tissues functioning as structural supports in the form of long (up to 37 cm) spicules in association with a cone (Serezhnikova and Ivantsov Reference Serezhnikova and Ivantsov2007; Clites et al. Reference Clites, Droser and Gehling2012). During this Ediacaran second wave, there is also a diversification of bilaterians (Evans et al. Reference Evans, Diamond, Droser and Lyons2018), including mobile taxa (Glaessner Reference Glaessner1959; Fedonkin and Waggoner Reference Fedonkin and Waggoner1997) and a corresponding increase in ichnodiversity (Mángano and Buatois Reference Mángano and Buatois2020). Some bilaterians are interpreted to have burrowed in and out of the microbial mats (Xiao et al. Reference Xiao, Chen, Zhou and Yuan2019), underneath them (Ivantsov et al. Reference Ivantsov, Nagovitsyn and Zakrevskaya2019), and through them (Evans et al. Reference Evans, Gehling and Droser2019). It is also around 555 Ma that within-substrate habitats become well occupied with erniettomorphs (Glaessner Reference Glaessner1959; Ivantsov et al. Reference Ivantsov, Narbonne, Dzaugis, Greentree and Vickers-Rich2016). Mat grazing by Kimberella (Fedonkin and Waggoner Reference Fedonkin and Waggoner1997) is complemented by trails of Yorgia and Dickinsonia that suggest they fed directly on the microbial mat (Ivantsov and Malakhovskaya Reference Ivantsov and Malakhovskaya2002; Gehling et al. Reference Gehling, Droser, Jensen and Briggs2005; Ivantsov et al. Reference Ivantsov, Nagovitsyn and Zakrevskaya2019). Trails that wind underneath decaying body fossils suggest the first occurrences of scavenging (Gehling and Droser Reference Gehling and Droser2018). The irregular morphology of Attenborites, in contrast to most other Ediacaran taxa, suggests that it settled out of the water column and was pelagic (Droser et al. Reference Droser, Evans, Dzaugis, Hughes and Gehling2020). Thus, the White Sea assemblage records a radiation of life habitats, feeding, and mobility.
Bridging the White Sea assemblage (558–550 Ma) and the terminal Ediacaran assemblage, the Nama (550–539 Ma), is the Shibantan assemblage (551–543 Ma) (Condon et al. Reference Condon, Zhu, Bowring, Wang, Yang and Jin2005; Huang et al. Reference Huang, Chen, Ding, Zhou and Zhang2020). In common with the South Australian and Russian sites, Shibantan communities include rangeomorphs, arboreomorphs, bilaterians, and macroalgae, with an increased abundance of ichnofossils as well as erniettomorphs (Xiao et al. Reference Xiao, Chen, Pang, Zhou and Yuan2021). What is notably different between the White Sea and South Australia localities and the Shibantan is the abundance of trace fossils, for which the Shibantan has a consistently high proportion of trace fossils that dominate many communities (Xiao et al. Reference Xiao, Chen, Pang, Zhou and Yuan2021), some in association with complex body fossils of bilobed, segmented bilaterians (Chen et al. Reference Chen, Zhou, Yuan and Xiao2019).
Advent of Biomineralization: Nama Assemblage and the Terminal Ediacaran (550–539 Ma)
Crucially, the Nama assemblage is where abundant biomineralizers are first found forming metazoan reefs (Hofmann and Mountjoy Reference Hofmann and Mountjoy2001; Wood et al. Reference Wood, Dalrymple, Narbonne, Gehling and Clapham2003; Cortijo et al. Reference Cortijo, Martí Mus, Jensen and Palacios2010; Warren et al. Reference Warren, Fairchild, Gaucher, Boggiani, Poiré, Anelli and Inchausti2011; Becker-Kerber et al. Reference Becker-Kerber, Pacheco, Rudnitzki, Galante, Rodrigues and Leme2017). These reefs capture the first evidence of macropredation in the form of drill holes in the reef-building Cloudina (Hua et al. Reference Hua, Pratt and Zhang2003). As well as reef complexity, increasing behavioral complexity is observed within ichnodiversity in the form of more complex and 3D burrows of treptichnids (Jensen et al. Reference Jensen, Saylor, Gehling and Germs2000), as well as evidence of sediment bulldozing Parapsammichnites (Buatois et al. Reference Buatois, Almond, Mángano, Jensen and Germs2018). Soft-bodied organisms such as rangeomorphs and erniettomorphs lived attached to and within the substrate (Pflug Reference Pflug1966; Meyer et al. Reference Meyer, Elliott, Schiffbauer, Hall, Hoffman, Schneider, Vickers-Rich and Xiao2014), but mat stickers are not found, and soft-bodied mobile taxa are not preserved (Boag et al. Reference Boag, Darroch and Laflamme2016). Thus, at the end of the Ediacaran, we have occupation of pelagic, erect, surficial, semi-infaunal, and shallow infaunal habitat occupation, the full complement of feeding strategies, from suspension feeding, deposit feeding, mining, grazing, and predatory modes with a range of mobility from sessile attached to fully mobile.
Digging Deeper, Building Bigger: The Terreneuvian (539–521 Ma)
Microbial mats persisted into the Terreneuvian, with evidence for mat grazers, scratchers, and miners continuing into the Fortunian (Buatois et al. Reference Buatois, Narbonne, Mángano, Carmona and Myrow2014). Over the 20 Myr of the Terreneuvian, the maximum depth of burrows increased dramatically (from ~6 to 100 cm), and a range of hitherto unrecorded behaviors and structures can be observed in the ichnofossil record, including penetrative feeding traces, farming burrows, and domiciles for suspension feeders (Buatois et al. Reference Buatois, Mángano, Minter, Zhou, Wisshak, Wilson and Olea2020). Archaeocyath reefs of active suspension feeders emerged during the early Terreneuvian, ca. 535 Ma, initially as small patch reefs in shallow-water environments of the Siberian Platform (Zhuravlev et al. Reference Zhuravlev, Naimark and Wood2015; Antcliffe et al. Reference Antcliffe, Jessop and Daley2019; Gibson et al. Reference Gibson, Chipman, Attanasio, Qureshi, Darroch, Rahman and Laflamme2023). The Terreneuvian also records the first appearance of a new group of metazoan predators—chaetognaths—into the pelagic realm (Szaniawski Reference Szaniawski1983, Reference Szaniawski2002; Vannier Reference Vannier, Williams, Haywood, Gregory and Schmidt2007) and the earliest evidence for durophagous predation (Bengtson Reference Bengtson1968; Conway Morris and Bengtson Reference Conway Morris and Bengtson1994), while the number of predatory drill holes—and diversity of groups with them—increased (Bicknell and Paterson Reference Bicknell and Paterson2018; Vinn Reference Vinn2018).
Radiation of Feeding Modes and Mobility: Cambrian Epoch 2 and Beyond (Post-521 Ma)
Ichnodiversity, disparity, and the modes of life represented in the trace fossil record increased again in Cambrian Epoch 2, across siliciclastic and carbonate environments (Buatois et al. Reference Buatois, Mángano, Minter, Zhou, Wisshak, Wilson and Olea2020), complemented by a huge array of morphological innovations across the sensory, trophic, and movement spectra, as well as an overall increase in the maximum size of animals. Archaeocyath diversity and geographic spread increased, and meter-scale reefs appear in the fossil record, with different biohermal communities and structures reflecting the latitudes and depths of their locations (Gandin and Debrenne Reference Gandin and Debrenne2010; Zhuravlev and Wood Reference Zhuravlev and Wood2018).
The widespread appearance of sensory organs (antennae, eyes, palps) in animals from Cambrian deposits of Epoch 2 and younger, and corresponding increases in brain complexity evidenced by exceptional fossils of nervous tissues (Ma et al. Reference Ma, Hou, Edgecombe and Strausfeld2012b; Tanaka et al. Reference Tanaka, Hou, Ma, Edgecombe and Strausfeld2013; Cong et al. Reference Cong, Ma, Hou, Edgecombe and Strausfeld2014; Ortega-Hernández et al. Reference Ortega-Hernández, Lerosey-Aubril and Pates2019, Reference Ortega-Hernández, Lerosey-Aubril, Losso and Weaver2022; Parry and Caron Reference Parry and Caron2019; Parry et al. Reference Parry, Lerosey-Aubril, Weaver and Ortega-Hernández2021; Wolfe and Ortega-Hernández Reference Wolfe and Ortega-Hernández2021) has been termed the “Cambrian information revolution” (Plotnick et al. Reference Plotnick, Dornbos and Chen2010), due to the sharp increase in the ability of organisms to sense and process their environment. Eyes are best known, most abundant, and most disparate in euarthropods (Zhao et al. Reference Zhao, Bottjer, Hu, Yin and Zhu2013), but originated across a range of phyla in animals occupying very different niches. The oldest eyes in the fossil record—focal apposition eyes in the trilobite Schmidtiellus—are from the base of Stage 3 (Schoenemann et al. Reference Schoenemann, Pärnaste and Clarkson2017). Some total-group euarthropod compound eyes contained thousands to tens of thousands of lenses (Zhao et al. Reference Zhao, Bottjer, Hu, Yin and Zhu2013; Paterson et al. Reference Paterson, Edgecombe and García-Bellido2020), with variation in eye morphology indicating specializations for different depths and feeding ecologies, including mesopelagic suspension feeders (Schoenemann and Clarkson Reference Schoenemann and Clarkson2011; Paterson et al. Reference Paterson, Edgecombe and García-Bellido2020). Camera-type eyes have been reported in the mollusk Nectocaris (Smith Reference Smith2013), with simpler eyes and eye spots recorded in annelids (J. Zhao et al. Reference Zhao, Li and Selden2023), chordates (Shu et al. Reference Shu, Conway Morris, Han, Zhang, Yasui, Janvier and Chen2003), lobopodians (Ma et al. Reference Ma, Hou, Aldridge, Siveter, Siveter, Gabbott, Purnell, Parker and Edgecombe2012a), and even priapulids (Schoenemann Reference Schoenemann2006). Beyond eyes, metazoan sensory structures first recorded at this time include antennulae and antennae in total-group euarthropods (Ma et al. Reference Ma, Hou, Edgecombe and Strausfeld2012b; Ortega-Hernández et al. Reference Ortega-Hernández, Yang and Zhang2018; Zhai et al. Reference Zhai, Ortega-Hernández, Wolfe, Hou, Cao and Liu2019a, Reference Zhai, Williams, Siveter, Harvey, Sansom, Gabbott and Siveter2019b), and annelid palps (Eibye-Jacobsen Reference Eibye-Jacobsen2004; J. Liu et al. Reference Liu, Ou, Han, Li, Wu, Jiao and He2015; Nanglu and Caron Reference Nanglu and Caron2018; Parry and Caron Reference Parry and Caron2019).
