INTRODUCTION
The climate of eastern Africa is driven by the position of the tropical rain belt. Increasing north hemisphere insolation during northern hemisphere summer forces the tropical rain belt and ITCZ northward and the Congo Air Boundary (CAB) migrates eastward (Nakamura, Reference Nakamura1968; Hills, Reference Hills1979; Davies et al., Reference Davies, Vincent and Beresford1985). The eastward extent of CAB movement is related to the seasonal changes of Indian Summer Monsoon (ISM) strength (Camberlin, Reference Camberlin1997). Moreover, an increased W-E atmospheric pressure gradient between Africa and India during the northern hemisphere summer enhances the ISM (Wagner et al., Reference Wagner, Wennrich, Viehberg, Junginger, Kolvenbach, Rethemeyer, Schaebitz and Schmiedl2018). The enhanced ISM redirects Indian Ocean air masses towards India preventing them from penetrating deeply into eastern Africa. Therefore, the convergence zone of Indian Ocean air masses and Congo Basin air masses shifts to the east (Costa et al., Reference Costa, Russell, Konecky and Lamb2014).
In the past, in addition to the effects described above, an increased land-ocean temperature gradient caused strengthening of the West African Monsoon (WAM) and ISM. The interaction of monsoon intensity and eastward migration of the CAB may have been responsible for enhanced moisture in the region, thereby generating a water level rise in eastern African lakes (Junginger et al., Reference Junginger, Roller, Olaka and Trauth2014; Lezine et al., Reference Lezine, Bassinot and Peterschmitt2014). Additionally, increased lake levels may have been driven by a northward shift in the mean position of the tropical rain belt during the early Holocene northern hemisphere summer insolation maximum (Gasse, Reference Gasse2000). These processes led to a more pluvial early–mid Holocene (12–5 cal ka BP), termed the African Humid Period (AHP) (deMenocal et al., Reference deMenocal, Ortiz, Guilderson, Adkins, Sarnthein, Baker and Yarusinsky2000), which was particularly intense in North Africa and extended south to 10°S in eastern Africa (Gasse, Reference Gasse2000). While the general mechanisms for the orbitally forced AHP are well understood, the spatial and temporal patterns are intensively debated.
Due to the complexity of atmospheric circulation in eastern Africa, it is not surprising that the reconstructed timing of the AHP differs across sites. The termination of the AHP has been described as rapid (Collins et al., Reference Collins, Prange, Caley, Gimeno, Beckmann, Mulitza, Skonieczny, Roche and Schefuß2017), as synchronous and abrupt (Tierney and deMenocal, Reference Tierney and deMenocal2013), or asynchronous and gradual (Foerster et al., Reference Foerster, Junginger, Langkamp, Gebru, Asrat, Umer and Lamb2012; Costa et al., Reference Costa, Russell, Konecky and Lamb2014; van der Lubbe et al., Reference van der Lubbe, Krause-Nehring, Junginger, Garcin, Joordens, Davies, Beck, Feibel, Johnson and Vonhof2017). However, the inferred timing of the AHP termination may depend largely on the analyzed proxy (Castañeda et al., Reference Castañeda, Schouten, Pätzold, Lucassen, Kasemann, Kuhlmann and Schefuß2016) and the type of archive. Furthermore, in addition to a variable climate, the scarce and patchy paleoenvironmental records of Northern and eastern Africa supply insufficient data for comprehensive climate reconstruction. The hydrological history of eastern Africa has been reconstructed mainly from low-elevation sites.
High-altitude lakes have proved to be excellent sensors of environmental change (Catalan et al., Reference Catalan, Camarero, Felip, Pla, Ventura, Buchaca and Bartumeus2006, Reference Catalan, Pla-Rabés, Wolfe, Smol, Rühland, Anderson and Kopáček2013). They incorporate information about changes in the catchment, including varying erosion, often without significant anthropogenic disturbance or desiccation (Arnaud et al., Reference Arnaud, Poulenard, Giguet-Covex, Wilhelm, Révillon, Jenny and Revel2016). In eastern Africa, high-altitude paleolimnological research has been carried out at Lake Ashenge (2442 m asl; Marshall et al., Reference Marshall, Lamb, Davies, Leng, Bedaso, Umer and Bryant2009), Lake Dendi (2840 m asl; Wagner et al., Reference Wagner, Wennrich, Viehberg, Junginger, Kolvenbach, Rethemeyer, Schaebitz and Schmiedl2018), Sacred Lake (2350 m asl on Mount Kenya; Barker et al., Reference Barker, Street-Perrott, Leng, Greenwood, Swain, Perrott, Telford and Ficken2001, Reference Barker, Hurrell, Leng, Wolff, Cocquyt, Sloane and Verschuren2011; Street-Perrott et al., Reference Street-Perrott, Barker, Leng, Sloane, Wooller, Ficken and Swain2008; Loomis et al., Reference Loomis, Russell and Lamb2015, Reference Loomis, Russell, Verschuren, Morrill, De Cort, Sinninghe Damsté, Olago, Eggermont, Street-Perrott and Kelly2017), and Lake Garba Guracha (3950 m asl on the Bale Mountains; Umer et al., Reference Umer, Lamb, Bonnefille, Lézine, Tiercelin, Gibert, Cazet and Watrin2007; Tiercelin et al., Reference Tiercelin, Gibert, Umer, Bonnefille, Disnar, Lézine, Hureau-Mazaudier, Travi, Keravis and Lamb2008; Bittner et al., Reference Bittner, Bliedtner, Grady, Gil-Romera, Martin-Jones, Lemma and Mekonnen2020).
Paleolimnological research has advanced in recent decades by the use of stable isotope ratios as environmental indicators. The oxygen isotope composition (δ18O) of biogenic compounds and autochthonous carbonates has been established as a valuable paleoclimate proxy because the value of δ18O in water, which is incorporated into biogenic and autochthonous compounds, depends on fractionation processes linked to temperature, air mass source and trajectory, and global ice volume.
