Introduction
Varroa destructor Anderson and Trueman (Reference Anderson and Trueman2000), hereafter Varroa, is an obligate ectoparasitic mite of the honey bee, Apis mellifera, and has been widely reported to be the major factor behind the continued poor health of this species, and collapse of honey bee colonies throughout most regions of the world (Allen-Wardell et al., Reference Allen-Wardell, Bernhardt, Bitner, Burquez, Buchmann, Cane, Cox, Dalton, Feinsinger, Ingram, Inouye, Jones, Kennedy, Kevan, Koopowitz, Medellin, Medellin-Morales and Nabhan1998; Gallai et al., Reference Gallai, Salles, Settele and Vaissiere2009; Nazzi and LeConte, Reference Nazzi and LeConte2016). Honey bees are essential for pollinating most domestic fruit and vegetable crops. Consequently, the continuing loss of honey bee colonies from Varroa parasitism (Varroosis) threatens a potential catastrophic loss of billions of dollars’ worth of crop production (Nazzi and LeConte, Reference Nazzi and LeConte2016; Rozenkranz et al., Reference Rozenkranz, Aumeier and Ziegelmann2010). The threat of Varroa mites to the bee keeping industry has stimulated much research to understand Varroa mite biology and to develop methods for its control.
A search of the existing literature to date (2018) revealed relatively few articles relating to Varroa nutritional biology (see Evans and Cook, Reference Evans and Cook2018 for a review). Varroa mites ingest a slurry of hemolymph containing dissolved nutrients, predigested host tissues and cellular components (Ramsey et al., Reference Ramsey, Culbronson, Mowery, Ochoa, van Engelsdorp and Bauchan2018). Comparison of the fatty acid composition between Varroa and their honeybee hosts suggests that targeted feeding on host fatty acids may occur (Dmitryjuk et al., Reference Dmitryjuk, Zalewski, Raczkowski and Zoltowska2015). Other clues to the nature of Varroa nutrition include the presence of metabolic enzymes, including esterases, especially cholinesterases, carboxylesterases, alkaline and acid phosphatases, believed secreted by the mites' salivary glands (Dmitryjuk et al., Reference Dmitryjuk, Zoltowska, Fraczek and Lipinski2014). Additional studies by Fraczek et al. (Reference Fraczek, Zoltowska and Lipinski2009) revealed evidence of up to 19 hydrolases that were found in both honey bees and Varroa mites that feed on them, and the activities of certain hydrolases, e.g. glycosidases, were higher in the parasite than in the honey bee hemolymph. Also, the inhibition of the activity of bee hemolymph proteinases by Varroa extracts has been observed (Fraczek et al., Reference Fraczek, Żółtowska, Lipiński and Dmitryjuk2012). A comprehensive study of the carbohydrate metabolism of Varroa detected two major glycogen metabolism enzymes, glycogen phosphorylase and glycogen synthase, along with other important findings regarding their lipid metabolism (Lopieńska–Biernat et al., Reference Lopieńska–Biernat, Dmitryjuk, Zaobidna, Lipiński and Żółtowska2013). Additional work has provided details of the sterol composition and synthesis capacity of host honey bees and Varroa mites (Hartfelder and Feldlaufer, Reference Hartfelder and Feldlaufer1997). Care should be exercised when interpreting Varroa nutrition based on the presence of certain metabolic enzymes, particularly those of host origin, as these may not be functional enzymes but simply represent undigested proteinaceous ‘food’ (see, Erban et al., Reference Erban, Harant, Hubalek, Vitamvas, Kamler, Poltronieri, Tyl, Markovic and Titera2015). In general, since the life history of Varroa is tightly linked to that of its host, understanding honey bee nutrition may provide basic insights into Varroa nutrition (see Wright et al., Reference Wright, Nicholson and Shafir2018 for a review). Finally, mite nutrition may also be supplemented by contributions from their microbiomes, which can differ in composition to that of their hosts (Hubert et al., Reference Hubert, Kamler, Nesvorna, Ledvinka, Kopecky and Erban2016), or may affect the composition of host's microbiome (Hubert et al., Reference Hubert, Bicianova, Ledvinka, Kamler, Lester, Nesvoran, Kopeckyi and Erban2017). Direct measures of microbial contributions to Varroa nutrition have not yet been undertaken.