The first large active swimmers are also found in Cambrian Stage 3 deposits, adding multiple trophic levels to the pelagic realm. The largest swimming animals in Cambrian oceans were stem-group euarthropods such as radiodonts, predators that could reach up to 1 m in length at this time (Potin and Daley Reference Potin and Daley2023; Wu et al. Reference Wu, Pates, Pauly, Zhang and Fu2024), and stem-group chaetognaths, reaching up to about 30 cm (Park et al. Reference Park, Nielsen, Parry, Sørensen, Lee, Kihm and Ahn2024). Adaptations for active swimming can be seen across a range of groups, including specialized swimming flaps, fins, and streamlined fusiform bodies (Whittington and Briggs Reference Whittington and Briggs1985; Chen et al. Reference Chen, Dzik, Edgecombe, Ramsköld and Zhou1995; Shu et al. Reference Shu, Luo, Conway Morris, Zhang, Hu, Chen, Han, Zhu, Li and Chen1999; Vannier and Chen Reference Vannier and Chen2000; Usami Reference Usami2006; Daley et al. Reference Daley, Budd, Caron, Edgecombe and Collins2009; Vannier et al. Reference Vannier, García-Bellido, Hu and Chen2009; Conway Morris and Caron Reference Conway Morris and Caron2012; Smith Reference Smith2013; Daley and Edgecombe Reference Daley and Edgecombe2014; J. Liu et al. Reference Liu, Ou, Han, Li, Wu, Jiao and He2015; Lerosey-Aubril and Pates Reference Lerosey-Aubril and Pates2018; Izquierdo-López and Caron Reference Izquierdo-López and Caron2019; Pates et al. Reference Pates, Daley, Legg and Rahman2021a; Lerosey-Aubril and Ortega-Hernández Reference Lerosey-Aubril and Ortega-Hernández2022; Potin and Daley Reference Potin and Daley2023). Closer to the seafloor, specialized walking appendages facilitated more rapid movement for vagrant epifaunal animals (Minter et al. Reference Minter, Mángano and Caron2011).
The Cambrian Epoch 2 also saw increased specialization in feeding appendages across groups, in particular within euarthropods. Multisegmented appendages for raptorial predation (Briggs Reference Briggs1979; Daley and Budd Reference Daley and Budd2010; Daley and Edgecombe Reference Daley and Edgecombe2014; Jiao et al. Reference Jiao, Pates, Lerosey-Aubril, Ortega-Hernández, Yang, Lan and Zhang2021; Potin and Daley Reference Potin and Daley2023), sediment sifting and sweep feeding (Daley et al. Reference Daley, Budd and Caron2013; Yang et al. Reference Yang, Ortega-Hernández, Butterfield and Zhang2013; Moysiuk and Caron Reference Moysiuk and Caron2019; Potin and Daley Reference Potin and Daley2023), and durophagy (Pratt Reference Pratt1998; Haug et al. Reference Haug, Walosek, Maas, Liu and Haug2012) are found across a number of total-group euarthropods, complemented by robust gnathobases, gnathobase-like structures, mandibles, and mandible-like structures (Harvey and Butterfield Reference Harvey and Butterfield2008, Reference Harvey and Butterfield2022; Zacaï et al. Reference Zacaï, Vannier and Lerosey-Aubril2016; Aria and Caron Reference Aria and Caron2017a,Reference Aria and Caronb; Cong et al. Reference Cong, Daley, Edgecombe and Hou2017, Reference Cong, Edgecombe, Daley, Guo, Pates and Hou2018; Vannier et al. Reference Vannier, Aria, Taylor and Caron2018; Yang et al. Reference Yang, Ortega-Hernández, Legg, Lan, Hou and Zhang2018; Bicknell et al. Reference Bicknell, Holmes, Edgecombe, Losso, Ortega-Hernández, Wroe and Paterson2021; Moysiuk and Caron Reference Moysiuk and Caron2021).
Computational fluid dynamics analyses support both active pharangeal filter feeding and passive suspension feeding in Cambrian echinoderms (Rahman et al. Reference Rahman, Zamora, Falkingham and Phillips2015b, Reference Rahman, O'Shea, Lautenschlager and Zamora2020), while numerous groups display feeding apparatuses with rows of finely spaced spines, setae, cilia, or comb-like elements, suitable for suspension or filter feeding. These include total-group euarthropods (Vinther et al. Reference Vinther, Stein, Longrich and Harper2014), luolishaniids (Ma et al. Reference Ma, Hou and Bergström2009; Yang et al. Reference Yang, Ortega-Hernández, Gerber, Butterfield, Hou, Lan and Zhang2015; Caron and Aria Reference Caron and Aria2017; Howard et al. Reference Howard, Hou, Edgecombe, Salge, Shi and Ma2020), hemichordates (Nanglu et al. Reference Nanglu, Caron, Conway Morris and Cameron2016, Reference Nanglu, Caron and Cameron2020a), and the problematica Siphausauctum (O'Brien and Caron Reference O'Brien and Caron2012) and dinomischids (Ou et al. Reference Ou, Han, Zhang, Shu, Sun and Mayer2017; Y. Zhao et al. Reference Zhao, Hou and Cong2023). This time period also sees the first documentations of brood care and extended parental care in the fossil record (Caron and Vannier Reference Caron and Vannier2016; Fu et al. Reference Fu, Ortega-Hernández, Daley, Zhang and Shu2018; Ou et al. Reference Ou, Vannier, Yang, Chen, Mai, Shu, Han, Fu, Wang and Mayer2020; Ma et al. Reference Ma, Pates, Wu, Lin, Liu, Wu, Zhang and Fu2023), demonstrating a diversification of behavioral ecology, as well as trophic ecology.
Summary of Organismal Ecology over the ECT
A fundamental shift in organismal ecology occurred over the ECT. Before the Ediacaran, macro-organisms were exclusively primary producers, such as algae, and all heterotrophic organisms were microbial. The earliest animals still fed, like their microbial counterparts, on microscopic life such as plankton and dissolved organic carbon (DOC), particulate organic matter (POM) and particulate organic carbon (POC) in the water column. Key innovations such as large body size, coupled with differentiated tissues likely enabled further diversification of feeding modes, and the expansion to grazing, scavenging, and indeed predation. Coupled to this trophic diversification was the advent of mobility, facilitating access to nutrients that were becoming increasingly concentrated on the seafloor. Yet, despite the origination of mobility and predation in the Ediacaran, it is not until Cambrian Epoch 2 that specializations really start to intensify. The specializations and variation in form of feeding appendages, sensory equipment, movement types, and burrowing employed by a diverse range of animal groups set the Cambrian Epoch 2 apart from the Terreneuvian and Ediacaran and laid the groundwork for the rest of the Phanerozoic.
Community Ecology
Dominant Ecological Modes within Communities
The simplest way to consider the community ecology of an ecosystem is in terms of the relative abundance of ecological modes—that is, what is the primary niche of the community, and how are niches distributed within it? The oldest Ediacaran communities, the Lantian assemblage (~600 Ma), are dominated by algae with a few putative cnidarians (Yuan et al. Reference Yuan, Chen, Xiao, Wan, Guan, Wang, Zhou and Hua2013), so were mainly autotrophic with some possible suspension feeders (Wan et al. Reference Wan, Yuan, Chen, Guan, Pang, Tang and Xiao2016). The majority of communities before 560 Ma, from Newfoundland, Canada, and Charnwood Forest, U.K. (Clapham et al. Reference Clapham, Narbonne and Gehling2003; Wilby et al. Reference Wilby, Carney and Howe2011), only have suspension-feeding organisms, rendering them ecologically monotypic. Ecological diversification of relative abundance of ecological modes starts to occur around 550 Ma, with communities from Nilpena, South Australia (Droser et al. Reference Droser, Gehling, Tarhan, Evans, Hall, Hughes and Hughes2019), and the White Sea, Russia (Zakrevskaya Reference Zakrevskaya2014), hosting communities with only suspension feeders (cf. Funisia beds; Droser and Gehling Reference Droser and Gehling2008), as well as more ecologically diverse communities with algae, suspension feeders, scavengers (Gehling and Droser Reference Gehling and Droser2018), and microbial mat feeders (both under and on top of the mat) (Ivantsov and Malakhovskaya Reference Ivantsov and Malakhovskaya2002; Droser et al. Reference Droser, Gehling, Tarhan, Evans, Hall, Hughes and Hughes2019; Evans et al. Reference Evans, Gehling and Droser2019; Fig. 3). Less is known about the period between 550 Ma and 539 Ma, as fewer communities are preserved in situ, but the Shibantan (551–543 Ma) records a diversity of complex mobile animals such as Yilingia (Chen et al. Reference Chen, Zhou, Yuan and Xiao2019) and ichnofossils as well as a diversity of suspension feeders and macroalgae (Xiao et al. Reference Xiao, Chen, Pang, Zhou and Yuan2021). The relative abundances of Shibantan communities are not (yet) known, but from the diversity of organisms, appear ecologically similar to White Sea assemblage communities. Note, that because the feeding mode of some complex, endemic species such as Yilingia are unresolved, there could be as yet unreported additional feeding complexity. As in older (~555 Ma) communities, the terminal Ediacaran (550–539 Ma) has a plethora of suspension-feeding tubular organisms preserved alongside trace fossils, but biomineralized forms dominate (Becker-Kerber et al. Reference Becker-Kerber, Pacheco, Rudnitzki, Galante, Rodrigues and Leme2017, Reference Becker-Kerber, Paim, Chemale Junior, Girelli, da Rosa, Albani and Osés2020; Warren et al. Reference Warren, Inglez, Xiao, Buatois, Mángano, Okubo and Alessandretti2023), and the relative proportions of predators are not known (Hua et al. Reference Hua, Pratt and Zhang2003). These suspension feeding–dominated communities form two distinct community types. In Namibia, Brazil, and Paraguay, Cloudina and Namaclathus reefs dominate (Hofmann and Mountjoy Reference Hofmann and Mountjoy2001; Grotzinger et al. Reference Grotzinger, Adams and Schroder2005; Warren et al. Reference Warren, Fairchild, Gaucher, Boggiani, Poiré, Anelli and Inchausti2011; Penny et al. Reference Penny, Wood, Curtis, Bowyer, Tostevin and Hoffman2014; Becker-Kerber et al. Reference Becker-Kerber, Pacheco, Rudnitzki, Galante, Rodrigues and Leme2017). Soft-bodied rangeomorphs and erniettomorphs are also present, occupying different settings than the reefs (Pflug Reference Pflug1966; Narbonne et al. Reference Narbonne, Saylor and Grotzinger1997; Grazhdankin and Seilacher Reference Grazhdankin and Seilacher2002) and are found mainly in transported deposits (Bowyer et al. Reference Bowyer, Wood and Poulton2017).
