Introduction
It's now been 25 years since we prepared a spherical container compound – the ‘softball’. It was not our first synthetic container, but it was the first large enough to confine two molecules inside and it triggered our study of chemistry – bond making and breaking – in isolation. Many enzymes were known that could fuse two molecules together as substrates, but synthetic vessels that promoted bimolecular reactions were rare (Mock et al., Reference Mock, Irra, Wepsiec and Manimaran1983; Breslow and Guo, Reference Breslow and Guo1988), and incapable of completely surrounding and isolating reactants. By isolation we mean confinement by reversible encapsulation of molecules in small spaces, rather than, say, isolation through very low pressures in the gas phase. And by small spaces we mean container compounds which more or less completely surround a well-defined volume, rather than open-ended compounds. This is a review of our experimental probes of molecules confined in these containers enhanced by the computational observations of our collaborators. The results led to the notion that 55% represents an optimal filling of space in solution, about which more, later.
Reaction
The earliest container we prepared was the notional ‘tennis ball’, a dimeric capsule that could bind molecules no larger than ethane (Wyler et al., Reference Wyler, Mendoza and Rebek1993). But when we had in hand the proportionally larger softball (Fig. 1) (Meissner et al., Reference Meissner, Rebek and Mendoza1995) and found it accommodated one adamantane or two molecules of benzene inside, who could resist its use as a reaction vessel? A paradise for reacting species – what could be better for a cycloaddition reaction? The translational and rotational motions of the reactants are coupled by containment; entropic prices for bringing reactants together are paid for by the forces of encapsulation and the costs of solvent reorganization at the transition state are of no concern, since the container is the solvent cage, arranged carefully by synthesis. And it gets better. Concentrations? Great – the volume of the softball is 3.5 × 10−25 l so any molecule in this space enjoys a concentration of >4 M. Timing? Luxurious – the lifetime of the capsular assembly is >1 s compared to the nanosecond lifetime of a diffusion complex in a typical solvent cage. These benefits for the reaction of cyclohexadiene with p-benzoquinone are summarized in the cartoon of Fig. 1.

Fig. 1. (a) Chemical structure of the monomer of the softball. (b) Modeled structures of the softball dimer (left) and with adamantane guest (middle). Cartoon of co-encapsulated cyclohexadiene and p-benzoquinone (right). From Kang et al. (Reference Kang, Hilmersson, Santamaria and Rebek1998a, Reference Kang, Santamaria, Hilmersson and Rebek1998b).
And the reaction did succeed (Kang and Rebek, Reference Kang and Rebek1997), with regiospecificity in several contexts (Kang et al., Reference Kang, Hilmersson, Santamaria and Rebek1998a), and in one case even with (feeble) catalytic turnover (Kang et al., Reference Kang, Santamaria, Hilmersson and Rebek1998b). Most cases showed classic product inhibition because two reactant molecules were unable to displace the single, well-fitting product (Kang and Rebek, Reference Kang and Rebek1996). Other peculiarities made this case difficult to study quantitatively. For example, the resting state of the capsule contained two molecules of the quinone inside and the ‘Michaelis complex’ shown in the cartoon was never actually observed. To be sure, the reaction inside was thousands of times faster than the reaction proceeding outside the container (reflecting molar versus millimolar concentrations) but there is an entire cottage industry for setting up systems to calculate, interpret, and exaggerate rate enhancements. We did not pursue these analyses with the softball. It was not until we had a container of the right shape and size to favor the Michaelis complex – one molecule of each reactant observed inside – that we had confidence in kinetic analyses. This was accomplished with a cylindrical capsule (Fig. 2) (Heinz et al., Reference Heinz, Rudkevich and Rebek1998).

Fig. 2. (a) Chemical structure of a resorcinarene (top) and its vase-shaped, deep cavitand (bottom). (b) The shape and dimensions of the space inside the cylindrical capsule formed through hydrogen bonding. (c) Cartoon of dicyclohexylcarbodiimide in the capsule. Adapted from Heinz et al. (Reference Heinz, Rudkevich and Rebek1998).