Studies of ingesta is not the only means by which one can learn about animal nutritional biology; analyzing both the chemical composition of animal excreta and characterizing behaviours of excreta deposition, can inform researchers of an animal's nutritional balance (i.e. nutrient excesses), and provide insights into their metabolism, and possibly their hygienic behaviours. However, no studies have fully characterized the chemical makeup of Varroa excrement or investigated the excretory behaviours of Varroa during feeding, revealing that much still remains to be learned about Varroa nutritional biology and behaviour. Hence, here we characterize the chemical (purine) composition of Varroa excreta, observe the frequency of excreta deposition, and quantify and estimate mass and volumes, respectively, of individual deposits. Our findings will likely provide important insights into the metabolism of ingested bee tissues in the feeding mites.
Materials and methods
Collecting mites and honey bee pupae
Female Varroa mites and honey bee host pupae were obtained from honey bee colonies located on grounds of the United States Department of Agriculture-Agricultural Research Service (USDA-ARS), Beltsville Agricultural Research Center, Beltsville, Maryland, USA, that were untreated with acaricides to control Varroa infestations. Phoretic stage (sensu lato) mites used in experiments were collected from approximately 300 adult honey bees using the sugar roll method as described elsewhere (Dietemann et al., Reference Dietemann, Nazzi, Martin, Anderson, Locke, Delaplane, Wauquiez, Tannah, Frey, Ziegelmann, Rosenkranz, Ellis, Dietemann, Ellis and Neumann2013). Mites washed of sugar were collected onto moist tissue paper lining a petri dish and transported to the laboratory. Honey bee host pupae were obtained from the same untreated honey bee hives as the mites. To do so, a frame having a large number of capped worker cells was removed, and cells gently opened to find white or pink-eye pupae, which were removed from their cells using soft forceps (Bioquip, USA) to minimize injury during extraction. All pupae were placed on moist tissue paper in a petri dish and transported to the laboratory. Back at the laboratory, both mites and pupae were inspected, and moribund mites and pupae showing evidence of injury were discarded. These same quality control procedures were implemented regularly for all mite and host collections. Using soft forceps, healthy pupae were transferred to gelatin capsules (size 0, Thermofisher Scientific, Waltham, Massachusetts 02451, USA). A single female mite was placed on pupa using a paint brush, then capsule closed by attaching the lid. Subsequently, the pupa and mite-filled capsules were placed in an Heratherm IGS-100 incubator (Thermo-Electric, Langenselbold, Germany) at 32 °C. and ~75% RH (Egekwu, et al., Reference Egekwu, Posada, Sonenshine and Cook2018).
Excretory behaviours
A total of 12 Varroa were monitored over a 10-day experimental period. Each day the number of excretory deposits was recorded and whether each occurred on the capsule wall or on the host pupa. Additional measurements included weight and volume estimates of deposits made on, and collected from, the surface of hosts; weighed deposits were stored for subsequent analysis of their chemical composition. Excretory deposits on the capsule wall dissolved the gelatin, or were smeared, and thus not used for making physical measurements, nor included in the chemical analysis. To mitigate the risk of double counting deposits or compromise their chemical make-up, mites were transferred daily to a new capsule containing a fresh honey bee pupa. Statistical analyses of the differences in daily deposition rates and rates between capsule and pupa were determined by the General Linear Model and the Duncan Multiple Range test.
Excreta deposit volume and weight
Each day, excretory deposits on the surfaces of the host pupae were observed under a 50X stereoscope and carefully removed using a fine heat-sterilized needle and then transferred to a stage micrometer (0.02 mm delineations) (MSWM2 stage micrometer, Microscope World Corp.). Deposits on host integument were often circular or subcircular in shape (Supplymentary Fig. S1); the diameter of each deposit was measured, and from this the volume of each deposit was estimated using the formula 4/3 πr 3. Subsequently, excreta deposits were collected to a 1.5 mL microcentrifuge tube pre-weighed (± 0.1 mg) on an Ohaus Discovery Model DV-114C (Parsippany, New Jersey, USA) semi-microbalance. Excretion deposits collected from all pupae each day were amassed into the same tube and allowed to air dry for 3 h, after which the samples were re-weighed. The empty tube weight was subtracted from this final weight to determine the weight of the excreta deposited on host pupae. The same procedure was followed for each successive day of the experiment. Excreta deposits on the capsule wall could not be collected for weight/volume measurements (above). However, their total weight was estimated by taking the weight of a single deposit (calculated average individual excreta weight for deposits collected from pupae) and multiplying this value by the number of excreta deposited on the capsule wall.