Oxygen isotope composition has been determined for several Quaternary records in eastern Africa: for example, δ18Oice cores (Thompson et al., Reference Thompson, Mosley-Thompson, Davis, Henderson, Brecher, Zagorodnov and Mashiotta2002), δ18Ocarbonate (Lamb et al., Reference Lamb, Leng, Sloane and Telford2005), δ18Odiatom (Barker et al., Reference Barker, Street-Perrott, Leng, Greenwood, Swain, Perrott, Telford and Ficken2001, Reference Barker, Leng, Gasse and Huang2007, Reference Barker, Hurrell, Leng, Wolff, Cocquyt, Sloane and Verschuren2011; Lamb et al., Reference Lamb, Leng, Sloane and Telford2005), and δ18Osugar (Hepp et al., Reference Hepp, Zech, Rozanski, Tuthorn, Glaser, Greule, Keppler, Huang, Zech and Zech2017). δ18Osugar has been successfully applied to several other paleolimnological archives (Zech et al., Reference Zech, Tuthorn, Zech, Schlütz, Zech and Glaser2014b; Hepp et al., Reference Hepp, Tuthorn, Zech, Mügler, Schlütz, Zech and Zech2015, Reference Hepp, Glaser, Juchelka, Mayr, Rozanski, Schäfer, Stichler, Tuthorn, Zech and Zech2019). In contrast to this relatively new proxy (Zech and Glaser, Reference Zech and Glaser2009), numerous δ18Odiatom records have been published, proving the potential for reconstructing past climate changes from biogenic silica (δ18Odiatom) (Leng et al., Reference Leng, Barnker, Greenwood, Roberts and Reed2001; Barker et al., Reference Barker, Talbot, Street-Perrott, Marret, Scourse, Odada, Battarbee, Gasse and Stickley2004, Reference Barker, Leng, Gasse and Huang2007, Reference Barker, Hurrell, Leng, Wolff, Cocquyt, Sloane and Verschuren2011; Lamb et al., Reference Lamb, Leng, Sloane and Telford2005; Wilson et al., Reference Wilson, Maslin, Leng, Kingston, Deino, Edgar and Mackay2014; Narancic et al., Reference Narancic, Pienitz, Chapligin, Meyer, Francus and Guilbault2016; Cartier et al., Reference Cartier, Sylvestre, Paillès, Sonzogni, Couapel, Alexandre, Mazur, Brisset, Miramont and Guiter2019; Kostrova et al., Reference Kostrova, Meyer, Bailey, Ludikova, Gromig, Kuhn, Shibaev, Kozachek, Ekaykin and Chapligin2019).
At Garba Guracha, early work by Umer et al. (Reference Umer, Lamb, Bonnefille, Lézine, Tiercelin, Gibert, Cazet and Watrin2007) and Tiercelin et al. (Reference Tiercelin, Gibert, Umer, Bonnefille, Disnar, Lézine, Hureau-Mazaudier, Travi, Keravis and Lamb2008) focused on sedimentological, geochemical, and pollen analyses. Their work indicates that Garba Guracha is one of the longest, most continuous high-resolution Late Quaternary environmental archives from highland Africa. With a new core, Bittner et al. (Reference Bittner, Bliedtner, Grady, Gil-Romera, Martin-Jones, Lemma and Mekonnen2020) established a higher resolution chronology and analyzed XRF and biomarkers. Gil-Romera et al. (Reference Gil-Romera, Adolf, Benito Blas, Bittner, Johansson, Grady and Lamb2019) carried out charcoal and pollen analyses on the same core to show that fire has been a constant disturbance at millennial time scales in the Afromontane vegetation of the Bale Mountains, driving the long-term ecological dynamics of Erica spp.
Here we present the first record in eastern Africa of lake-level variation derived from the oxygen isotope composition of sugar biomarkers and diatoms and the first high-altitude, long-term oxygen isotope record from above 3500 m asl in Ethiopia. We compare the results of well-established δ18Odiatom analyses with the more novel δ18Ofuc analyses (Zech and Glaser, Reference Zech and Glaser2009) to gain more detailed knowledge regarding the δ18Ofuc proxy and interpretations of the proxy from the archive itself. Our main aims are: (1) to determine the allochthonous versus autochthonous source of the analyzed sugar biomarkers, (2) to use δ18Odiatom analyses to test and corroborate the δ18Ofuc results, (3) to describe the hydrological history of high-altitude Garba Guracha, and (4) to consider the implications of our data for understanding regional paleoclimate.
REGIONAL SETTING
Study area
Garba Guracha is situated in the Bale Mountains of the Bale-Arsi Massif, located east of the Main Ethiopian Rift (Fig. 1). The Sanetti Plateau is the highest plateau in the Bale Mountains at an altitude between ~3800 and ~4200 m asl, encompassing an area of 600 km2, bordered by a steep escarpment to the south (Osmaston et al., Reference Osmaston, Mitchell and Osmaston2005). Deep valleys incised by northward descending rivers characterize the northern slopes. The volcanic plateau is formed of solidified horizontal lava consisting of alkali basalt, trachyte, and tuffs with rhyolites, overlying older volcanic material (Uhlig and Uhlig, Reference Uhlig and Uhlig1991; Williams, Reference Williams and Williams2016). During the Last Glacial Maximum, the plateau and the valleys were locally glaciated (Osmaston et al., Reference Osmaston, Mitchell and Osmaston2005; Ossendorf et al., Reference Ossendorf, Groos, Bromm, Girma Tekelemariam, Glaser, Lesur and Schmidt2019; Groos et al., Reference Groos, Niederhauser, Wraase, Hänsel, Nauss, Akçar and Veit2020, Reference Groos, Akçar, Yesilyurt, Miehe, Vockenhuber and Veit2021). The glacial cirque Garba Guracha (6.875781°N, 39.878075°E) (Fig. 2b) was first mentioned by Werdecker (Reference Werdecker1962) and was described in detail by Umer et al. (Reference Umer, Lamb, Bonnefille, Lézine, Tiercelin, Gibert, Cazet and Watrin2007) and Tiercelin et al. (Reference Tiercelin, Gibert, Umer, Bonnefille, Disnar, Lézine, Hureau-Mazaudier, Travi, Keravis and Lamb2008). It is located at 3950 m asl, has a maximum water depth of 6 m, a very small watershed (0.15 km2), and extends to ~500 x 300 m in area (Fig. 2c). The catchment bedrock is carbonate-poor (Löffler, Reference Löffler1978; Uhlig and Uhlig, Reference Uhlig and Uhlig1991). The lake has an outlet during the rainy season. A marshy alluvial plain fed by several springs extends to the south of the lake.

Figure 1. Overview of the region: (1) Dongge caves, (2) Qunf cave, (3) Lake Abhè, (4) Ziway-Shala, (5) Garba Guracha (this study), (6) Chew Bahir, (7) Lake Turkana, (8) Paleolake Suguta.
Climate
The climate of the Bale Mountains differs from north to south due to differences in altitude, aspect, and continental air masses (Uhlig and Uhlig, Reference Uhlig and Uhlig1991; Kidane et al., Reference Kidane, Stahlmann and Beierkuhnlein2012). The mean annual temperature at Dinsho (Fig. 2a A) is 11.8°C, and the mean minimum temperature for the coldest month is 0.6°C (Hillman, Reference Hillman1986). Ten newly installed climate stations across the Bale Mountains have provided climate data since 2017. The results from 2017 show a mean annual temperature of 4.9°C at the Angesso Station (Fig. 2a B), which is located 4 km northeast of Garba Guracha.