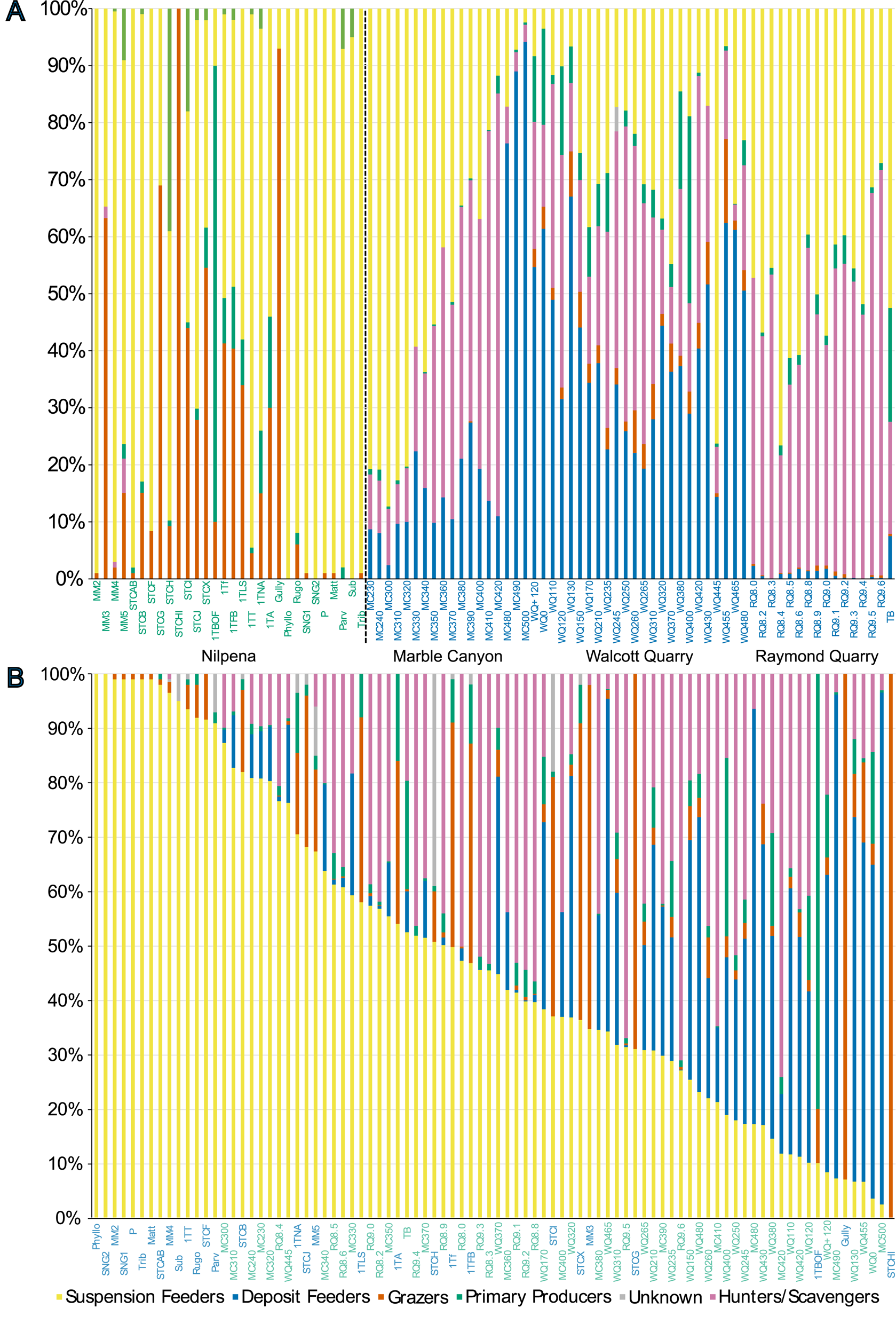
Figure 3. The relative proportions of feeding type in Ediacaran (green) from Nilpena, South Australia (Droser and Gehling Reference Droser and Gehling2015; Droser et al. Reference Droser, Gehling, Tarhan, Evans, Hall, Hughes and Hughes2019) and Cambrian (blue) from the Burgess Shale, Canada (Nanglu et al. Reference Nanglu, Caron and Gaines2020b). Feeding types are suspension feeding (yellow), deposit feeding (blue), grazing (orange), primary production (green), hunters and scavengers (red), and unknown in gray. The top plot is ordered temporally, and the bottom plot is ordered by relative proportion of suspension feeders from left to right. Note that while there is a trend of Ediacaran on the left to Cambrian on the right, there is a significant overlap between them.
Less is known about the Terreneuvian, due to the lack of deposits preserving exceptional soft tissues (see “Digging Deeper, Building Bigger”), so ecological dynamics cannot be resolved until the Cambrian Epoch 2. Deposits of this age and younger, for which the Chengjiang and Burgess Shale have been studied in most detail, show a variety of different dominant ecological modes. Chengjiang communities (518 Ma), are dominated by carnivorous taxa (mainly polychaetes) (Zhao et al. Reference Zhao, Zhu and Hu2010, Reference Zhao, Caron, Bottjer, Hu, Yin and Zhu2014), similar to near-contemporaneous Sirius Passet communities (Harper et al. Reference Harper, Hammarlund, Topper, Nielsen, Rasmussen, Park and Smith2019). In contrast, Burgess Shale localities (506 Ma; Fig. 3) have a mixture of deposit feeding– (Walcott Quarry) or suspension feeding–dominated communities (Tulip Beds) (O'Brien and Caron Reference O'Brien and Caron2016), although it is important to note that recent flume experiments and reanalysis of sedimentary structures suggest that organisms from the classic Walcott locality in the Burgess Shale may have been transported large distances (Bath Enright et al. Reference Bath Enright, Minter, Sumner, Mángano and Buatois2021). When the localities are sampled at a finer scale (10 cm sample intervals), a more complex pattern emerges, with four community types: suspension feeding, hunting/scavenging, deposit feeding, and nektobenthic–vagrant–deposit feeding (Nanglu et al. Reference Nanglu, Caron and Gaines2020b), with a more general Cambrian Stage 3 pattern of the four Burgess Shale-type community types (Hou et al. Reference Hou, Siveter, Siveter, Aldridge, Cong, Gabbot, Ma, Purnell and Williams2017; Fu et al. Reference Fu, Tong, Dai, Liu, Yang, Zhang, Cui, Li, Yun, Wu, Sun, Liu, Pei, Gaines and Zhang2019) alongside those dominated by archaeocyath reefs (Sun et al. Reference Sun, Zhao, Steiner, Li, Na, Pan, Yin, Zeng, Van Iten and Zhu2020).
While the diversification of feeding modes throughout the ECT is high, the innovation of predation-dominated systems does not lead to a unidirectional change in ecosystem functioning, but more a broadening of different sorts of relative abundances of ecological niches across a spectrum. This point is illustrated by the notable overlap that occurs in the ranking of Nilpena Ediacaran communities (Droser and Gehling Reference Droser and Gehling2015; Droser et al. Reference Droser, Gehling, Tarhan, Evans, Hall, Hughes and Hughes2019) with Burgess Shale Cambrian communities (Nanglu et al. Reference Nanglu, Caron and Gaines2020b) when ordered by proportion of suspension feeding (Fig. 3).