Chemical analysis of mite excreta
High-performance liquid chromatography coupled mass spectrometry with electrospray ionization (HPLC-MS/MS), together with multiple reaction monitoring (MRM) were used to detect and quantify purines in excreta samples. For this analysis, the more than 100 dried excretory deposits collected into separate microcentrifuge tubes on days 3, 6 and 9 from the host pupae were then dissolved in 1 mL 10% NH4OH added to the tubes. The three samples of V. destructor excreta extracts were submitted to the USDA-ARS Sustainable Agricultural Systems Laboratory, Beltsville, Maryland, USA for chemical analysis. In addition, authentic samples of guanine, hypoxanthine and xanthine (Sigma/Aldrich, St. Louis, Missouri, USA) were submitted for use as standards for comparison with the excreta samples. Prior to injection, the samples were diluted with methanol 1000-fold (samples 1 and 2) and 100-fold (sample 3). Subsequently, following the HPLC and mass spectroscopy procedures, the amount of guanine, hypoxanthine and xanthine in excreta samples was determined by comparison with purine standards.
Chromatographic separation of purines was performed on Waters Alliance e2695 HPLC (Waters Inc., Milford, Massachusetts, USA) using a reverse phase Phenomenex Luna C-8 column 4.6 × 150 mm, 3 µm (Phenomenex, Torrance, California, USA). The mobile phase was a binary gradient with solvent A composed of 90% H2O and 10% 50 mm aqueous ammonium acetate and solvent B composed of 90% acetonitrile and 10% 50 mm aqueous ammonium acetate. The gradient was as follows: 0–4 min 100% A, 4–8 min linear change to 1.5% B, 8–11 min linear change to 20% B, 11–21 min 20% B, 21–23 min linear change to 100% B, 23–28 min 100% B, 28–30 min linear change to 100% A and 30–40 min 100% A. The total run time was 40 min. The flow rate was 0.3 mL min−1. The temperature of the column was 20 °C. The injection volume was 10 µL.
The HPLC analysis was coupled with a Micromass model LC-Quattro Ultima triple quadrupole mass spectrometer with an ESI ionization (Waters Inc., Milford, Massachusetts, USA). The detection of purines was performed using MRM function in negative ionization mode for xanthine and positive ionization for hypoxanthine and guanine. The following parent–daughter ion transitions were used: M/z 151.1→108.1 (cone voltage 40 V, collision energy 15 V) for xanthine, M/z 137.1→119.1 (cone voltage 40 V, collision energy 19 V) for hypoxanthine and M/z 152.1→135.1 (cone voltage 40 V, collision energy 19 V) for guanine. Quantitation was performed by external standard methods without an internal standard. The source temperature was 140 °C and the desolvation temperature was 400 °C. Data were acquired and processed using MassLynx 4.1 software.
The concentration of guanine was determined using a 5-point calibration curve in the range from 0.05 to 1 ppm and linearity (r 2 = 0.999).
Results
Excretory behaviours
Attrition of mites began on the eighth evaluation day until only six and three mites remained alive on days 11 and 12, respectively. Despite this, on average each day mites deposited 18 excretions. However, over the 12-day evaluation period, the mean number of deposits made each day varied, showing two separate peaks occurring on days 2–5 and 8–9 (Fig. 1). In total, a slight trend was observed showing fewer daily deposits over time (F 11,108 = 1.69, P < 0.085). From the 120 total observations made of excretory behaviour, mites deposited excretions predominately on the body of their hosts (χ 2 = 118.70; df = 1, P < 0.0001); on average each day, mites deposited about 14 excretions on the pupae vs only about 4 on the gelatin capsules.