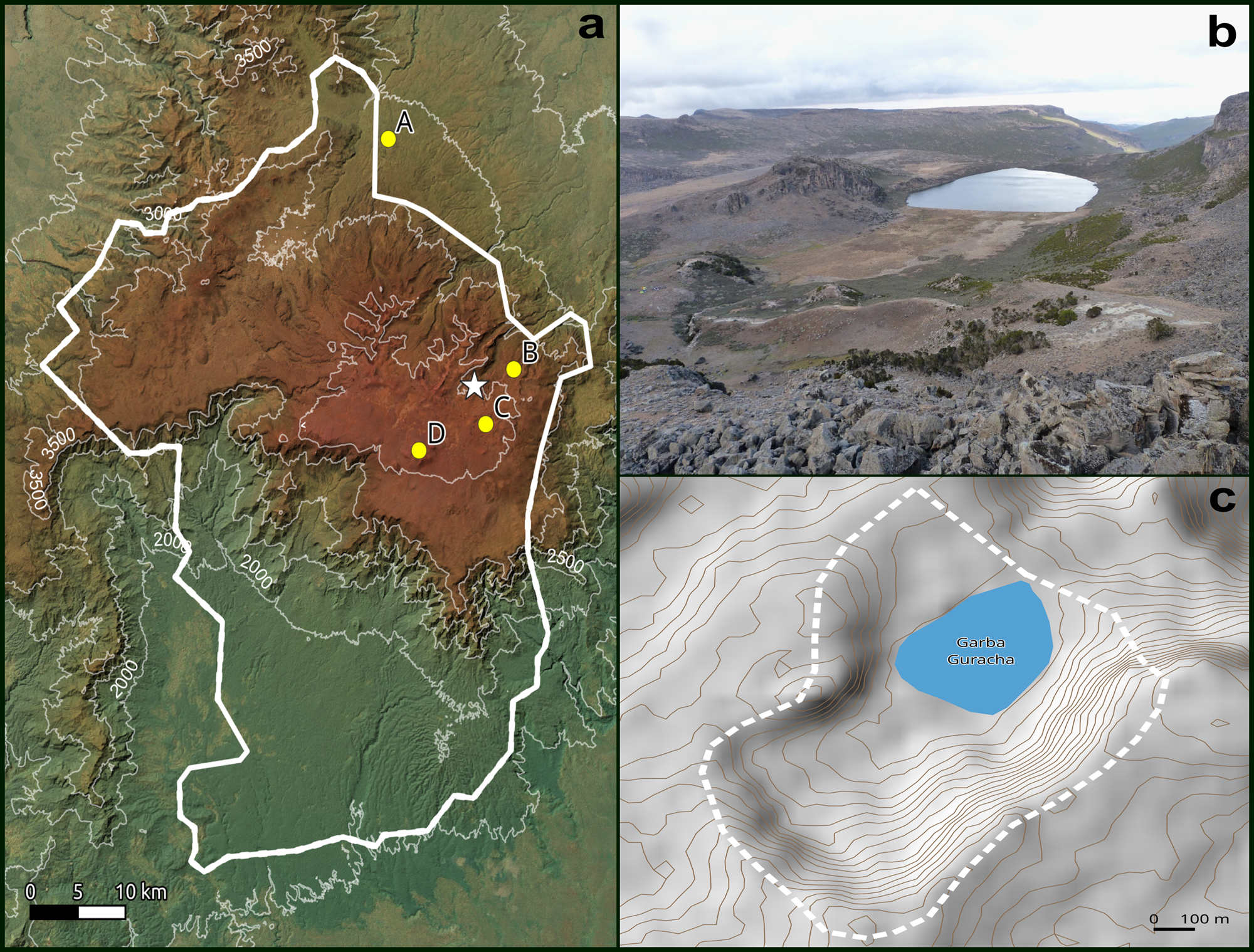
Figure 2. Location of the study area. (a) Bale Mountain National Park (thick white line); climate stations: (A) Dinsho, (B) Angesso station, (C) EWCP station, (D) Tulu Dimtu; (b) northeastward view over the glacial cirque of the Garba Guracha catchment; (c) Garba Guracha catchment structure and watershed (dashed white line).
Precipitation in the Bale Mountains originates from two moisture sources, the Indian Ocean monsoon, and the Equatorial Westerlies (Uhlig, Reference Uhlig1988; Miehe and Miehe, Reference Miehe and Miehe1994). A dry season from November to February and a bimodal wet season from March to October define the climate. Precipitation maxima occur in April/May and September/October, respectively (Woldu et al., Reference Woldu, Feoli and Nigatu1989; Lemma et al., Reference Lemma, Kebede Gurmessa, Nemomissa, Otte, Glaser and Zech2020). The highest monthly rainfalls (July to September) are related to the convergence of southwest air masses. In 2017, Angesso Station (3949 m asl) (Fig. 2a B) recorded 1097 mm while 711 mm were registered at the EWCP Station (elevation 4124 m) (Fig. 2a C) and 468 mm at Tulu Dimtu (4385 m asl) (Fig. 2a D). Garba Guracha (3950 m asl) lies at a similar altitude to the Angesso Station. The afro-alpine regions, including the Sanetti Plateau, are characterized by strong diurnal temperature differences between day and night (-15°C to +26°C) (Hillman, Reference Hillman1988).
MATERIAL AND METHODS
Material and sampling
In February 2017, we retrieved two overlapping sediment cores using a Livingstone piston corer from a raft anchored at 4.8 m water depth. A maximum sediment depth of 1550 cm was reached with an organic-rich upper part (0–900 cm) (Fig. 3) and an organic-poor lower part (900–1550 cm). For radiocarbon dating, we took a total of 31 samples, comprising 18 bulk sediment samples, 8 bulk n-alkane, and 5 charcoal samples. More details on sediment and chronology can be found in Bittner et al. (Reference Bittner, Bliedtner, Grady, Gil-Romera, Martin-Jones, Lemma and Mekonnen2020). For sugar biomarker analyses (n = 80), the organic-rich upper core sections (top 900 cm, compressed to ~800 cm during coring) were sampled at contiguous 10 cm intervals, each sample representing ca. 103 years of sedimentation. This sampling technique enabled us to create a continuous record where variability is smoothed, but without missing information. Nineteen of those samples were later selected for diatom analyses.

Figure 3. Depth profiles of age and TOC (Bittner et al., Reference Bittner, Bliedtner, Grady, Gil-Romera, Martin-Jones, Lemma and Mekonnen2020), δ18Ofuc (blue), and δ18Odiatom (purple) (this study). The black line shows the running mean of 5. Stacked core photos are shown on the left and shaded areas indicate the standard error range.
Compound-specific δ18O analyses of sugar biomarkers
Sugar biomarker extraction was done at the Institute of Agricultural and Nutritional Sciences, Soil Biogeochemistry, Martin-Luther-University Halle-Wittenberg following the method described by Zech and Glaser (Reference Zech and Glaser2009). Briefly, monosaccharides were extracted from the homogenized samples by hydrolysis using 4 M trifluoroacetic acid at 105°C for 4 hours (Amelung et al., Reference Amelung, Cheshire and Guggenberger1996). The dissolved monosaccharides were first filtered over glass fiber filters, evaporated, and then further cleaned by transferring them with H2O onto XAD-7 columns and finally over DOWEX 50WX8 columns. After freeze-drying, the samples were derivatized with methylboronic acid (MBA) for 1 hour at 60°C (Knapp, Reference Knapp1979). The derivatized samples were measured in triplicates on a Trace GC 2000 coupled to a Delta V Advantage IRMS via an 18O-pyrolysis reactor (GC IsoLink) and a ConFlow IV interface (all devices from Thermo Fisher Scientific, Bremen, Germany). Sugar standards with known δ18O values, containing arabinose (ara), fucose (fuc), and xylose (xyl), were measured in various concentrations after every six sample triplicates (Zech and Glaser, Reference Zech and Glaser2009). Correction of the sample δ18O values was applied for possible amount dependency and drift during a sample batch and for the hydrolytically exchangeable oxygen atoms of the carbonyl group (Zech and Glaser, Reference Zech and Glaser2009). The δ18O values of the monosaccharides are presented in the usual δ-notation versus the Vienna Standard Mean Ocean Water (VSMOW).