Abiotic and Non-trophic Biotic Interactions within Communities
The relative abundances of different ecological groups within each community are a result of the interactions of taxa within the community, and how they interact with the local resources/environment. The environmental impacts on organisms, and the feedback relationships between organisms and their abiotic environment, are dependent on the relative needs of the organisms and the extent of abiotic nutrients. Organisms adapt to their environments to optimize their efficiency, for example, rangeomorphs maximized their surface area to volume ratios in order to optimize nutrient/resource exchange with the water column (Narbonne Reference Narbonne2004; Laflamme et al. Reference Laflamme, Xiao and Kowalewski2009). These abiotic impacts can, and most commonly do, include organisms only existing in suitable habitats—these can be very clear, such as only organisms with good cold tolerance existing at high latitudes, or more subtle, such as trees having higher densities in regions of higher soil nutrients. These habitat associations and influences can be detected through a variety of different methods. In the Ediacaran, spatial point process analyses have been used to detect increased densities where taxa (or pairs of taxa) exhibit higher densities in areas of good-quality patchy habitat (Baddeley and Turner Reference Baddeley and Turner2005; Illian et al. Reference Illian, Penttinen, Stoyan and Stoyan2008; Wiegand and Moloney Reference Wiegand and Moloney2013; Mitchell et al. Reference Mitchell, Bobkov, Bykova, Dhungana, Kolesnikov, Hogarth and Liu2020). Furthermore, these increased densities or aggregations can be statistically distinguished from other aggregation processes such as dispersal limitation and reproductive processes and facilitation. These methods have shown that environmental, or niche, influences are limited from 572 to 560 Ma (Mitchell and Kenchington Reference Mitchell and Kenchington2018; Mitchell et al. Reference Mitchell, Harris, Kenchington, Vixseboxse, Roberts, Clark, Dennis, Liu and Wilby2019), and increase from 560 to 550 Ma (Mitchell et al. Reference Mitchell, Bobkov, Bykova, Dhungana, Kolesnikov, Hogarth and Liu2020). Preferential settlement (Boan et al. Reference Boan, Evans, Hall and Droser2023) with community composition corresponding to different microbial mat maturity/complexity (Droser et al. Reference Droser, Evans, Tarhan, Surprenant, Hughes, Hughes and Gehling2022) demonstrates the importance of local environment to communities. In the terminal Ediacaran (550–539 Ma), the reef builders Cloudina, Corumbellina, and Namacalathus show clear associations with biolaminites such stromatolites and thrombolites (Hofmann and Mountjoy Reference Hofmann and Mountjoy2001; Grotzinger et al. Reference Grotzinger, Adams and Schroder2005; Warren et al. Reference Warren, Fairchild, Gaucher, Boggiani, Poiré, Anelli and Inchausti2011; Cai et al. Reference Cai, Hua, Schiffbauer, Sun and Yuan2014), reflecting either biotic interactions and/or mutual habitat associations.
The importance of local habitat patchiness increases into the Cambrian, as can be seen through the trace fossil record, through an increase in sediment bulldozing into the Fortunian as sediment feeders diversified (Mángano and Buatois Reference Mángano and Buatois2014; Buatois et al. Reference Buatois, Almond, Mángano, Jensen and Germs2018) and where the complexity of interactions of ichnotaxa with patchiness increases (Mitchell et al. Reference Mitchell, Evans, Chen and Xiao2022). As the depth and complexity of burrowing increased into Cambrian Stage 2, with the onset of mix-ground ecology and suspension feeding from within the sediment (Mángano and Buatois Reference Mángano and Buatois2014), these subtle differences of substrate local patchiness likely decreased in importance, because large-scale and more significant habitat variations took over, and the benthic realm became more akin to the modern, with other factors becoming a first-order process of habitat variation, rather than subtle differences in microbial mat or sediment variations.
Biotic interactions, that is, the interactions between two taxa, consist of interactions such as competition, facilitation and mutualisms, and trophic interactions. For the oldest Ediacaran communities, there is limited evidence of competition for resources, with only rare intra- and interspecific competition (Mitchell and Butterfield Reference Mitchell and Butterfield2018; Mitchell and Kenchington Reference Mitchell and Kenchington2018). As the extent of intra- and interspecific competition increases throughout the Ediacaran (Droser et al. Reference Droser, Tarhan and Gehling2017), coupled to an increase in relative importance of niche/environment interactions (Mitchell et al. Reference Mitchell, Bobkov, Bykova, Dhungana, Kolesnikov, Hogarth and Liu2020; Droser et al. Reference Droser, Evans, Tarhan, Surprenant, Hughes, Hughes and Gehling2022), so does the complexity of interactions, with facilitation first detected in the terminal Ediacaran in terms of preferred settling near favorable fluid dynamics flow (Gibson et al. Reference Gibson, Darroch, Maloney and Laflamme2021).
Complexity of types of ecological interactions increases in the Cambrian, with symbiosis (Vinn Reference Vinn2017), parasitism (Peel Reference Peel2015; Li et al. Reference Li, Williams, Harvey, Wei, Zhao, Guo, Gabbott, Fletcher, Hou and Cong2020; De Baets et al. Reference De Baets, Huntley, Scarponi, Klompmaker and Skawina2021; Chen et al. Reference Chen, Topper, Skovsted, Strotz, Shen and Zhang2022), mutualisms and commensalism (Topper et al. Reference Topper, Holmer and Caron2014; Peel Reference Peel2015; Nanglu and Caron Reference Nanglu and Caron2021), and epibionts (Zhang et al. Reference Zhang, Han, Wang, Emig and Shu2009; Topper et al. Reference Topper, Strotz, Holmer and Caron2015) all present. Yet, in terms of observed competition within Cambrian ecosystems, there is only limited evidence of its impact. Competitive exclusion is extremely rare in Phanerozoic benthic marine ecosystems (Klompmaker and Finnegan Reference Klompmaker and Finnegan2018), likely due to the higher levels of bioturbation, predation, and disturbance preventing ecosystems from reaching carrying capacity (Stanley Reference Stanley2008; Klompmaker and Finnegan Reference Klompmaker and Finnegan2018). An exception is possible in the case of archaeocyath reefs. Distorted specimens likely resulted from competition for space (Zhuravlev and Wood Reference Zhuravlev and Wood1995), in an ecosystem lacking known predators and—due to its biomineralized nature—less prone to environmental disturbance. Even then, both niche and neutral processes appear to have played a role in these early sponge reef ecosystems (Zhuravlev et al. Reference Zhuravlev, Naimark and Wood2015).
Trophic Interactions
Trophic interactions, namely the interactions that occur between an organism and its food source, have been suggested to be a key driver of the Cambrian Explosion, with predators and their prey driving the morphological changes and diversity seen during the Cambrian (Bengtson Reference Bengtson2002). Trophic complexity started relatively simply (Fig. 4), with early Ediacaran organisms feeding primarily/exclusively on plankton and POM/POC from the water column (Laflamme et al. Reference Laflamme, Xiao and Kowalewski2009; Butterfield Reference Butterfield2022). New trophic links formed between organisms and the microbial mat, with Dickinsonia and Yorgia feeding on it (Ivantsov and Malakhovskaya Reference Ivantsov and Malakhovskaya2002; Sperling and Vinther Reference Sperling and Vinther2010; Ivantsov Reference Ivantsov2011; Evans et al. Reference Evans, Gehling and Droser2019; Ivantsov et al. Reference Ivantsov, Nagovitsyn and Zakrevskaya2019; Ivantsov and Zakrevskaya Reference Ivantsov and Zakrevskaya2021; Bobrovskiy et al. Reference Bobrovskiy, Nagovitsyn, Hope, Luzhnaya and Brocks2022), and with the origination of the first scavengers (Gehling and Droser Reference Gehling and Droser2018; Fig. 4). There is no evidence of pelagic macroscopic predation until the terminal Ediacaran (Hua et al. Reference Hua, Pratt and Zhang2003). Further insights into the extent of resource niche overlap found that the majority of Ediacaran communities from 572 to 560 Ma had shared niches, so likely shared food sources; niche complexity increased with complex/partitioned niches within communities during 560–550 Ma, then decreased with simpler/shared niches in 550–540 Ma (Darroch et al. Reference Darroch, Laflamme and Wagner2018a). These data likely reflect an increase in trophic complexity around 555 Ma, potentially followed by a decrease in the terminal Ediacaran.
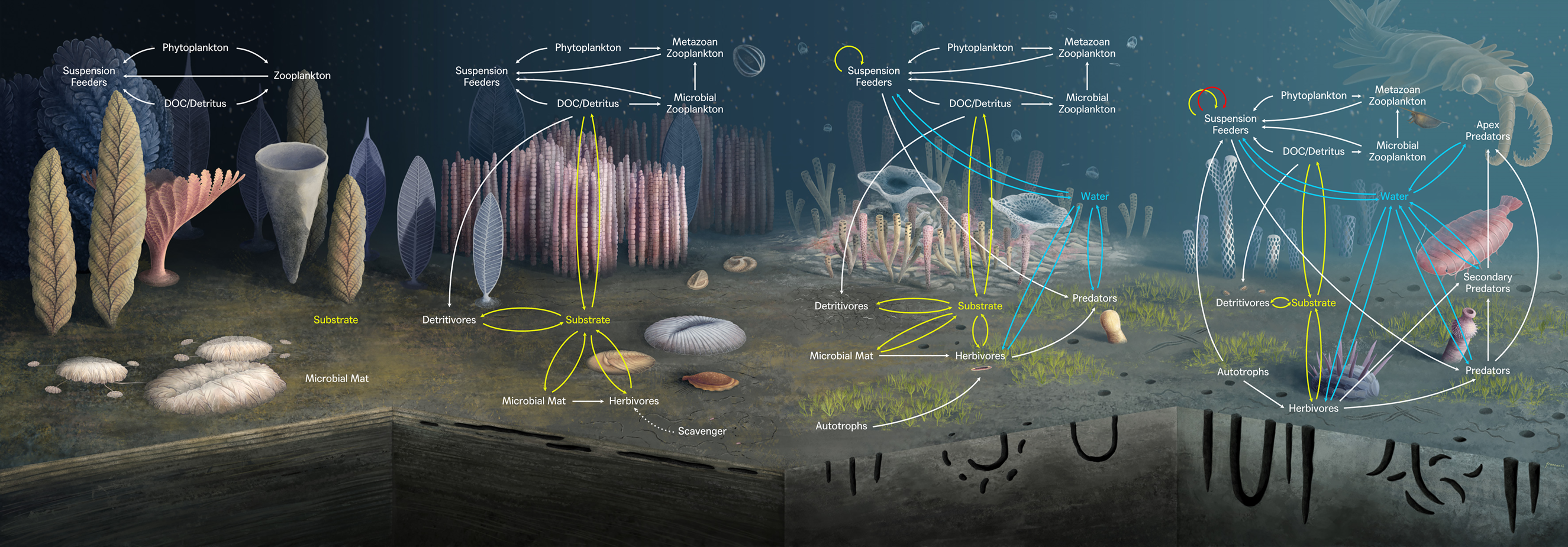
Figure 4. Ecological networks across the Ediacaran/Cambrian transition (ECT), with four time periods illustrated (from left to right: 565 Ma, 550 Ma, 539–521 Ma, 521 Ma). Suspension-feeding communities and simple trophic interactions (white arrows) are present 565 Ma. An increase in habitat modification (yellow arrows) in the form of grazing and scavenging appear 550 Ma. In the Terreneuvian (539–521 Ma), the active pumping of archaeocyaths and swimming of chaetognaths increase aquatic bioturbation (blue arrows), archaeocyath reefs modify the substrate, and vertical as well as horizontal bioturbation complexity and depth increase dramatically. In the Cambrian Epoch 2 (521 Ma), there is a further increase in trophic interactions, aquatic bioturbation, and vertical bioturbation, as well as symbiotic interactions and epibionts (red arrows). DOC, dissolved organic carbon. Reconstruction by Franz Anthony.