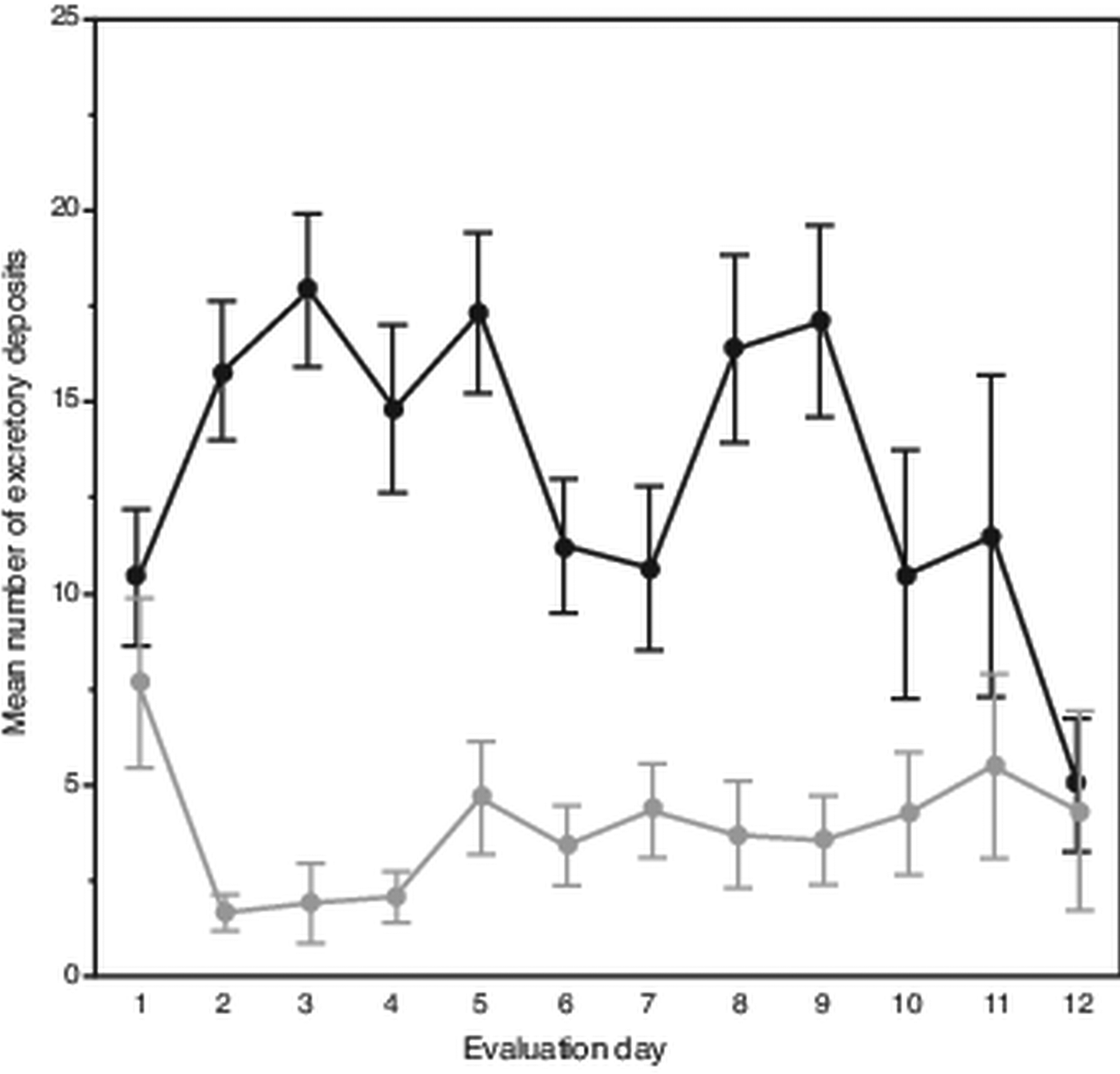
Fig. 1. Daily number and location of female Varroa mite excretory deposits. Mean (± s.e.m) daily deposits of waste excretions made by mites observed over 12 days on integument of host honey bee pupae (upper dark line) and on inner wall of gelatin capsule (lower light line) housing mites and hosts.