δ18O analyses of diatoms
Nineteen sediment samples were processed for diatom oxygen isotope (δ18Odiatom) analyses. Two g of dry sediment were used to purify samples using a multi-step cleaning procedure. Sediment samples were first treated with 35% H2O2 on a heating plate at 50°C for 72 hours to remove organic matter, adding 10% HCl at 50°C to eliminate carbonates, then washed pH neutral. The subsequent heavy liquid separation was carried out with sodium polytungstate (SPT; 3Na₂WO₄⋅9WO₃⋅H₂O) heavy liquid solutions with decreasing densities (2.50−2.05 g/ml) and subsequent centrifuging at 2500 rpm for 30 minutes, leading to the separation of diatoms from heavier detrital contaminants. Decreasing densities were subsequently used to properly separate diatoms from the mineral particles of the terrigenous fraction. Different diatom species have slightly different densities, which makes this step-wise separation procedure necessary in order not to lose any diatoms during heavy liquid separation. This detritus was retained for contamination assessment and used for δ18Odiatom correction following Chapligin et al. (Reference Chapligin, Meyer, Friedrichsen, Marent, Sohns and Hubberten2010). Diatom samples were washed in ultra-pure water at 2500 rpm for 20 minutes and sieved through a 3 μm filter. Sixteen of the purified diatom samples (n = 19) yielded enough material (>1.5 mg) to be measured for δ18O at the AWI Potsdam ISOLAB Facility.
To remove exchangeable oxygen, inert Gas Flow Dehydration (iGFD) and heating to 1100°C under Argon gas (following Chapligin et al., Reference Chapligin, Meyer, Friedrichsen, Marent, Sohns and Hubberten2010) was applied. Dehydrated samples were then quantitatively reacted to liberate O2 by laser fluorination under BrF5 atmosphere (Clayton and Mayeda, Reference Clayton and Mayeda1963). Sample oxygen was directly measured against a calibrated oxygen reference with a PDZ Europa 2020 mass spectrometer. The working standard BFC (δ18O = 29.0 ± 0.3‰; Chapligin et al., Reference Chapligin, Leng, Webb, Alexandre, Dodd, Ijiri and Lücke2011) was used for calibration (this study: δ18O = +28.88 ± 0.24‰; n = 10) for controlling both accuracy and precision of the isotope analyses. The long-term analytical reproducibility (1σ) is ± 0.25‰ (Chapligin et al., Reference Chapligin, Meyer, Friedrichsen, Marent, Sohns and Hubberten2010). All measured diatom δ18O values were corrected for contamination, mainly due to clay particles (Supplementary Material Fig. 7), using a geochemical mass-balance approach (Chapligin et al., Reference Chapligin, Meyer, Bryan, Snyder and Kemnitz2012). Contamination was calculated for all samples individually and corrected following the method described in Chapligin et al. (Reference Chapligin, Meyer, Bryan, Snyder and Kemnitz2012). Briefly, we calculated the correction following Equation 2 in Chapligin et al. (Reference Chapligin, Meyer, Bryan, Snyder and Kemnitz2012):

where δ18Omeasured is the isotopic composition of the sample after purification, %cont. is the contamination percentage left in the purified sample and δ18Ocont. is the isotopic composition of the contaminants (i.e., the heavy [mineral] fraction).
RESULTS
δ18Ofuc record of Garba Guracha
Compound-specific δ18O values for the sugar biomarker fucose reveal a total range from +24.7 to +36.2‰ (Fig. 3). The mean standard error for fucose was ± 1.4‰. The δ18O values of fucose increase from 840 cm (11 cal ka BP) and begin to decrease at ~800 cm (10.5 cal ka BP) showing minima between 665 and 535 cm (9 and 7 cal ka BP). Thereafter, a continuous trend towards more positive δ18O values of fucose is apparent until 290 cm (3.5 cal ka BP). Above this depth, stable values continue with the exception of one pronounced minimum at ~85 cm (0.5 cal ka BP).
In detail, the Early Holocene reveals centennial-scale δ18Ofuc shifts with minima at 840 and 750 cm (10.9 and 10.2 cal ka BP), and a maximum at 800 cm (10.6 cal ka BP). The phase of lowest δ18Ofuc values is interrupted by a short maximum at 635 cm (8.4 cal ka BP). The Late Holocene reveals major fluctuations of δ18Ofuc between 425 and 370 cm (5.5 and 4.6 cal ka BP). Moreover, a maximum at 290 cm (3.5 cal ka BP), a minimum at 85 cm (0.5 cal ka BP), and numerous centennial-scale variations are visible in the Late Holocene. However, the variability of δ18O is highest during the Early Holocene and decreases towards the Late Holocene.
δ18Odiatom record of Garba Guracha
For most of the 16 diatom samples, duplicate or triplicate oxygen isotope analyses yielded a mean (max) standard deviation of ±0.26‰ (±0.51‰: Supplemental Table 1). One sample could only be measured once due to low diatom content after the separation procedure. In general, the δ18Odiatom values span a range of 7.9‰ (Fig. 3). Like the δ18Ofuc data, the δ18Odiatom values decrease above 750 cm (10 cal ka BP), with lowest values co-occurring between 665 and 535 cm (9 and 7 cal ka BP) (δ18Odiatom ~+37‰). An overall increasing trend between 535 and 285 cm (7 and 3.5 cal ka BP) is interrupted by a decrease between 425 and 370 cm (5.5 and 4.6 cal ka BP). More less-positive values follow the maximum at 285 cm (3.5 cal ka BP) (δ18Odiatom = +44.8‰) until 140 cm. A small maximum at 140 cm (1.5 cal ka BP) and subsequent decreasing values are similar to the δ18Ofuc record.
DISCUSSION
The Garba Guracha δ18Ofuc record - lake or leaf water?