These matground–dominated dynamics persisted into the early Cambrian (Fortunian), with a shift in trophic dynamics starting in Cambrian Age 2, with a similar style of trophic dynamics to the modern present in Age 3 (Cambrian Epoch 2) (X. Zhang et al. Reference Zhang, Chang, Cui and Qiao2021; Fig. 4). There is a distinct shift in the importance of biotic interactions within Cambrian food webs from Age 3 and younger, that is, with trophic interactions, where we see a sharp increase in the functional morphology of feeding apparatuses, predatory apparatuses, and defensive tools (see “Radiation of Feeding Modes and Mobility”). Initially it was thought these trophic chains were relatively short, with only primary predators (Vannier and Chen Reference Vannier and Chen2005), but more recent work has suggested that there can be relatively long trophic chains four to five species long (Hu et al. Reference Hu, Steiner, Zhu, Erdtmann, Luo, Chen and Weber2007; Dunne et al. Reference Dunne, Williams, Martinez, Wood and Erwin2008). Such complex chains are not necessarily present in all Cambrian ecosystems, such as those dominated by suspension feeders or detritovores (cf. O'Brien and Caron Reference O'Brien and Caron2016; Fig. 3).
All these trophic interactions translate into relatively complex trophic networks (or food webs), with food webs of the Burgess Shale and Chengjiang displaying topologies similar to those of modern ones in terms of distributions of feeding links (Dunne et al. Reference Dunne, Williams, Martinez, Wood and Erwin2008). These Cambrian food webs show a dependence of their structure on the number of taxa and feeding links, which is found in modern webs modeled by simple “niche models.” However, the Chengjiang web has long paths (feeding chains) and more feeding loops than modern webs. One cause of this is that the prey species with the most predator links have many more predators than expected from modern webs, perhaps due to a lack of specialized defense mechanisms. Both Burgess Shale and Chengjiang food webs show a higher variation in the number of trophic links per taxa than is seen in the modern. These results are thought to suggest that as ecosystems evolved after the Cambrian, the trophic structure became more constrained (Dunne et al. Reference Dunne, Williams, Martinez, Wood and Erwin2008).
Ecosystem Resilience and Complexity
For ecosystems and the organisms within them to persist over geological timescales, these ecosystems must be stable, that is, have resilience. Ecosystem stability is strongly correlated to taxonomic and functional diversity, because more diverse systems have a better capacity to cope with biotic and abiotic changes and fluctuations through feedbacks within the network of interactions (abiotic, biotic, and trophic) within the community (May Reference May1974; Tilman Reference Tilman1994; McCann Reference McCann2000). These feedbacks and redundancy mean that the more complex an ecosystem is, the more stable it is (Ruiter et al. Reference Ruiter, Neutel and Moore1995; Neutel et al. Reference Neutel, Heesterbeek, van de Koppel, Hoenderboom, Vos, Kaldeway, Berendse and de Ruiter2007), in contrast to theoretical (random) networks, where complex networks are less stable (May Reference May1974, Reference May2019).
Ecological complexity is most simply measured in terms of taxonomic diversity, with more diversity corresponding to more stable ecosystems (Ives and Carpenter Reference Ives and Carpenter2007). However, ecological stability is not as straightforward as just taxonomic diversity stabilizing the ecosystems. If there is a single species that is crucial for ecosystem function/stability, then the system is vulnerable (Paine Reference Paine1969; Jain and Krishna Reference Jain and Krishna2002). As such, communities in which many species occupy similar niches tend to be more stable, so that if a specialist predator or pathogen or habitat changes, other species can fill that ecological role (Petchey and Gaston Reference Petchey and Gaston2006). The capacity for ecosystems to have this redundancy is most simply measured using evenness (Shannon Reference Shannon1948), that is, the distribution of relative abundances of taxa within an community. If the community is very skewed, and so has a few very high-abundance taxa and many rarer taxa, the system is likely much more sensitive to perturbations compared with communities that are more even (Hillebrand et al. Reference Hillebrand, Bennett and Cadotte2008).
Over the ECT, some Ediacaran communities exhibit relative high evenness, corresponding to many relatively abundant taxa and complex niches, alongside simpler, more monospecific communities (Darroch et al. Reference Darroch, Laflamme and Wagner2018a). As such, it is likely that Ediacaran ecosystems were not all equally resilient. The stabilizing mechanisms for this resilience can vary, with systems stabilized primarily through primary production availability (bottom-up), and other systems stabilized by predators (top-down). Due to the lack of higher predators and short trophic links (Fig. 4), Ediacaran ecosystems were stabilized from the bottom-up, so dependent on abiotic resources and environmental factors. The lack of resource competition suggests that such resources are not limited (Mitchell and Kenchington Reference Mitchell and Kenchington2018; Mitchell et al. Reference Mitchell, Harris, Kenchington, Vixseboxse, Roberts, Clark, Dennis, Liu and Wilby2019), likely making these Ediacaran systems relatively stable. This bottom-up stability likely persists until Cambrian Ages 2 and 3, when some (but not all; see “Dominant Ecological Modes within Communities”) systems exhibit many trophic levels, which could potentially lead to top-down stabilizing mechanism. However, longer feeding chains seen in the Chengjiang are sufficiently long that species may be functionally separated from one another, potentially destabilizing the systems (Dunne et al. Reference Dunne, Williams, Martinez, Wood and Erwin2008). The potential (relative) lack of stability in the Cambrian was also possibly present within Burgess Shale communities, where the Tulip Bed and Walcott Quarry communities have high abundances of a few dominant taxa, with many rarer taxa (O'Brien and Caron Reference O'Brien and Caron2016).
Macroecology
Determining the processes that shape community-level ecological metrics such as diversity and community composition is enhanced through comparisons with other communities, enabling the teasing apart of the relative importance of different temporal and environmental drivers, and how they interact with the organisms at a community level.
Beta Diversity
Beta diversity is a measure of how much variation there is between communities (Anderson et al. Reference Anderson, Crist, Chase, Vellend, Inouye, Freestone and Sanders2011), with high diversity indicating little species overlap and thus different species in different communities, and low beta diversity indicating high levels of overlap and thus similar species are found across different communities. Regions that exhibit high beta diversity are more stochastic, that is, the community compositions are not the systematic result of adaptions to their local environments and biotic interactions, but instead reflect colonization and dispersal dynamics, for example, as seen at modern hydrothermal vents (Giguère and Tunnicliffe Reference Giguère and Tunnicliffe2021). If biotic interactions and environmental filtering are strong drivers of community composition, we would expect the same community compositions to emerge within a given environment, and so beta diversity would be low, for example, in shallow-water reefs (Harborne et al. Reference Harborne, Mumby, ZŻychaluk, Hedley and Blackwell2006). As such, quantifying beta diversity can help inform the key factors underlying community composition and development.
Ediacaran beta diversity is significantly higher than that of the Cambrian and the rest of the Phanerozoic. This beta-diversity increase has been suggested to be due to the rarity of mobile taxa, which may have increased the patchiness of communities (Finnegan et al. Reference Finnegan, Gehling and Droser2019). Strong dispersal limitation, such as is produced via asexual stoloniferous reproduction (Mitchell et al. Reference Mitchell, Kenchington, Liu, Matthews and Butterfield2015), provides a complementary cause for community patchiness. Competitive communities can also increase beta diversity (Stanley Reference Stanley2008), but the rarity and weakness of resource competition within Avalonian Ediacaran communities suggest that that niche processes and biotic interactions shape the communities less than in extant systems (Na and Kiessling Reference Na and Kiessling2015; Finnegan et al. Reference Finnegan, Gehling and Droser2019). As such, high Ediacaran beta diversity may result from processes similar to those in hydrothermal vent systems. These vent systems often exhibit high beta diversity (Giguère and Tunnicliffe Reference Giguère and Tunnicliffe2021) due to the stochastic nature of the creation-suitable habitat (the vents). Thus, the identity of organisms that reach and colonize the new habitat first are more stochastic than most extent benthic systems. Over the ECT, patterns of beta diversity do not necessarily follow those of alpha diversity. Alpha diversity increases from the Ediacaran through to Cambrian Age 3 (Fig. 2), yet beta diversity decreases into the Fortunian before increasing again through Cambrian Ages 2 and 3. Middle-level beta diversity later in the Cambrian suggests an increase in the influence of these niche and biotic interactions throughout (Zhao et al. Reference Zhao, Caron, Bottjer, Hu, Yin and Zhu2014). In these first three stages of the Cambrian, alpha/beta-diversity dynamics are consistent with a low-competition dynamics, that is, little pressure on resources (Hautmann Reference Hautmann2014). New species appear either in previously unexploited niches, or communities have more species within a single niche, potentially due to predation exerting a top-down control (Na and Kiessling Reference Na and Kiessling2015). Beta diversity increases from the Cambrian to the Devonian as resources are reduced, increasing competition and constricting niches (Na and Kiessling Reference Na and Kiessling2015; Penny and Kröger Reference Penny and Kröger2019). These beta-diversity patterns suggest that stochasticity is key to Ediacaran communities with limited niche and biotic interactions. Niches constrict and biotic interactions increase throughout the Cambrian, feasibly as a result of predation and top-down control.