Excreta total weights and volumes
Because high attrition of mites in last 2 evaluation days led to numbers of excretions on pupae falling below a preset threshold (>100 total deposits from all mites each day; Table 1), no deposits were accumulated from pupae for weighing on evaluation days 11 and 12. However, for these days, the number of deposits were multiplied by the average weight of a single deposit from host pupae (5.9 µg ± 1.4 µg s.e.m). Estimated weights of deposits on capsule wall (using a number of deposits and estimated weight of a single deposit) was 79% (±3% s.e.m) accurate when extrapolating from actual weighed excreta removed from host's body. For this complete dataset, the trend for the cumulative excreta weights shows a positive continuous accumulation over the 12-day period without any lapses (i.e. deposits were not absent on any evaluation day) (Fig. 2A). The estimated weight of excreta deposited daily per mite is shown in Fig. 2B; excreta weights peaked on days 4 and 7 (each nearly 0.2 mg), then declined steadily to day 12.
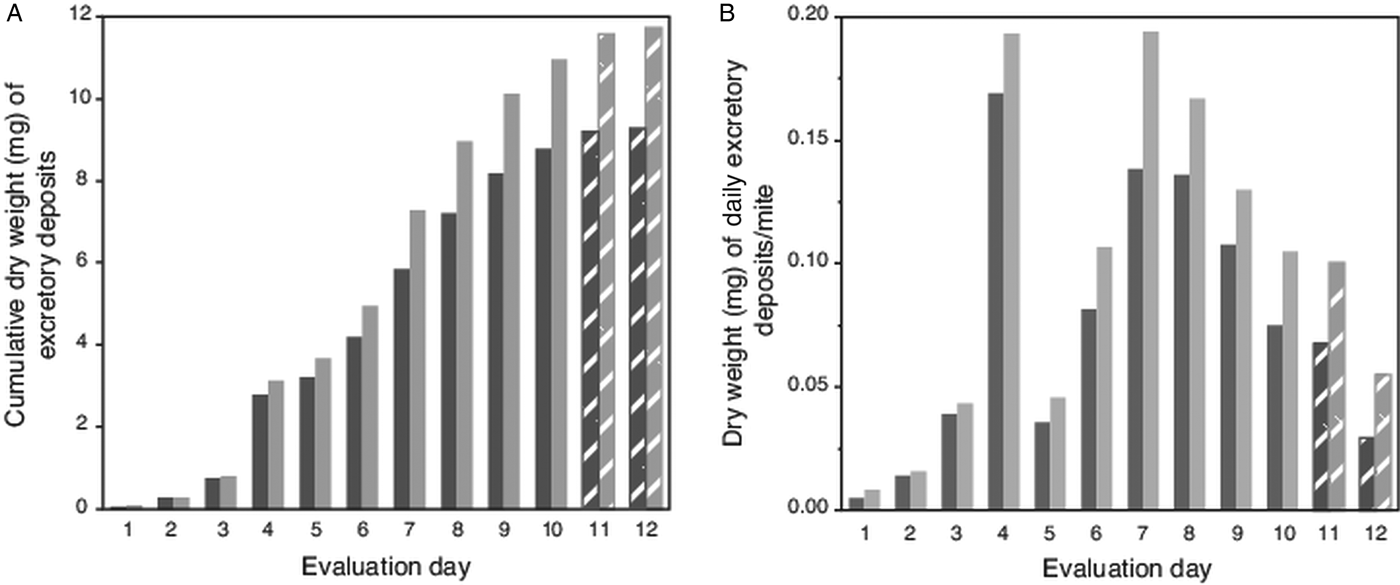
Fig. 2. Weight of cumulative total, and estimated weights of individual excretory deposits from female Varroa mites. (A) Cumulative weight of excretory deposits collected over 12 days from integument of host pupae (dark bars) and total weight of deposits (light bars) including estimated weights of deposits on capsule walls. (B). Estimated total weight of individual excretory deposits made daily by individual mites. Textured bars in both (A) and (B) represent estimated weights (see text).
Table 1. Number of excretory deposits made by individual female Varroa mites on either their honey bee pupa host or wall of gel capsule housing both pupa and mites over a 12-day monitoring period

The diameter was measured for an average of 17 individual circular/subcircular shaped excretory deposits (haphazardly selected subset of total deposits collected each day 1–10), resulting in calculated volumes ranging from a minimum of 0.002 µL to a maximum of 0.153 µL, with many deposits having small volumes (Fig. 3A). The mean volume of each deposit measured (N = 102) was 0.049 µL ± 0.004 s.e.m. If mites excreted 18 such deposits each day, compounded over the 12-day evaluation period, each mite would have consumed an estimated 10 µL of host fluid (Fig. 3B).