A crucial issue for the interpretation of δ18Ofuc records is the aquatic (autochthonous) or terrestrial (allochthonous) origin of the sedimentary sugar biomarkers. Plant-derived sugar biomarkers, modified by a biosynthetic fractionation factor (ɛbio), can either reflect lake water δ18O (δ18Olake water) or terrestrial leaf water δ18O (δ18Oleaf water) origin. In the case of Garba Guracha, a high aquatic organic matter content of the sediments can be inferred from several proxies. First, relatively positive δ13C values of > -23‰ and a low TOC/N ratio of <15 indicate a high aquatic organic matter content (Bittner et al., Reference Bittner, Bliedtner, Grady, Gil-Romera, Martin-Jones, Lemma and Mekonnen2020). Second, the sugar biomarker quantification pattern indicates a high relative abundance of fucose in the Garba Guracha sediments. Fucose is a major component of phytoplankton, zooplankton, and bacteria (Ogier et al., Reference Ogier, Disnar, Albéric and Bourdier2001), as well as of aquatic plants (Hepp et al., Reference Hepp, Rabus, Anhäuser, Bromm, Laforsch, Sirocko, Glaser and Zech2016). In terrestrial vascular plants, fucose is produced in low concentrations, according to Hepp et al. (Reference Hepp, Rabus, Anhäuser, Bromm, Laforsch, Sirocko, Glaser and Zech2016) who developed two ratios, fuc/(ara + xyl) and (fuc + xyl)/ara, to distinguish between aquatic (> 0.10) and terrestrial (≤ 0.10) input. In our data, both ratios suggest that the sediments of Garba Guracha contain sugar biomarkers that are principally of aquatic origin (Bittner et al., Reference Bittner, Bliedtner, Grady, Gil-Romera, Martin-Jones, Lemma and Mekonnen2020, Fig. 6). While a partial terrestrial contribution of sugar biomarkers to the sediments cannot be fully excluded, the terrestrial contribution, especially of fucose, can be neglected. We therefore interpret our δ18Ofuc data as a record of changes in δ18Olake water.
The Garba Guracha δ18Odiatom record
δ18Olake water and temperature at the time of frustule formation define the δ18Odiatom composition of aquatic diatoms (Labeyrie, Reference Labeyrie1974; Leclerc and Labeyrie, Reference Leclerc and Labeyrie1987; Leng and Barker, Reference Leng and Barker2006). Some processes in the incorporation of oxygen isotopes into diatom silica (summarized by Bird et al., Reference Bird, Haig, Hadeen, Rivera-Araya, Wurster and Zwart2020) still require a better understanding, such as (1) species-specific fractionation (Bailey et al., Reference Bailey, Henderson, Sloane, Snelling, Leng and Kaufman2014), (2) post-mortem alteration of the oxygen isotopic composition (Tyler et al., Reference Tyler, Sloane, Rickaby, Cox and Leng2017), and (3) the effect of diagenesis on oxygen isotope fractionation and exchange (Dodd et al., Reference Dodd, Sharp, Fawcett, Brearley and McCubbin2012). However, δ18Odiatom analyses have been applied successfully to many archives as a proxy for P/E to identify wet and dry conditions (Polissar et al., Reference Polissar, Abbott, Shemesh, Wolfe and Bradley2006; Meyer et al., Reference Meyer, Chapligin, Hoff, Nazarova and Diekmann2015), moisture source (Rosqvist et al., Reference Rosqvist, Jonsson, Yam, Karlén and Shemesh2004; Leng et al., Reference Leng, Metcalfe and Davies2005; Schiff et al., Reference Schiff, Kaufman, Wolfe, Dodd and Sharp2009), δ18Oprecipitation (Morley et al., Reference Morley, Leng, Mackay and Sloane2005; Mackay et al., Reference Mackay, Swann, Fagel, Fietz, Leng, Morley, Rioual and Tarasov2013; Bailey et al., Reference Bailey, Kaufman, Henderson and Leng2015), hydrological changes (Narancic et al., Reference Narancic, Pienitz, Chapligin, Meyer, Francus and Guilbault2016; Kostrova et al., Reference Kostrova, Meyer, Bailey, Ludikova, Gromig, Kuhn, Shibaev, Kozachek, Ekaykin and Chapligin2019), and temperature (Kostrova et al., Reference Kostrova, Meyer, Chapligin, Tarasov and Bezrukova2014). The interpretation of δ18Odiatom as a proxy for temperature is difficult due to the variability of δ18Oprecipitation and, especially in warm and/or dry environments, due to evaporative enrichment. These factors may have a more significant influence on δ18Odiatom than temperature (Leng and Barker, Reference Leng and Barker2006). Especially in the tropics, where inter-annual and seasonal temperature variability is low, δ18Odiatom generally has been interpreted as a proxy for precipitation amount (Cole et al., Reference Cole, Rind, Webb, Jouzel and Healy1999; Lamb et al., Reference Lamb, Leng, Sloane and Telford2005; Barker et al., Reference Barker, Leng, Gasse and Huang2007). Barker et al. (Reference Barker, Street-Perrott, Leng, Greenwood, Swain, Perrott, Telford and Ficken2001) suggested that the δ18Odiatom record of Small Hall Tarn and Simba Tarn at Mount Kenya represents the moisture balance of lake-level stands and overflows.
For reconstructing the δ18Olake water, several calibration studies for modern diatoms (Brandriss et al., Reference Brandriss, O'Neil, Edlund and Stoermer1998; Moschen et al., Reference Moschen, Lücke and Schleser2005; Crespin et al., Reference Crespin, Sylvestre, Alexandre, Sonzogni, Paillès and Perga2010; Dodd and Sharp, Reference Dodd and Sharp2010) and one for sedimentary diatoms (Leclerc and Labeyrie, Reference Leclerc and Labeyrie1987) have been published. The temperature-coefficient of these studies is quite similar (-0.16‰/°C to -0.29‰/°C), although offsets of several per mil between the regression lines are present. Explanations suggested by Crespin et al. (Reference Crespin, Sylvestre, Alexandre, Sonzogni, Paillès and Perga2010) include (1) an under- or overestimation of temperature or δ18Olake water in the calibration, (2) incomplete accounting for exchangeable oxygen, and (3) post-mortem 18O enrichment of sedimentary diatoms (Schmidt et al., Reference Schmidt, Botz, Rickert, Bohrmann, Hall and Mann2001). To account for post-mortem 18O enrichment, we reconstructed the δ18Olake water of the Garba Guracha sedimentary diatoms using the equation of Leclerc and Labeyrie (Reference Leclerc and Labeyrie1987). Taking into consideration a mean annual Tair of ~4.9°C (min: 3.5°C, max: 6.0°C) in 2017, measured at the closest meteorological station (Angesso), which is at the same altitude as Lake Garba Guracha, an isotope fractionation factor α in the system (silica-water) of 1.0435 ± 0.004 can be calculated (Leclerc and Labeyrie, Reference Leclerc and Labeyrie1987). The calculated value is subject to some uncertainty due to the lack of more recent δ18Odiatom values, an incomplete record of modern δ18Olake water variability, and an underestimation of frustule growth temperature by using mean annual air temperature.
Based on these factors, the measured most recent (931 cal. BP) δ18Odiatom of ~ +43.4‰ at Lake Garba Guracha leads to a calculated “modern” δ18Olake water value of +0.8 ± 0.3‰, which is lower than the measured δ18Olake water of +4.7‰ at the end of the 2017 dry season, representing strong 18O enrichment of lake water (Lemma et al., Reference Lemma, Kebede Gurmessa, Nemomissa, Otte, Glaser and Zech2020). For comparison, precipitation in 2017 yielded a mean δ18O value of -4.5‰ (max -6.2‰, min -1.9‰). The reconstructed δ18Olake water value of +0.8‰ for the uppermost diatom sample is hence a reasonable estimated value between those of modern precipitation (-4.5‰) and the seasonally enriched lake water (+4.7‰) (Lemma et al., Reference Lemma, Kebede Gurmessa, Nemomissa, Otte, Glaser and Zech2020).