The cylindrical capsule is prepared from a resorcinarene platform by building walls with hydrogen bonding functions on the periphery. The vase-shaped structure known as a cavitand can dimerize through a cyclic seam of hydrogen bonds. The space inside is tapered at the ends as shown in the figure and the shape controls the alignment of guests inside. For example, a long molecule such as dicyclohexylcarbodiimide is readily accommodated, but two (or even three) smaller molecules such as chloroform can be arranged inside – about which more later. For the moment, the cylinder takes in two phenyl acetylene or two phenyl azide guests aligned as shown in Fig. 3a. But, a mixture of the two symmetrically-filled capsules spontaneously disproportionate (K D) favoring the Michaelis complex of the newly introduced, but not yet a famous ‘click’ reaction (Kolb et al., Reference Kolb, Finn and Sharpless2001).
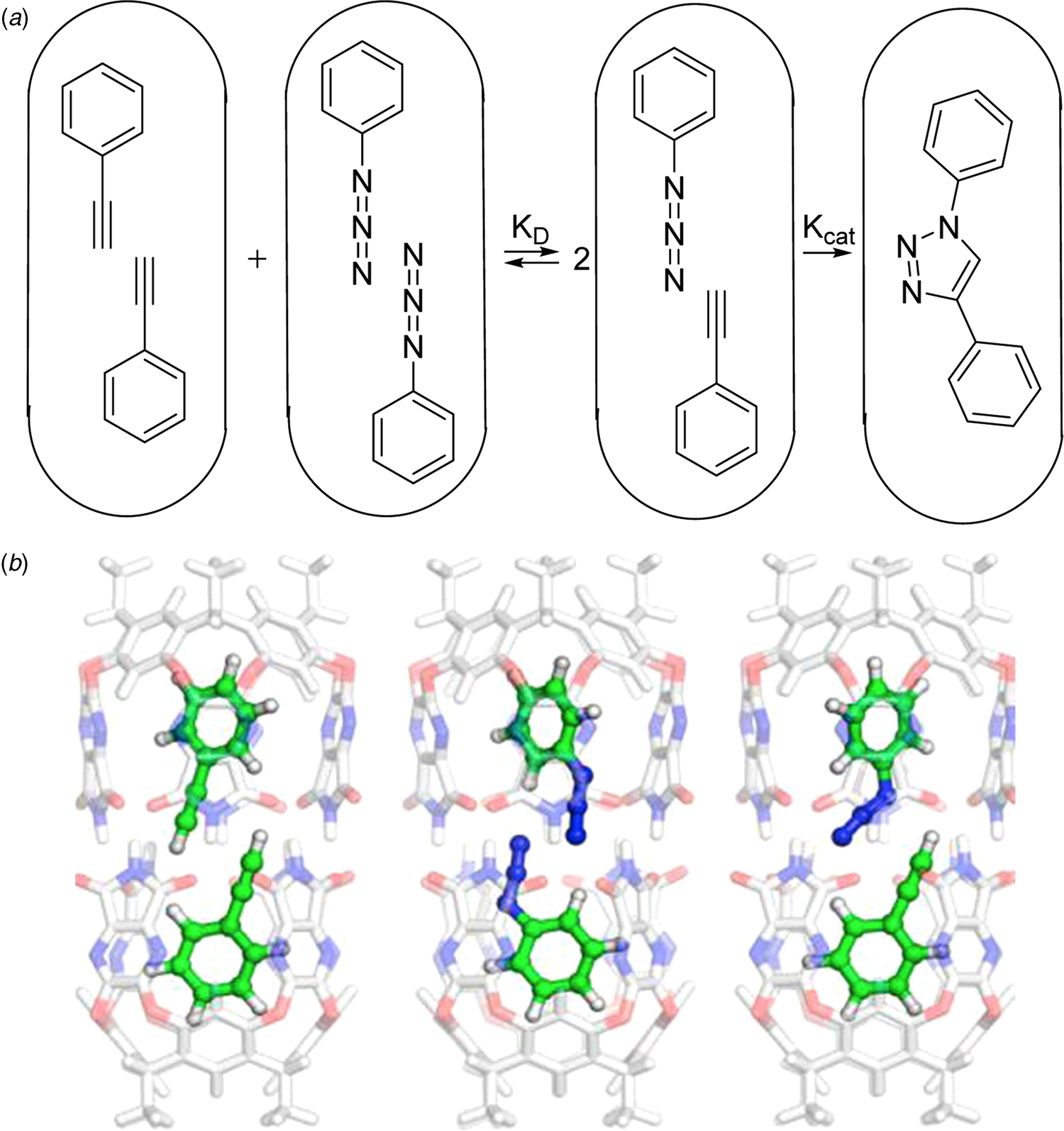
Fig. 3. (a) Cartoons of the encapsulated components of the click reaction. (b) Energy-minimized models for disproportionation of encapsulated acetylene (left) and azide (middle) to give the Michaelis complex. From Daver et al. (Reference Daver, Harvey, Rebek and Himo2017).