Biogeography
Biogeographic patterns require sufficient sample sizes to resolve, which means that inferences over the ECT are limited. Within the Ediacaran, detailed biogeographic analyses are limited to differences between high and low paleolatitudes, due to the number and geographic placement of localities (Boddy et al. Reference Boddy, Mitchell, Meredith and Liu2021). The frondomorph group, which includes rangeomorphs and arboreomorphs, radiated from high to low paleolatitudes, in contrast to algal and protist groups, which showed no paleolatitudual differences (Boddy et al. Reference Boddy, Mitchell, Meredith and Liu2021). Further paleolatitudual differences are found with the bilaterian group, which radiated from low to high latitudes, unlike algal, tubular, soft-bodied, and biomineralizing taxa (Boddy et al. Reference Boddy, Mitchell, Meredith and Liu2021), showing Ediacaran biogeographic differences with taxon distributions through time, even at this coarse spatial scale. The drivers behind these two patterns are not yet resolved, but the frondomorph high to low radiation is likely due to evolutionary processes with a deep-water, high-latitude, origination (Griffiths et al. Reference Griffiths, Whittle and Mitchell2023), while the bilaterian paleolatitude signal could be a reflection of high rates of diversification at the tropics leading to quicker evolution at these lower latitudes (Mittelbach et al. Reference Mittelbach, Schemske, Cornell, Allen, Brown, Bush and Harrison2007).
In the Cambrian, the presence and strength of biogeographic biodiversity patterns increased, showing increase in provinciality, or bioregions, coupled to a decrease in geographic ranges (Hendricks et al. Reference Hendricks, Lieberman and Stigall2008; Na et al. Reference Na, Kocsis, Li and Kiessling2023). This increase in biogeographic patterns has been suggested to relate to several factors, including the geographic differences in origination of groups (Lieberman Reference Lieberman2002, Reference Lieberman2008; Lieberman and Meert Reference Lieberman and Meert2004); changes from low-competition to high-competition models with a change from wide to narrower geographic ranges (Na and Kiessling Reference Na and Kiessling2015); differences within groups, relating to dispersal abilities (e.g., with soft-bodied, pelagic Cambrian arthropods showing greater geographic ranges than trilobites; Hendricks et al. Reference Hendricks, Lieberman and Stigall2008); and more broadly to biogeographic differentiation at regional and local scales (Na et al. Reference Na, Kocsis, Li and Kiessling2023). However, in some cases, for example, the trilobites, their origination within the Cambrian (Paterson et al. Reference Paterson, Edgecombe and Lee2019) does not correlate with the breakup of Pannotia tens of millions of years earlier, leaving many patterns still to be explained.
Metacommunity Ecology
The many different types of ECT communities (see “Dominant Ecological Modes within Communities”) could be due to evolutionary/temporal environmental filtering, and/or the result of different biotic interactions. Cluster analyses based on community composition can shed led on these drivers, because if environment dominates, we expect the groups of communities that share similar composition to have lived in similar environments. If the communities represent a progressive evolution, then the community groups of similar geological ages should cluster together (Anderson Reference Anderson1971). Once different groups of communities, or metacommunities, have been established, the metacommunity structure within these groups can shed further light on the driving forces within them, elucidating differences in environmental and biotic drivers and the relative importance of stochasticity between metacommunities (Leibold et al. Reference Leibold, Holyoak, Mouquet, Amarasekare, Chase, Hoopes and Holt2004).
The three classic assemblages of the Ediacaran—Avalon, White Sea, and Nama—as first proposed by Waggoner in 2003 occupied distinct temporal, environmental as well as species-specific assemblages (Waggoner Reference Waggoner2003), with a direct reading suggesting an evolutionary radiation from deep-water, high-latitude sites. Since then, the number of sites has increased dramatically from 15 in Waggoner's initial analyses to 127, with more overlap between environments and time, yet these three key assemblages (or biozones) remain robust when examined using a variety of different clustering and network approaches (Boag et al. Reference Boag, Darroch and Laflamme2016; Muscente et al. Reference Muscente, Bykova, Boag, Buatois, Mángano, Eleish and Prabhu2019; Boddy et al. Reference Boddy, Mitchell, Meredith and Liu2021), so are likely fundamental to the Ediacaran. Because the environmental and temporal range of the Ediacaran localities are largely (but not totally) non-overlapping, it is not possible to determine the extent to which the differences between the oldest, Avalon assemblage and the much more diverse White Sea assemblage are evolutionary or environmental (Grazhdankin Reference Grazhdankin2004; Boag et al. Reference Boag, Darroch and Laflamme2016). As work on other sites, such as the Lantian, Shibantan, and Corumbá localities increases, it possible that additional assemblages may emerge, such as with the Shibantan (Xiao et al. Reference Xiao, Chen, Pang, Zhou and Yuan2021), with the potential of overlapping temporal and species ranges of the new data enabling a teasing apart of environmental versus evolutionary trends.
Ediacaran diversity increases between the Avalon and White Sea, then decreases between the White Sea and Nama, which at a global scale is not due to sampling differences (Muscente et al. Reference Muscente, Bykova, Boag, Buatois, Mángano, Eleish and Prabhu2019; Boddy et al. Reference Boddy, Mitchell, Meredith and Liu2021). This diversity decrease has been suggested to be caused by a catastrophic mass extinction (Darroch et al. Reference Darroch, Smith, Laflamme and Erwin2018b, Reference Darroch, Smith, Nelson, Craffey, Schiffbauer and Laflamme2023b; Zhuravlev and Wood Reference Zhuravlev and Wood2018; Muscente et al. Reference Muscente, Bykova, Boag, Buatois, Mángano, Eleish and Prabhu2019; Evans et al. Reference Evans, Tu, Rizzo, Surprenant, Boan, McCandless, Marshall, Xiao and Droser2022); change in taphonomic window (Laflamme et al. Reference Laflamme, Darroch, Tweedt, Peterson and Erwin2013); or a biotic replacement model, whereby Phanerozoic-type animals outcompeted Ediacaran-type animals, driving them extinct (Darroch et al. Reference Darroch, Sperling, Boag, Racicot, Mason, Morgan and Tweedt2015), potentially through “wormworld” fauna (Schiffbauer et al. Reference Schiffbauer, Huntley, O'Neil, Darroch, Laflamme and Cai2016). This diversity decrease is robust to sampling differences on a global scale (Muscente et al. Reference Muscente, Bykova, Boag, Buatois, Mángano, Eleish and Prabhu2019); however, there are paleolatitudinal differences in sampling biases, with low-latitude diversity not significantly different from the number of low-latitude localities, and high-latitude decoupled with only weak significance (Boddy et al. Reference Boddy, Mitchell, Meredith and Liu2021). This latitudinal difference suggests that some (but not all) of the drop in diversity in the terminal Ediacaran may be an artifact of sampling biases. Further differences in diversity could be due to internal restructuring: analyses of the metacommunity structure between the three large assemblages suggest diversity differences between the White Sea and Nama assemblages are more likely due to internal ecological restructuring, because there is an increase in turnover, a maintenance/increase in significant pairwise taxon associations, and an increase in depth specialization, which are all inconsistent with a catastrophic mass extinction (Eden et al. Reference Eden, Manica and Mitchell2022). As such, biological (within-organism) and ecological complexity likely increased throughout the Ediacaran.
In the Cambrian, the paleocommunity data from contemporaneous Cambrian sites within different paleoenvironments enable the teasing apart of niche partitioning (Na et al. Reference Na, Kocsis, Li and Kiessling2023). However, Lagerstätten with soft-bodied preservation that are sufficiently sampled to be able to make ecological inferences are relatively constrained to an ~20 Myr between 518 and 499 Ma, which limits the ability to infer evolutionary or temporal trends from the data. In addition, the vast majority of these Lagerstätten are found in the paleotropics. Nonetheless, temporal trends are observable in some cases, such as in echinoderms, where the differences in their presence between the Chengjiang and Burgess Shale localities could be evolutionary (O'Brien and Caron Reference O'Brien and Caron2016). Temporal trends can also be detected at a fine-scale resolution through collection of data from narrow sample intervals of Burgess Shale communities (10 cm, ~600 yr Nanglu et al. Reference Nanglu, Caron and Gaines2020b). These fine-scale analyses reveal periods of relative ecological stability, followed by a turnover in community composition, suggesting periodic environmental disturbances and then recolonization (Caron and Jackson Reference Caron and Jackson2008), with similar community composition between successive sample intervals. Temporal trends also changed Burgess Shale community compositions, with the youngest communities (Tulip Bed) lacking hemichordates and annelids, which are abundant in the older localities. One such suggestion for these changes is competitive exclusion within a niche, for example, of sponges by hyoliths and hemichordates (Nanglu et al. Reference Nanglu, Caron, Conway Morris and Cameron2016, Reference Nanglu, Caron and Gaines2020b; Moysiuk et al. Reference Moysiuk, Smith and Caron2017).
The relative effects of environmental filtering (i.e., different taxa living within different environments) varies in the Cambrian. In the House Range (Utah), the higher diversity of pelagic animals in the Marjum Formation compared with the slightly older Wheeler Formation was suggested to be due to the deepening of the environment in the Marjum Pass area of the Great Basin and/or changing regional ocean circulation (Pates et al. Reference Pates, Lerosey-Aubril, Daley, Kier, Bonino and Ortega-Hernández2021b). Older Chengjiang communities (518 Ma) also show evidence of environmental filtering with a gradient from lower diversity nearshore to higher diversity offshore (Zhao et al. Reference Zhao, Hu, Caron, Zhu, Yin and Lu2012), and a high facies control on community compositions (Sun et al. Reference Sun, Zhao, Steiner, Li, Na, Pan, Yin, Zeng, Van Iten and Zhu2020), but such signals are not present in Burgess Shale data (O'Brien and Caron Reference O'Brien and Caron2016).