Comparison of reconstructed δ18Olake water from δ18Ofuc versus δ18Odiatom
The predominantly aquatic origin of sugar biomarkers in the Garba Guracha sediments, as discussed earlier, leads to the conclusion that the isotopic composition of the fucose sugar biomarkers primarily represents δ18Olake water modified by εbio, the biosynthetic fractionation factor. εbio can be estimated as +29‰ between aquatic cellulose δ18O and δ18Olake water (Mayr et al., Reference Mayr, Laprida, Lücke, Martín, Massaferro, Ramón-Mercau and Wissel2015). An εbio of +29‰ may slightly underestimate the fractionation factor of aquatic hemicellulose, as is known for terrestrial cellulose and hemicellulose (Zech et al., Reference Zech, Mayr, Tuthorn, Leiber, Glaser, Leiber-Sauheitl and Glaser2014a, Reference Zech, Tuthorn, Zech, Schlütz, Zech and Glaserb; Hepp et al., Reference Hepp, Glaser, Juchelka, Mayr, Rozanski, Schäfer, Stichler, Tuthorn, Zech and Zech2019). The slight offset derives from the loss of the relatively 18O-depleted oxygen atom attached to C-6 during 6-deoxyhexose (fucose) biosynthesis (C-6 decarboxylation; Altermatt and Neish, Reference Altermatt and Neish1956; Harper and Bar-Peled, Reference Harper and Bar-Peled2002; Burget et al., Reference Burget, Verma, Mølhøj and Reiter2003). This is supported by the findings of Waterhouse et al. (Reference Waterhouse, Cheng, Juchelka, Loader, Mccarroll, Switsur and Gautam2013) that ~80% of the oxygen atoms at the C-6 position are isotopically exchanged during cellulose synthesis. Similarly, the Garba Guracha δ18Odiatom record can be interpreted as δ18Olake water due to a lesser influence of temperature in tropical regions, as discussed previously.
The reconstructed δ18Olake water values of sugar biomarker and diatoms are consistent after correction for εbio (+29‰) (Mayr et al., Reference Mayr, Laprida, Lücke, Martín, Massaferro, Ramón-Mercau and Wissel2015) and temperature-dependent fractionation during diatom growth (Leclerc and Labeyrie, Reference Leclerc and Labeyrie1987), respectively (Fig. 4). Additionally, the δ18Odiatom and δ18Ofuc ranges of the 16 analyzed samples are similar (7.9‰ and 7.1‰, respectively). Despite the rather small number of analyses, the aquatic δ18Odiatom and δ18Ofuc show identical trends during the Holocene (Fig. 3), pointing to a common source of δ18Ofuc and δ18Odiatom. Therefore, we conclude that the δ18Odiatom record corroborates the δ18Ofuc record to reflect primarily δ18Olake water and, moreover, that δ18Ofuc of aquatic sugar biomarker is a valuable proxy for δ18Olake water in the Garba Guracha sedimentary archive.

Figure 4. Comparison of the δ18Odiatom and δ18Ofucose records of Garba Guracha. The black line shows the running mean of 5 and shaded areas the standard error range.
Paleoclimatic significance and proxy interpretation
Past variations of δ18Olake water reflect past changes in δ18Oprecipitation and evaporative 18O enrichment of lake water. In the tropics, δ18Oprecipitation values in paleo-archives and rainfall records have been interpreted to represent changes in the amount of precipitation—depleted δ18O values indicating high amounts of precipitation (amount effect; Dansgaard, Reference Dansgaard1964; Rozanski et al., Reference Rozanski, Araguás-Araguás, Gonfiantini, Swart, Lohmann, Mckenzie and Savin1993). However, besides amount effect, the history of the entire moist air mass, including source region (source effect), the air mass convection (altitude effect), (re)evaporative history (recycling), and transport distance (continental effect), must be considered (Sharp, Reference Sharp2017). Moreover, δ18Oprecipitation is influenced by temperature (temperature effect; Rozanski et al., Reference Rozanski, Araguás-Araguás, Gonfiantini, Swart, Lohmann, Mckenzie and Savin1993). However, temperature changes in the tropics, especially during the Holocene, were rather small (Berke et al., Reference Berke, Johnson, Werne, Schouten and Sinninghe Damsté2012), implying that the temperature effect is negligible (Rozanski et al., Reference Rozanski, Araguás-Araguás, Gonfiantini, Swart, Lohmann, Mckenzie and Savin1993; Vuille et al., Reference Vuille, Werner, Bradley and Keimig2005).
The relatively small temperature effect means that δ18Oprecipitation is predominantly influenced by the amount and the source effect in eastern Africa. For the tropics in general, high-resolution δ18O speleothem records, such as those of Fleitmann et al. (Reference Fleitmann, Burns, Neff, Mangini and Matter2003) and Dykoski et al. (Reference Dykoski, Edwards, Cheng, Yuan, Cai, Zhang, Lin, Qing, An and Revenaugh2005) (Fig. 5), are generally interpreted as mainly reflecting variations in the intensity of the monsoon systems (amount effect)—East Asian Summer (EASM) and the Indian Summer Monsoon (ISM). Overall trends of Qunf cave δ18O (Fleitmann et al., Reference Fleitmann, Burns, Neff, Mangini and Matter2003) and Dongge cave δ18O (Dykoski et al., Reference Dykoski, Edwards, Cheng, Yuan, Cai, Zhang, Lin, Qing, An and Revenaugh2005) are in agreement with our δ18O records (Fig. 5). Monsoon intensity in eastern Africa may have played an essential role in controlling the stable isotope composition of precipitation, as recorded in our Bale Mountain archive and reconstructed δ18O values. However, modern data suggest that the amount effect alone does not explain the δ18Oprecipitation pattern in eastern Africa (Costa et al., Reference Costa, Russell, Konecky and Lamb2014; Lemma et al., Reference Lemma, Kebede Gurmessa, Nemomissa, Otte, Glaser and Zech2020). Therefore, Costa et al. (Reference Costa, Russell, Konecky and Lamb2014) questioned the primary influence of the amount effect for Lake Tana, Ethiopia, and suggested that an Atlantic moisture source (Congo Basin) was responsible for both past and current depleted δ18Oprecipitation values. Back-trajectories for modern precipitation at Lake Tana show an average contribution from Congo Basin air masses of 13%. The primary source is the Indian Ocean (80%), with only a minor contribution of 7% from the Mediterranean and Red Sea (Costa et al., Reference Costa, Russell, Konecky and Lamb2014). Other climate studies for Ethiopia have found only minor (Viste and Sorteberg, Reference Viste and Sorteberg2013) or no (Levin et al., Reference Levin, Zipser and Ceding2009) air mass contributions to southern Ethiopia from the Congo Basin. However, a recent study by Finney et al. (Reference Finney, Marsham, Walker, Birch, Woodhams, Jackson and Hardy2020) suggests that despite the low air mass contribution, westerly winds are responsible for a disproportionally high amount of rainfall in eastern Africa. For the Bale Mountains, a recent back-trajectory study found air mass contributions principally from the Indian Ocean and the Mediterranean and Red Sea, but no evidence for any contribution or even an isotopic depletion due to Congo Basin air masses (Lemma et al., Reference Lemma, Kebede Gurmessa, Nemomissa, Otte, Glaser and Zech2020).