The reaction proceeds inside to give a single regioisomer which eventually fills the capsule (Chen and Rebek, Reference Chen and Rebek2002). At the millimolar concentrations used in the experiment, the reaction inside the capsule is calculated to proceed at a rate 240 times faster than that outside the capsule. The acceleration (not catalysis) of the reaction in the capsule caught the attention of theoreticians Fahmi Himo, Jeremy Harvey and collaborators and triggered computational analysis in Fig. 3b (Daver et al., Reference Daver, Harvey, Rebek and Himo2017). In particular, the question they raised was why – given the advantages listed above – was the reaction not faster? The answer emerged as follows.
Initially, the resting states of the capsule were calculated, and with guest solvents present, a previously unrecognized approximately C4-symmetric geometry for the capsule was identified as the most stable complex. In this complex, the walls of the capsule collapse to make better contacts with guests and the structure is maintained also when other guests bind inside.
Rearrangement
The second reaction studied inside was an intramolecular one, the rearrangement is shown in Fig. 4 (Sather et al., Reference Sather, Berryman, Ajami and Rebek2011). The reaction involves a 1,3 N → O acyl shift of a N-nitroso amide but in simpler, visually accessible terms the rearrangement makes the molecule thinner and longer. We had expected that substrates that fit snugly in the capsule would be less likely to undergo the step, although mere inhibition of the reaction was not our goal. The simple fact that the capsule alters the course of the reaction speaks volumes-reactions inside confined spaces are different whether enzymes or synthetic containers as shown capsules and deep cavitands – have emerged as the most realistic models of enzymes active sites. The use of small molecule compounds in dilute solutions to model enzyme reactions behavior is inadequate. Encapsulated compounds had been used with photochemistry as the trigger (Kaanumalle et al., Reference Kaanumalle, Gibb, Gibb and Ramamurthy2005), to show how confinement affects reaction pathways in a number of processes (Ramamurthy, Reference Ramamurthy2015; Mohan Raj et al., Reference Mohan Raj, Sharma, Prabhakar and Ramamurthy2019). These features comprise high-binding selectivity, large rate enhancements, and stabilization of reactive intermediates.

Fig. 4. The rearrangement involving a 1,3 N → O acyl shift of a N-nitroso amide.
The calculations nicely reproduced the inhibition of the decomposition inside the capsule (Brea et al., Reference Brea, Daver, Rebek and Himo2019a, Reference Brea, Daver, Rebek and Himo2019b). They confirm that the inhibition is due to an increase in the energy barrier of the 1,3 N → O acyl transfer to form the diazoester intermediate, which is the rate-determining step of the reaction. A distortion–interaction energy breakdown was used to analyze the energy barrier increase. The calculations also predicted that ion pairs that are unstable in mesitylene solution can be stabilized in the capsule by nearby hydrogen bonds. That is, confinement favors (hypothetical) intermediates that might form inside the capsule but are unexpected in solution. The details of the reaction outside were thoroughly examined by density-functional theory (DFT) calculations (Brea et al., Reference Brea, Daver, Rebek and Himo2019a, Reference Brea, Daver, Rebek and Himo2019b).