Other differences between the Chengjiang and Burgess Shale localities include differences in specialization. In contrast to Chengjiang taxa, dominant Burgess Shale taxa show broad stratigraphic and geographic ranges, with the four different community types not split by locality or environment, but instead varying at local and regional scales (Nanglu et al. Reference Nanglu, Caron and Gaines2020b). The reoccurrence of the abundant taxa within Walcott Quarry communities suggests that these abundant taxa are likely environmental and/or geographic generalists, with the majority of abundant Walcott Quarry taxa also present in Tulip Beds, albeit as rare taxa (e.g., Marella) (Caron and Jackson Reference Caron and Jackson2008). There are signals of localization, because many of the taxa from the Walcott Quarry and Tulip Beds occur only within a single horizon, suggesting that some taxa are adapted to their local environments, but not as well adapted to the wider regional environment (O'Brien and Caron Reference O'Brien and Caron2016). Note that some of these differences could be taphonomic or due to transportation, as the mobile detritivores that dominate Walcott Quarry communities may have escaped from the small burial events at the Tulip Beds (O'Brien and Caron Reference O'Brien and Caron2016), and fossils from the classic Burgess Shale quarry may have undergone significant transport (Bath Enright et al. Reference Bath Enright, Minter, Sumner, Mángano and Buatois2021). While these patterns suggest recolonization from a species pool of taxa with good dispersal and/or colonization ability was important, that is, community composition was still relatively stochastic (as suggested by the beta-diversity patterns; Na and Kiessling Reference Na and Kiessling2015), the dispersal/recolonization cannot account for all the community differences found, with priapulids and sponges abundant throughout most Cambrian localities, but not at Marble Canyon, suggesting some temporal or environmental filtering (Nanglu et al. Reference Nanglu, Caron and Gaines2020b).
There are differences in ecological dominance between Chengjiang and Burgess Shale communities, with Chengjiang dominated by carnivorous taxa (Zhao et al. Reference Zhao, Caron, Bottjer, Hu, Yin and Zhu2014) in contrast to deposit- and suspension-feeding ecological dominance in Burgess Shale localities (O'Brien and Caron Reference O'Brien and Caron2016). A comparable signal is seen in the large swimming radiodonts, with raptorial amplectobeluids and anomalocaridids dominant in Chengjiang, but sediment-sifting hurdiids more diverse in the Burgess Shale and Great Basin (Wu et al. Reference Wu, Pates, Ma, Lin, Wu, Zhang and Fu2022). Again, these could be the result of environmental differences, rather than temporal or geographic differences, but to resolve the underlying drivers of these differences requires a series of community-level samples from sites spanning a broad temporal range but limited geographically. Thus, data from Chengjiang and the Burgess Shale could be complemented with similar high-resolution studies from Kaili or the Great Basin respectively.
Stochastic to Deterministic Trends
Metacommunity comparisons reveal patterns of change across the ECT. In the Ediacaran, such metacommunity analyses have shown a pattern of increasing specialization not only of organisms, but of communities (Eden et al. Reference Eden, Manica and Mitchell2022). The oldest Ediacaran assemblage, the Avalon, is populated by generalists, with little environmental influence. In the White Sea assemblage, there is increased metacommunity variation, with metacommunity structure dependent on environment and biogeographic differences in the pairwise interactions of taxa. The terminal Ediacaran assemblage, the Nama, shows strong niche segregation (Eden et al. Reference Eden, Manica and Mitchell2022). Patterns of increasing specialization and habitat segregation are also seen in early Cambrian ichnotaxa, which start out as generalists in the Fortunian, but then increase in specialization, leading to habitat segregation in the Cambrian Stage 2 (Meek et al. Reference Meek, Buatois, Mángano and Eglington2023). However, the overarching change over the ECT from stochasticity populated by generalists to more deterministic, with more specialists is not necessarily a unidirectional process. Cambrian archaeocyath sponge reef communities on the Siberian Platform (525–514 Ma) show changes between individually driven metacommunity structure to within-community interactions and metacommunity complexity as reef habitat expands, and then a subsequent reduction in metacommunity complexity as reef habitat is reduced (Zhuravlev et al. Reference Zhuravlev, Mitchell, Bowyer, Wood and Penny2022). Coupled to diversity patterns in and between different Cambrian clades (Servais et al. Reference Servais, Cascales-Miñana, Harper, Lefebvre, Munnecke, Wang and Zhang2023), these patterns demonstrate that while ecological complexity increased, it was not a simple linear process, but instead was likely pulsed and multidirectional (Zhuravlev and Wood Reference Zhuravlev and Wood2018) and heterogeneous environmentally and geographically.
Ecosystem Engineering
Interactions and feedbacks within and between ecosystems can not only stabilize, but also drive ecological dynamics through ecosystem engineering, whereby taxa change their local environment in a way that makes it more habitable for other organisms. The precise definition of ecosystem engineering is much debated: on one side, every living organism changes its environment (Jones et al. Reference Jones, Lawton and Shachak1994), but on the other side, it is not useful to discuss ecosystem engineering in terms of ecosystem changes that do not significantly change and improve the environment for other organisms (Reichman and Seabloom Reference Reichman and Seabloom2002). As such, we will define ecosystem engineering as occurring when organisms have impacted their environments sufficiently to produce observable (positive) impacts on other organisms (cf. Hastings et al. Reference Hastings, Byers, Crooks, Cuddington, Jones, Lambrinos, Talley and Wilson2007).
There are four main ecosystem engineering mechanisms that have been suggested to contribute to the ECT diversification: (1) Savanna hypothesis, (2) reefs, (3) vertical substrate bioturbation, and (4) aquatic bioturbation. The Savanna hypothesis is the oldest form of metazoan ecosystem engineering, whereby early bilaterian evolution is driven by the search for patchy food sources on the seafloor that come from the decaying remains of sessile organisms (Plotnick et al. Reference Plotnick, Dornbos and Chen2010; Budd and Jensen Reference Budd and Jensen2017). This mechanism persists until Cambrian Stage 3, when multiple different processes, namely pervasive burrowing, detritivores, and scavenging, ensure that such patchy resources do not remain in the sediment long enough to be evolutionary drivers. This hypothesis is consistent with analyses from Ediacaran community ecology. These analyses show an increase in the amount of interactions that Ediacaran organisms had with their environments (Mitchell et al. Reference Mitchell, Bobkov, Bykova, Dhungana, Kolesnikov, Hogarth and Liu2020), increased scavenging (Evans et al. Reference Evans, Gehling and Droser2019), and increased ichnodisparity (Mángano and Buatois Reference Mángano and Buatois2014), and the extent and complexity to which trace fossil interact substrate patchiness (Mitchell et al. Reference Mitchell, Evans, Chen and Xiao2022).
The second group of ECT ecosystem engineers are reefs, which dramatically changed their environment through the creation of hard substrate and complex 3D space. Through this reef creation they created more niches, which in modern systems are highly correlated with diversity, as reef animals use coral structures for food, protection from predation, habitat, and nurseries (Bozec et al. Reference Bozec, Yakob, Bejarano and Mumby2013; Brandl et al. Reference Brandl, Rasher, Côté, Casey, Darling, Lefcheck and Duffy2019). However, when these opportunities within reefs started to be fully utilized is not clear-cut. The Ediacaran reefs of Cloudina, Corumbella, and Namacalathus demonstrate significant ability to build large structures, but there is no direct evidence of facilitative ecological interactions of other animals with these Ediacaran reefs (Warren et al. Reference Warren, Quaglio, Simões, Gaucher, Riccomini, Poiré, Freitas, Boggiani and Sial2017; Wood et al. Reference Wood, Curtis, Penny, Zhuravlev, Curtis-Walcott, Iipinge and Bowyer2017), although the extent to which this could be a preservational artifact is not clear, because soft-bodied Ediacaran organisms are not easily preserved within such carbonate settings (Hall et al. Reference Hall, Kaufman, Vickers-Rich, Ivantsov, Trusler, Linnemann and Hofmann2013). Archaeocyath reefs are abundant from the early Cambrian, yet again the evidence of direct facilitation of diversity and habitat creation is missing. A second potential ecosystem engineering effect of archaeocyath reefs is to increase nutrient flux to the benthos through increased fluid flow over the reefs, which then creates capacity for an increase in diversity of scavengers and detrivores as well as increasing the capacity for higher trophic–level predators (Manzuk et al. Reference Manzuk, Maloof, Kaandorp and Webster2023). The reduction in diversification after the extinction of archaeocyaths suggests that these reefs may have contributed to diversification over the ECT (Na and Kiessling Reference Na and Kiessling2015; Figs. 1, 2). The first demonstration of colonization of biotic hard substrates is not until Cambrian Stage 4, when brachiopods form the Guanshan Biota are the first to host epibionts (Chen et al. Reference Chen, Topper, Skovsted, Strotz, Shen and Zhang2022). While the presence of reefs correlates with increasing diversity (Reaka-Kudla et al. Reference Reaka-Kudla, Wilson and Wilson1996; Small et al. Reference Small, Adey and Spoon1998), it is not until the terminal Cambrian that there is a significant correlation between taxonomic diversity origination in reefs (Kiessling et al. Reference Kiessling, Simpson and Foote2010). As such, while reefs substantially changed their environment, the evidence that organisms utilized their structures as ecosystem engineers is not in evidence until the terminal Cambrian, so the impact of such reef ecosystem engineering on the ECT diversity patterns is not clear.