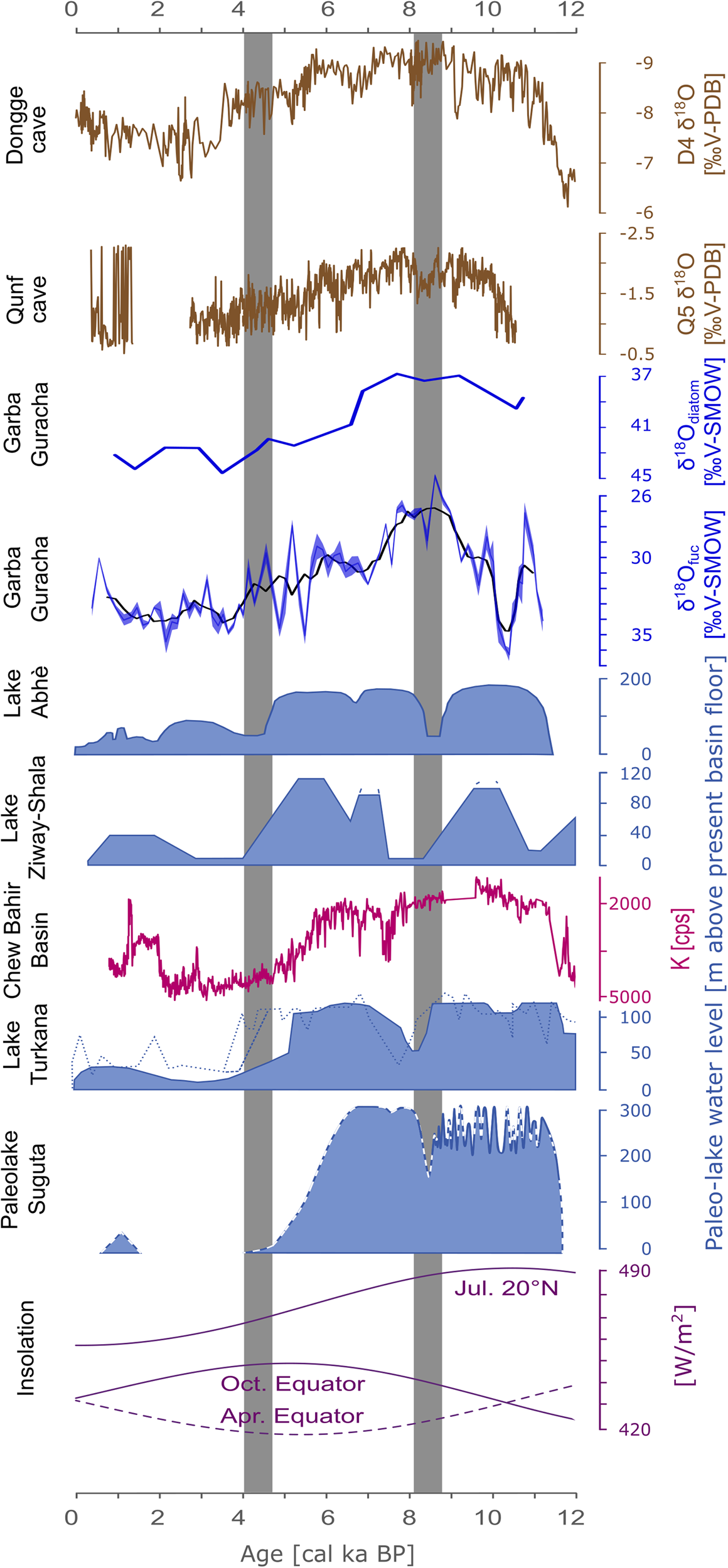
Figure 5. Comparison of lake level reconstructions in eastern Africa and δ18O records for the past 12,000 years (adapted from Junginger et al., Reference Junginger, Roller, Olaka and Trauth2014). Dongge cave (Dykoski et al., Reference Dykoski, Edwards, Cheng, Yuan, Cai, Zhang, Lin, Qing, An and Revenaugh2005), Qunf cave (Fleitmann et al., Reference Fleitmann, Burns, Neff, Mangini and Matter2003), Garba Guracha (this study), Lake Abhè (Gasse, Reference Gasse2000), Lake Ziway-Shala (Gillespie et al., Reference Gillespie, Street-Perrott and Switsur1983), Chew Bahir Basin (Foerster et al., Reference Foerster, Junginger, Langkamp, Gebru, Asrat, Umer and Lamb2012), Lake Turkana (Garcin et al., Reference Garcin, Melnick, Strecker, Olago and Tiercelin2012; filled curve), (Johnson et al., Reference Johnson, Halfman and Showers1991; dotted curve), (Brown and Fuller, Reference Brown and Fuller2008; dashed curve), Paleolake Suguta (Junginger et al., Reference Junginger, Roller, Olaka and Trauth2014), and insolation variations (Laskar et al., Reference Laskar, Robutel, Joutel, Gastineau, Correia and Levrard2004).
Even if these air mass contributions are not nowadays influencing rainfall in southeastern Ethiopia (Levin et al., Reference Levin, Zipser and Ceding2009) or the Bale Mountains (Lemma et al., Reference Lemma, Kebede Gurmessa, Nemomissa, Otte, Glaser and Zech2020), a past change of atmospheric circulation could have exerted a significant effect on the isotopic composition of paleo records (Tierney et al., Reference Tierney, Lewis, Cook, LeGrande and Schmidt2011a; Junginger et al., Reference Junginger, Roller, Olaka and Trauth2014). We, however, have no further evidence to test potential past source contributions at our study site. A change in moisture source might explain some δ18O variations in the Garba Guracha archive. However, because our 18O records are of aquatic origin, unlike other records, we reconstructed δ18Olake water, not δ18Oprecipitation, and suggest an additional strong influence of evaporative enrichment in the Garba Guracha record.