New stereochemistries
The cylindrical shape and volume of the capsule offered sustained arrangements of small molecules confined in its space. These arrays represent new forms of stereochemistry that are unknown in bulk solution – an emergent aspect of the behavior of confined molecules. First, molecules such as p-ethyl-toluene are taken in are too long to tumble in the capsule but leave enough space for a second guest, say, chloroform (Fig. 5b). Two arrangements can be observed in the NMR spectrum – one with the ethyl near the chloroform (80%) and one with the methyl near the chloroform (20%). The two occupants are too wide to slip past each other while in the container, so the isomeric arrangements are stable as long as they remain isolated, in the container. They can interconvert only by exiting the capsule and re-entering in the other arrangement, a process that occurs on the timescale of seconds. The ratio of the two isomers reaches equilibrium on mixing the components and allows calculation of small differences in energy between what we call ‘social isomers’. We examined a series involving 15 pairs of co-encapsulated guests and measured their social isomers in the container. The energetics of their pairwise functional group interactions could be evaluated at the sub-kilocalorie level. Much of what we know of these guest–guest interactions is due to our computational collaborators Giannoula Theodorakopoulos and Ioannis Petsalakis in Athens who provided the theoretical underpinnings for forces acting in small spaces (Tzeli et al., Reference Tzeli, Theodorakopoulos, Petsalakis, Ajami and Rebek2011).

Fig. 5. (a) Cartoons of constellational isomers ‒ chloroform with isopropyl chloride in cylindrical capsule. (b) Cartoons of social isomers ‒ chloroform with p-ethyl-toluene in cylindrical capsule. (c) Cartoons of rotational isomers ‒ two β-picoline in a cylindrical capsule.
Second, the cylindrical container's ability to accommodate three small molecules gave rise to yet another form of isomerism. For example, three chloroform molecules are taken up, and even though they are able to tumble freely in the space, they are too large to slip past each other while inside. The centrally-located guest stays there and shows a separate NMR signal from those guests at either end of the space. Now, suppose that a second guest is introduced to replace a resident chloroform molecule. We used isopropyl chloride since it is of comparable size and shape and polarity. Two new complexes were formed as there are two positions for the new guest: center or end. We called these constellational isomers as shown in Fig. 5a.
Third, co-encapsulation of the various picolines provided an opportunity to test another form of isomerism in the shape-space of the capsule. Aromatics fit each half of the container best when diagonally positioned (Fig. 5c). These guests are short enough to tumble freely inside, but the space of the container – two square prisms rotated by 45° does not allow the two aromatics to be coplanar (Tzeli et al., Reference Tzeli, Theodorakopoulos, Petsalakis, Ajami and Rebek2012). Instead, the ground state arrangements of β-picoline can place the nitrogen atoms at proximal or distal positions, as shown. This introduces new stereochemistry, ‘rotational isomerism’ for guests with hindered rotation along the capsule's long axis. The computations of the Athens group showed the preferred arrangement of the complexes in agreement with experiment (Fig. 5c) but also revealed guest–guest interactions and even unexpected host–guest hydrogen bonding for β-picoline (Ajami et al., Reference Ajami, Theodorakopoulos, Petsalakis and Rebek2013).
These emergent behaviors arise from the size and shape of the container's space operating on the confined guests. Why new names? In a formal view, the cases above are diastereomers, writ large, since they differ in ‘connectedness’. But rather than covalent connectedness, which is the test in classical chemistry, it is container connectedness in the chemistry of capsules. Carceroisomerism (Timmerman et al., Reference Timmerman, Verboom, van Veggel, van Duynhovan and Reinhoudt1994) has also been proposed to describe the different arrangements of molecules at such close range.