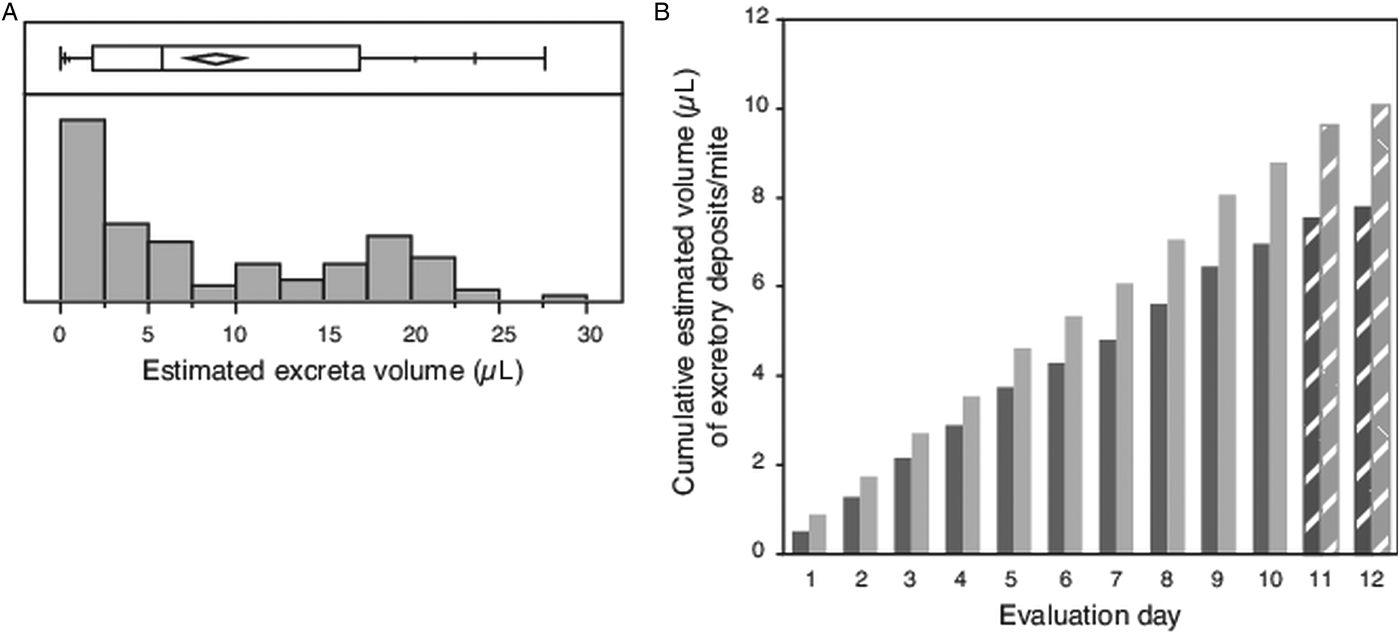
Fig. 3. Volume of individual excretory deposits from female Varroa mites. (A) Box plot giving range, quartiles and median of excretory deposit volumes calculated from the measured diameter of N = 102 circular/semi-circular excretory deposits, and the frequency distribution of these calculated volumes. (B) Cumulative volume of excretory deposits from individual mites over 12 days, estimated from the mean calculated volume of individual deposits and number of deposits made daily per mite. Textured bars in (B) are estimated data extrapolated from measured excreta from days 1 to 10.
Chemical analysis of mite excreta
Chemical analysis of the excretory deposits revealed the presence of guanine and traces of hypoxanthine, but no evidence of xanthine. The mass spectrum obtained from sample two closely matched the mass spectrum for the guanine standard (Fig. 4 and Supplymentary Fig. S2). For the other samples, slight deviations from the standard in the spectra are likely due to impurities in the samples. In addition, analysis of full scans and comparison with published mass spectra revealed the presence of uric acid and caffeine in all three samples (data not shown), and possibly an undetermined purine (mainly in samples 2 and 3). Guanine content in samples 1 and 2 was 0.23 and 0.18 mg mL−1, respectively. No evidence of guanine was detected in sample three. Analysis of the HPLC chromatogram and mass spectrum for MRM transition also showed guanine in both the standard and samples one and two. The MRM results are considered definitive; these results identified both the unique collision-induced fragment of guanine (the 135 m z−1 ion) determined from the standard, and the same 152 > 135 m z−1 ion chromatogram retention time as the standard. This retention time for guanine was also unique compared with the ionization fragments and retention time for hypoxanthine. Trace hypoxanthine identified in samples was not quantified.