In the third type of ecosystem engineering, the substrate was significantly changed through the ECT by increases in bioturbation, also known as the “agronomic revolution” or “Cambrian substrate revolution” (Seilacher Reference Seilacher, Krumbein, Paterson and Stal1994; Bottjer et al. Reference Bottjer, Hagadorn and Dornbos2000). The impact of the first trace makers on the physical environment was initially minimal, with horizontal trails such as Helminthoidichnites and Dickinsonia on or below microbial mats ~555 Ma (Ivantsov and Malakhovskaya Reference Ivantsov and Malakhovskaya2002; Buatois et al. Reference Buatois, Narbonne, Mángano, Carmona and Myrow2014; Evans et al. Reference Evans, Hughes, Gehling and Droser2020; Ivantsov and Zakrevskaya Reference Ivantsov and Zakrevskaya2021). These horizonal trails increased in density and complexity in the Shibantan and Nama assemblages (Cribb et al. Reference Cribb, Kenchington, Koester, Gibson, Boag, Racicot, Mocke, Laflamme and Darroch2019; Linnemann et al. Reference Linnemann, Ovtcharova, Schaltegger, Gärtner, Hautmann, Geyer and Vickers-Rich2019), with increased capacity to bulldoze (e.g., Parapsammichnites), or move sediments (Buatois et al. Reference Buatois, Almond, Mángano, Jensen and Germs2018), and increased depth penetration, cf. treptichnids (Mángano and Buatois Reference Mángano and Buatois2020). Matground coverage persisted into the Fortunian (Buatois et al. Reference Buatois, Narbonne, Mángano, Carmona and Myrow2014), indicating that sediment disturbance was insufficient to impact mat growth, but crucially, the development of vertical burrows, for example, Treptichnus pedum, and more complex structures, for example, Oldhamia, enabled more efficient feeding on the mats and sediments (Mángano and Buatois Reference Mángano and Buatois2020). Ichnodiversity and bioturbating organisms increased the most between the terminal Ediacaran and Fortunian within the ECT and Ordovician (Buatois et al. Reference Buatois, Mángano, Minter, Zhou, Wisshak, Wilson and Olea2020), but the most significant changes in benthic–pelagic coupling occurred during Cambrian Age 2 (529–521 Ma), with the colonization of the substrate by deep suspension-feeding burrowers, cf. Skolithos. Suspension feeders changed the benthic and pelagic environments by increasing organic carbon fluxes and geochemical recycling to the water column, expanding the surface area of the water–substrate interface through their burrows, thus ventilating the substrate, and bringing sediment up from deep to the water interface (Mángano and Buatois Reference Mángano and Buatois2020), and through the oxygenation of the sediment increasing niche space (Mángano and Buatois Reference Mángano and Buatois2014), although the realized impacts of these during the ECT may be minimal (Cribb et al. Reference Cribb, van de Velde, Berelson, Bottjer and Corsetti2023). Detritovores and deposit feeders further churned up the surface substrate, further enhancing geochemical fluxes (McIlroy and Logan Reference McIlroy and Logan1999; Mazurek Reference Mazurek2014; Herbers et al. Reference Herbers, MacNaughton, Timmer and Gingras2016; Stachacz Reference Stachacz2016; Gougeon et al. Reference Gougeon, Mángano, Buatois, Narbonne and Laing2018).
Finally, animals changed the water column by mixing the stratified oceans of the Ediacaran through movement of the water by filter feeding (Lenton et al. Reference Lenton, Boyle, Poulton, Shields-Zhou and Butterfield2014; Chen et al. Reference Chen, Topper, Skovsted, Strotz, Shen and Zhang2022) and swimming (Butterfield Reference Butterfield2009, Reference Butterfield2018). Biogenically induced aquatic bioturbation would have started with stromatolites and macroalgae changing fluid dynamics through their physical presence as they grew. As yet there is no evidence that these changes in fluid flow led to a feedback inducing further changes. Thus, the first evidence that animals started to contribute to disturbing the fluid flow, leading to changes in the occupation of vertical niches within the water column, comes from the Ediacaran ~572 Ma (Ghisalberti et al. Reference Ghisalberti, Gold, Laflamme, Clapham, Narbonne, Summons, Johnston and Jacobs2014). Mobility in the form of horizontal trace makers and mat stickers is unlikely to have impacted the fluid flow, as the physical changes were limited vertically. Planktonic pelagic macro-animals, such as Attenborites and jellyfish, also likely did not contribute to significant differences of fluid flow, because they only had limited ability to swim against the current. Therefore, the largest impact on ECT water-column fluid dynamics comes from filter feeding, as it increases in abundance both within and top on the substrate, increasing benthic–pelagic coupling (Mángano and Buatois Reference Mángano and Buatois2014). Today, the largest impact of animals comes from swimming, especially with the marine pump and diel vertical migration, whereby the vertical migration of zooplankton from the depths to the surface at night to feed is the largest (in terms of biomass) migration on this planet, with planktivores following the zooplankton, and predators following them (Barnes Reference Barnes1988; Cisewski et al. Reference Cisewski, Strass, Rhein and Krägefsky2010). Indeed, the feeding migrations of large marine animals such as whales contribute a substantial amount to oceanic mixing and the biological pump removing carbon from the atmosphere and bringing it into the deep sea (Berger Reference Berger2007; Cotte et al. Reference Cotte, d'Ovidio, Chaigneau, Lèvy, Taupier-Letage, Mate and Guinet2011; Lavery et al. Reference Lavery, Roudnew, Seuront, Mitchell and Middleton2012). However, with the advent of active swimming by macroscopic zooplankton in Cambrian Age 3 (Vannier Reference Vannier, Williams, Haywood, Gregory and Schmidt2007), nutrients and geochemical mixing would have massively increased vertically, as well as horizontally with larger organisms and larger dispersal ranges, thus increasing the habitability of the water column and opening up new pelagic niches (Butterfield Reference Butterfield2011, Reference Butterfield2018).
While the four types of different ecosystem engineering processes are not independent, taken together, these animals fundamentally changed the biosphere over the ECT (cf. Butterfield Reference Butterfield2011). Importantly, the strong positive feedbacks of these ecosystem engineering processes do not appear to have been fully realized immediately following the respective innovations. Indeed, it may not have been until the Ordovician that full ecosystem engineering feedbacks started, albeit with small effects (Erwin and Tweedt Reference Erwin and Tweedt2012).
Conclusions
Over the last 50 yr, we have seen a significant change in our understanding of the early evolution of animals with the taxonomic resolution of pre-Cambrian animals in the Ediacaran (Chen et al. Reference Chen, Zhou, Meyer, Xiang, Schiffbauer, Yuan and Xiao2013; Bobrovskiy et al. Reference Bobrovskiy, Hope, Ivantsov, Nettersheim, Hallmann and Brocks2018; Evans et al. Reference Evans, Hughes, Gehling and Droser2020; Dunn et al. Reference Dunn, Liu, Grazhdankin, Vixseboxse, Flannery-Sutherland, Green, Harris, Wilby and Donoghue2021, Reference Dunn, Kenchington, Parry, Clark, Kendall and Wilby2022) and the use of phylogenetic analyses to enable to recognition of Ediacaran and Cambrian taxa as stem-group representatives of modern classes and phyla (Daley et al. Reference Daley, Budd, Caron, Edgecombe and Collins2009; Legg et al. Reference Legg, Sutton and Edgecombe2013; Smith and Caron Reference Smith and Caron2015; Park et al. Reference Park, Nielsen, Parry, Sørensen, Lee, Kihm and Ahn2024; Rahman and Zamora Reference Rahman and Zamora2024). But key for the increase in our capacity to understand the drivers underlying the ECT is our changed approach of putting individual specimens and taxa in the context of the environments in which they lived. After all, it is the interactions that organisms experienced in their daily lives with one another and their environments, that is, their community ecology, that led to the morphological diversification and macroecology and evolutionary patterns that we see over the ECT.
Throughout the ECT, we not only see a diversification in the types of ecological interactions that exist, namely trophic interactions, habitat modifications, and biotic interactions, but also an increase in the number and type of these different interactions. Patterns across different ecological scales are remarkably consistent, with organismal, community, and macroecological patterns all showing a pattern of relative stochasticity, starting in the Ediacaran with low levels of biotic interactions such as competition (Mitchell and Kenchington Reference Mitchell and Kenchington2018; Mitchell et al. Reference Mitchell, Harris, Kenchington, Vixseboxse, Roberts, Clark, Dennis, Liu and Wilby2019; Dunn et al. Reference Dunn, Kenchington, Parry, Clark, Kendall and Wilby2022), which then changes with an increase in morphological and ecological diversity (Shen et al. Reference Shen, Dong, Xiao and Kowalewski2008; Bush et al. Reference Bush, Bambach, Erwin, Laflamme, Schiffbauer and Dornbos2011) at the organism level and increases in niche interactions (Gehling and Droser Reference Gehling and Droser2018; Mitchell et al. Reference Mitchell, Bobkov, Bykova, Dhungana, Kolesnikov, Hogarth and Liu2020; Boan et al. Reference Boan, Evans, Hall and Droser2023) throughout the Ediacaran and into the Cambrian (Bush et al. Reference Bush, Bambach, Erwin, Laflamme, Schiffbauer and Dornbos2011; Muscente et al. Reference Muscente, Bykova, Boag, Buatois, Mángano, Eleish and Prabhu2019; Buatois et al. Reference Buatois, Mángano, Minter, Zhou, Wisshak, Wilson and Olea2020; Nanglu et al. Reference Nanglu, Caron and Gaines2020b). These patterns are also captured at the macroecological scale (Na and Kiessling Reference Na and Kiessling2015; Eden et al. Reference Eden, Manica and Mitchell2022; Meek et al. Reference Meek, Buatois, Mángano and Eglington2023), demonstrating the buildup of these micro- to macroecological interactions.
By Cambrian Epoch 2, the ecosystem structure is similar in many aspects to that of marine systems today. However, while many of these the key ecological processes, such as predation, habitat modification, facilitation, symbiosis, mutualisms, and commensalism are present (Fig. 4), there are time delays between the origins of such processes and the time when they have an observed impact on other organisms—for example, reef production predates the advent of reef/hard substrate habitation by ~40 Myr, and complex trophic structures emerged around 30 Myr after the advent of predation. As such, while the key building blocks of ecosystem structure were in place by the end of the Cambrian, it takes evolutionary timescales for the impact of them to be realized.
Acknowledgments
This work was funded by the Natural Environment Research Council Independent Research Fellowship NE/S014756/1 to EGM and NE/X017745/1 to S.P. The authors would like to thank K. Nanglu and J. Ortega-Hernández (Harvard University) for useful discussions relating to this paper. We thank F. Anthony for the reconstruction in Figure 4.
Competing Interests
The authors declare no competing interests.