Solvent models
Incorporation of charged groups on the feet (periphery) of the containers and functions on the ‘rim’ that can hydrogen bond to water made water-soluble containers available. We used a rim featuring ureas (benzimidazolones) to form a formidable seam of hydrogen bonds (Ebbing et al., Reference Ebbing, Villa, Valpuesta, Prados and de Mendoza2002), a pattern introduced by De Mendoza. The expectation was that co-operativity and multivalency in rim-to-rim interactions of a dimeric capsule would overcome the hydrogen bonding to the ocean of water. In fact, millimolar water solubility resulted and an intact capsule persists with longer guests. In Gibb's cavitands and ours, hydrophobic processes drive the confinement and choices are made constantly between open-ended cavitand and more or less sealed capsules for quarantine. For our cases, shorter hydrocarbons form 1:1 cavitand complexes and longer alkanes 2:1 (capsular) complexes, but for n-decane, an equilibrium was observed between the 1:1 and 2:1 complexes, see Fig. 6a.

Fig. 6. (a) Chemical structures for benzimidazolone cavitand 1 (top); an equilibrium between the 1:1 and 2:1 complexes of n-decane and cavitand 1 (bottom). (b) Computational models for n-decane in cavitand 1 with different numbers of explicit water molecules. Adapted from Daver et al. (Reference Daver, Algarra, Rebek, Harvey and Himo2018).
Himo and collaborators realized that advanced computational protocols were prone to give very large errors arising from an implicit solvation model in water (Fig. 6b). Accordingly, a mixed explicit–implicit solvation protocol was developed. The explicit waters were placed around the rim in ways that were indicated to stabilize the vase conformation and observed in the X-ray structures of related cavitands. These waters bridge adjacent heterocyclic walls. The parameterization of the hydration free energy of water is set such that water cluster formation in water is predicted to be thermoneutral (Daver et al., Reference Daver, Algarra, Rebek, Harvey and Himo2018).
Magnetic environments of containers
Of the many forces recruited to assemble container structures, none is more exotic than chalcogen bonding (CB). Closely related to halogen bonding, CB made desultory appearances in other supramolecular assemblies, before it was introduced into capsules by Diederich, who recognized the 2,1,3 Z-benzodiazole as just another o-disubstituted panel with self-complementary assembly potential (Riwar et al., Reference Riwar, Trapp, Root, Zenobi and Diederich2018) (Fig. 7a). Using Z = S and Te, he found weak and strong CB, respectively, of capsules organic media and the solid state. Our own efforts – in water – began with the synthesis of Z = Se and a curiosity about the compatibility of CB with the powerful forces of hydrogen bonding.

Fig. 7. (a) Dimerization of the 2,1,3-benzochalcogenadiazole motif to give a capsule. Adapted from Riwar et al. (Reference Riwar, Trapp, Root, Zenobi and Diederich2018). (b) Typical upfield chemical shifts (−Δδ ppm) experienced by nuclei different depths in cavitands with heterocyclic walls. (C) Upfield shifts of encapsulated n-nonane calculated with PBE0/6-31G(d,p): benzimidazolone (top), benzoselenadiazole (middle), and pyrazine imide (bottom). From Rahman et al. (Reference Rahman, Tzeli, Petsalakis, Theodorakopoulos, Ballester, Rebek and Yu2020).
The CB capsules proved to be robust and ‘leakproof’ in water (D2O); the CB donors and acceptors were orthogonal to those of hydrogen bonding. The magnetic environment within the CB capsule, however, showed a peculiarity: unlike in capsules with benzimidazolone or pyrazine imide walls depicted in Fig. 7b, guest nuclei in the center of the space (near the Se atoms) showed sizable upfield shifts in their 1H NMR spectra. To understand the observed NMR chemical shifts, the Greek collaborators performed DFT calculations of capsular complexes corresponding to the three aromatic panels. The dimeric capsules studied used one molecule of n-nonane (C9H20) in an extended conformation inside. The computational approach involved DFT calculations using two functionals, M062X and PBE0, in conjunction with the 6-31G(d,p) basis set. The calculated geometries of the complexes and the computed 1H NMR chemical shifts of the guest are shown in Fig. 7c (Rahman et al., Reference Rahman, Tzeli, Petsalakis, Theodorakopoulos, Ballester, Rebek and Yu2020). The computations and experimental values are in excellent agreement.