Fig. 4. LC-MS analysis of purine standards and Varroa mite excreta samples amassed at day 3 (sample 1), day 6 (sample 2) and day 9 (sample 3), of a 12-day monitoring period. (A) LC-MS/MS chromatogram for MRM transition M/z 152.1→135.1 of sample 1 at 2.54 ppm. (B) sample 2 at 1.59 ppm, and (C) guanine standard at 0.25 ppm. Guanine was not detected in sample 3.
Discussion
To gain a better understanding of Varroa nutrition and excretory behaviour, we both characterized the purine composition of their excreta and described their excretory behaviours while housed with honey bee pupa hosts in the laboratory. One method to understand the nutritional biology and physiology of organisms is to identify the chemical components and their relative abundance in waste excretions. Guanine, hypoxanthine, xanthine and other purines serve as the nitrogenous wastes in ticks and other acarines (Kniest, Reference Kniest1995; Sonenshine, Reference Sonenshine, Sonenshine and Roe2014); high levels of purines in excreta may indicate a protein-rich diet and/or a high level of protein/nucleic acid metabolism. Guanine was also found to be abundant in the excretory waste of house dust mites, Dermatophagoides pteronyssinus and D. farinae (Quiox et al., Reference Quiox, Le Mao, Hoyet and Pauli1993). In contrast, for blood-feeding insects, such as kissing bugs (Triatominae), the primary nitrogenous waste is in the form of uric acid salts, e.g. potassium urate (Graça-Souza et al., Reference Graça-Souza, Silva-Neto and Oliveira1999; Bradley, Reference Bradley, Resh and Cardé2003). Previously, the waste excretions of Varroa mites were reported to consist of 95% guanine (Erickson et al., Reference Erickson, Cohn and Cameron1994). Our analyses indicate that the guanine content of excreta attenuated over time; the percentage of guanine in excreta collected on days 3 and 6 was 48.9 and 18.4%, respectively, and no measurable guanine was measured in excreta collected from day 9. In relation to this, we noted that mites began dying in large numbers after the 10-day monitoring period (coinciding with depletion of white material visible in the digestive tract of mites; Supplymentary Fig. S3). Although the reason for sudden increase in mite mortality is unknown, the attenuation of guanine content in excreta suggests that at latter days Varroa decreased feeding on its host, perhaps due to physical and/or physiological costs associated with making a new feeding hole each day. However, the estimated weight/volume of individual excreta did not show a corresponding decrease, suggesting that compounds other than those measured increased in prevalence. Indeed, unidentified purines appeared more abundant in the later samples (as noted above), raising the possibility of failure of metabolic processing as a major reason for mite mortality.
The chemical composition of Varroa excretions, besides providing basic information on mite nutritional biology and physiology, also can provide information for applied research. For example, in other acarines guanine can act as an arrestant pheromone alerting conspecifics to favourable locations to find new hosts (Leahy et al., Reference Leahy, Karuhize, Mango and Galun1975; Gray, Reference Gray1987; Dusbabek et al., Reference Dusbabek, Simek, Jegorov and Troshko1991; Allan and Sonenshine, Reference Allan and Sonenshine2002; Sonenshine et al., Reference Sonenshine, Adams, Allan, McLaughlin and Webster2003). Guanine may also serve as an assembly pheromone for Varroa, guiding other mites to infested brood (Sammataro et al., Reference Sammataro, Gerson and Needham2000; Yoder and Sammataro, Reference Yoder and Sammataro2003), or in an applied scenario to traps and/or poison-laced baits. In a different study, other authors have suggested that the presence of guanine in honey bee hives might act as a diagnostic tool for identifying infestations of the honey bee tracheal mite, Acarapis woodi (Mozes-Koch and Gerson, Reference Mozes-Koch and Gerson1997).
In terms of excretory behaviours, our data show that female mites defecated nearly four times more frequently on their hosts than on the capsule wall. This finding contrasts with the behaviour reported by others, who found that the mites preferred to deposit wastes in the posterior part of the brood cell (Nazzi and LeConte, Reference Nazzi and LeConte2016) or on the sides of the cells (Calderón et al., Reference Calderón, Fallas, Zamora, van Veen and Sánchez2009, Reference Calderón, Chaves, Sanchez and Calderón2012; Dieteman et al., Reference Dietemann, Nazzi, Martin, Anderson, Locke, Delaplane, Wauquiez, Tannah, Frey, Ziegelmann, Rosenkranz, Ellis, Dietemann, Ellis and Neumann2013). This behaviour may be adaptive, possibly to promote hygiene within the confines of the sealed host cell. Also, Calderón, et al. (Reference Calderón, Chaves, Sanchez and Calderón2012) hypothesized that reproductive females do not deposit wastes on the surface of hosts, and those that did so had reduced fecundity. Santilián-Galicia et al. (Reference Santillán-Galicia, Carzaniga, Ball and Alderson2008) reported that the honey bee pathogen Deformed wing virus was found located in large, dense spheres presumed to be excretory waste within the midgut of Varroa. If true, this may provide a better understanding of how host health is compromised, thus reducing mite fecundity. We also found evidence of granular material, presumably guanine, in the midgut of female mites (Supplymentary Fig. S3), similar to that shown by Erban et al. (Reference Erban, Klimov, Smrz, Phillips, Nesvorna, Kopecky and Hubert2016) in the stored product mites, Tyrophagus putrescentiae.
Monitored mites each made on average nearly 18 excretory deposits daily. The number of excretory deposits was similar each day over the monitoring period, suggesting female mites maintain a consistent feeding regiment. The mean volume per deposit, 0.049 nL ± 0.044, times an average of 18 excreta deposits for each mite per day, represents an average of 10 µL of waste excreted over 12 days. Comparing excreta volume to mass, which was estimated as >1 mg dry weight over 12 the water-saving properties of purines becomes apparent. Nevertheless, water loss from mite feeding, together with wounds remaining open via mite released anticoagulating proteins (Yang and Cox-Foster, Reference Yang and Cox-Foster2005) can equate to a substantial moisture loss from hosts, which may be injured as a result. Frequent daily excretion observed here supports data showing mites feed frequently from their hosts (Calderón et al., Reference Calderón, Fallas, Zamora, van Veen and Sánchez2009), or have a high metabolic turnover. Moreover, our data suggest that mites do not store any ingested food, but ingest relatively large volumes of food daily and frequently excrete the waste products. These behaviours and any associated internal anatomy might be necessary given the tight and crowded quarters inside capped honey bee pupa cells (Calderón et al., Reference Calderón, Fallas, Zamora, van Veen and Sánchez2009).
The high nitrogen content of Varroa excretions, together with the frequent deposition of large volumes of excreta, is reminiscent of filter-feeding insects, such as aphids, which strain carbohydrate (sugar) rich plant phloem for the trace amino acids and other nutrients it contains and expel sugar-rich honeydew. As presumably non-reproducing (or growing) phoretic-stage mites were used in our experiments (no eggs were noted at any point during monitoring period), it is unclear what nutrients, if any, mites may be straining from ingested host fluids. If female mites were actively reproducing, it could be predicted that the frequency, and perhaps volume and chemical composition of excretory deposits may differ; ingested protein may not be readily discarded, but rather shunted into forming several large, nutrient-dense eggs. Future work should determine the complete chemical composition of Varroa excreta, to provide clues of not only protein metabolism, but that of other nutritive substrates, such as lipids. These data, together with information of differences in feeding, and thus excretory behaviours between ‘phoretic’ and reproducing ‘foundress’ female mites, may help to distinguish the nutritional requirements of mites in these contrasting life stages. Finally, future work should also investigate the unknown, specific contributions of the mite's microbiome and host enzymes, if any, to the nutrition of Varroa mites.
Supplementary material
The supplementary material for this article can be found at https://doi.org/10.1017/S0031182018001762.
Acknowledgements
We would like to acknowledge Curtis Rogers of the USDA-ARS Bee Research Laboratory, Beltsville, Maryland, USA, for helping with the graphics.
Financial support
Francisco Posada-Florez would like to express his gratitude to ORAU/ORISE Fellowship awarded through the USDA-ARS.
Conflict of interest
None.
Ethical standards
None.