Filling space
It has become increasingly clear that the behavior of molecules in dilute aqueous solution does not reflect their behavior in confined spaces. The synthetic receptors recognize and fold around their target guests, isolate them from the bulk solvent, provide a hydrophobic environment and present the guests with each other fixed in arrays set by the limited space. These features combine to show high-binding selectivity, large rate enhancements, and stabilization of reactive intermediates – resembling enzymes and receptors. The rules that govern capsule assembly are written in the hydrogen bonding patterns and the curvature of the modules, but the final instruction is the proper filling of the space inside. Recognition – size, shape, and chemical surface complementarity – lies at the heart of the confinement phenomena. It requires a good fit between guest and container, but what makes a good fit? For rigid guests, the size estimates are accurate; the dimensions are fixed and cannot exceed those of the cavity. For flexible guests, folding, bending, and other contortions are often encountered. The hosts are not rigid; they collapse on the guest to make whatever contacts are on offer at favorable van der Waals distances. Some time ago, we proposed 55% occupancy based on limited data and crude estimations of volumes (Mecozzi and Rebek, Reference Mecozzi and Rebek1998). This figure corresponds to that encountered in typical organic liquids but the estimate has spread in the supramolecular community and even beyond academia to medicinal chemistry (Ehmke et al., Reference Ehmke, Kilchmann, Heindl, Cui, Huang, Schirmeister and Diederich2011). We would be happy to have theoretical community rescue us with a better formulation.
Conclusions and predictions
The water-soluble capsules discovered by Gibb (Jordan and Gibb, Reference Jordan and Gibb2015), who mapped the contortions of guests in his capsules (Wang and Gibb, Reference Wang and Gibb2017) and applied them as protecting groups (Liu et al., Reference Liu, Gan, Hermann, Rick and Gibb2010). Atoms buried deep in the cavitand are hidden from reagents while atoms near the middle of capsules can be exposed to reagents in the solvent. The chemistry of folded molecules is a relatively new undertaking. Folding brings the guest's ends closer together, and sites that are remote in bulk solution are no longer so in the container. The two ends are subject to mutual neighboring group participation, a form of entanglement (Yu and Rebek, Reference Yu and Rebek2018). Product distributions that are statistically determined in solution are significantly altered in cavitands (Shi et al., Reference Shi, Mower, Blackmond and Rebek2016) and chaperoned macrocyclization has given access to molecules that cannot be made by conventional means. Amplification and chain-reaction kinetic have also been encountered (Chen et al., Reference Chen, Körner, Craig, Rudkevich and Rebek2002). But any number of container compounds – MOFs, COFs, or capsules made of metals and ligands – can exhibit properties of confined molecules and expand the research beyond chemistry to materials science.
For speculation, we propose applications in data storage. The various forms of isomerism described above represent information. With three different guests in the cylindrical capsule, 18 constellations can be arranged. This is multiplied many times if social and rotational isomers are possible – all in a space of about 2 nm3. This application requires that the isomers be ‘written’ rapidly and accurately, stored indefinitely and ‘read’ instantly. The speed of these processes (determined by the motion of molecules) is at a disadvantage compared to electronic motion, but the space involved represents an advantage. The challenges are monumental but the rewards may be momentous.
Acknowledgements
The authors warmly thank collaborators Jeremy Harvey, Fahmi Himo, Ioannis Petsalakis, Giannoula Theodorakopoulos, and their coworkers for computational studies. They inspire us. We are indebted to our own students and postdoctorals for their dedicated experimental work. They sustain us. Their names can be found in the original publications cited. Financial support was provided by the US National Science Foundation (CHE 1801153), the National Natural Science Foundation of China (Grant No. 21801164), and Shanghai University (No. 13-G210-19-230). Yang Yu thanks the Shanghai Education Committee for a Special Professor Appointment (Dongfang Scholarship).