In open lake systems, δ18Oprecipitation is the most crucial control on δ18Olake water, whereas in closed lake systems, evaporative enrichment is the primary driver (Talbot, Reference Talbot1990; Lamb et al., Reference Lamb, Kebede, Leng, Ricketts, Telford, Umer, Odada and Olago2002; Horton et al., Reference Horton, Defliese, Tripati and Oze2016). Garba Guracha in modern times, with a sill level at 6 m above the sediment surface at the lake's maximum depth, is an open lake during the wet season and a closed lake system during the dry season (Supplementary Material Fig. 6). Lake water samples taken during the dry season (+ 4.7‰, ± 0.6‰; February 2017) are enriched by at least 9.2‰ compared to the annual mean δ18Oprecipitation of -4.5‰ (Angesso station 2017; max: -1.9‰, min -6.2‰). This confirms that evaporative enrichment is able to explain a large part of the variability in the δ18Ofucose record (range of 11.5‰). Therefore, the reconstructed δ18Olake water record of Garba Guracha likely represents changes from an open to a closed lake system and wet/dry phases, and can be interpreted in terms of precipitation-to-evaporation ratio (P/E).
Comparison with other records
11–7 cal ka BP
Our δ18Ofuc record suggests a high P/E at the end of the Younger Dryas, shown by values increasing to a maximum at 11 cal ka BP. This is supported by a change from organic-poor to organic-rich sedimentation, indicating a shift to favorable growth conditions for aquatic algae (Bittner et al., Reference Bittner, Bliedtner, Grady, Gil-Romera, Martin-Jones, Lemma and Mekonnen2020). Erica expanded near the lake (Gil-Romera et al., Reference Gil-Romera, Adolf, Benito Blas, Bittner, Johansson, Grady and Lamb2019) at the onset of the Holocene (11.5–10 cal ka BP), which supports our interpretation of increased rainfall and rising P/E values. Along with such an increase, but lagged for some centuries (ca. 10.7 cal ka BP), wildfires were triggered in the Garba Guracha catchment (Gil-Romera et al., Reference Gil-Romera, Adolf, Benito Blas, Bittner, Johansson, Grady and Lamb2019). This is possible evidence that rainfall seasonality was in place at this time, permitting dry phases favoring fire, and facilitated by enhanced biomass accumulation (Gil-Romera et al., Reference Gil-Romera, Adolf, Benito Blas, Bittner, Johansson, Grady and Lamb2019). Meyer et al. (Reference Meyer, Van Daele, Tanghe, De Batist and Verschuren2020) suggested a coincident wet phase (11–9.5 ka) in the Lake Chala region due to changes in the intensity of the monsoon system.
However, a maximum of δ18Ofuc in the Garba Guracha sediments at 10.2 cal ka BP coincides with a lake level drop in Lake Turkana (Junginger et al., Reference Junginger, Roller, Olaka and Trauth2014; Bloszies et al., Reference Bloszies, Forman and Wright2015) and interrupts the trend towards more humid conditions (Fig. 5). Both δ18Odiatom and δ18Ofuc, but especially δ18Ofuc, indicate a pronounced phase of depleted δ18O values between 10 and 7 cal ka BP; suggesting a high P/E ratio due to increased rainfall amounts, a more permanent open lake phase with more constant overflow, and less evaporative enrichment. This is in agreement with the modeled overflow of Paleolake Suguta, Kenya, eastern Africa (Junginger et al., Reference Junginger, Roller, Olaka and Trauth2014) (Fig. 5). Junginger et al. (Reference Junginger, Roller, Olaka and Trauth2014) suggested that the lake reached its sill level several times between 10–8.5 cal ka BP and overflowed more or less continuously from 8.5 cal ka BP until ca. 7 cal ka BP. Enhanced moisture transport into the region, indicated by reconstructed high lake levels and stronger runoff during the AHP, is well documented in the literature (Tierney et al., Reference Tierney, Russell, Sinninghe Damsté, Huang and Verschuren2011b; Foerster et al., Reference Foerster, Junginger, Langkamp, Gebru, Asrat, Umer and Lamb2012; Tierney and deMenocal, Reference Tierney and deMenocal2013; Morrissey and Scholz, Reference Morrissey and Scholz2014; Fersi et al., Reference Fersi, Lézine and Bassinot2016; Liu et al., Reference Liu, Rendle-Bühring, Kuhlmann and Li2017; Wagner et al., Reference Wagner, Wennrich, Viehberg, Junginger, Kolvenbach, Rethemeyer, Schaebitz and Schmiedl2018; Beck et al., Reference Beck, Feibel, Wright and Mortlock2019; Jaeschke et al., Reference Jaeschke, Thienemann, Schefuß, Urban, Schäbitz, Wagner and Rethemeyer2020; Mologni et al., Reference Mologni, Revel, Blanchet, Bosch, Develle, Orange, Luc, Khalidi, Ducassou and Migeon2020).
A brief shift towards more positive δ18O values is present in all records coinciding with the 8.2 cal ka BP event in the northern hemisphere, known as a short cold spell in Greenland with reduced precipitation (Dansgaard et al., Reference Dansgaard, Johnsen, Clausen, Dahl-Jensen, Gundestrup, Hammer and Hvidberg1993; Bond et al., Reference Bond, Showers, Cheseby, Lotti, Almasi, deMenocal, Priore, Cullen, Hajdas and Bonani1997). A period of low lake levels and drought has also been reconstructed in eastern Africa for the Ziway-Shala system (Gillespie et al., Reference Gillespie, Street-Perrott and Switsur1983), Lake Malawi (Gasse et al., Reference Gasse, Barker, Johnson, Odada and Olago2002), Lake Turkana (Garcin et al., Reference Garcin, Melnick, Strecker, Olago and Tiercelin2012), Paleolake Suguta (Junginger et al., Reference Junginger, Roller, Olaka and Trauth2014), Lake Abhè (Gasse, Reference Gasse2000), and Lake Tilo (Leng et al., Reference Leng, Lamb, Lamb and Telford1999) at that time. Thompson et al. (Reference Thompson, Mosley-Thompson, Davis, Henderson, Brecher, Zagorodnov and Mashiotta2002) found a maximum in wind-blown fluoride derived from dry lake basins in the Kilimanjaro ice record at ca. 8400 cal BP. However, the increase in δ18Odiatom and δ18Ofuc in Garba Guracha is rather small in comparison with the range of most regional records. This might be due to a muted precipitation change in our high-altitude archive compared to lower altitude sites or due to a temperature change rather than a precipitation decrease.
After this drought phase, δ18Odiatom and δ18Ofuc decrease again, indicating a return to humid conditions and high lake levels (Fig. 5). With the start of declining boreal summer insolation at ca. 8.7 cal ka BP, the atmospheric circulation pattern changed, and the influence of the CAB decreased in eastern Africa (Junginger et al., Reference Junginger, Roller, Olaka and Trauth2014; Wagner et al., Reference Wagner, Wennrich, Viehberg, Junginger, Kolvenbach, Rethemeyer, Schaebitz and Schmiedl2018). This can be seen in our record since 7 cal ka BP when δ18Odiatom and δ18Ofuc values start to increase, indicating a shift to decreased precipitation and stronger evaporative enrichment. The phase between 10–7 cal ka BP indicates an overall increase in moisture availability concurring with the AHP.
7–4 cal ka BP
Strong variability in δ18Ofuc from 6–4 cal ka BP coincides with the termination of the AHP. The timing of the AHP termination has been debated, and seems to be related to individual proxy responses (Castañeda et al., Reference Castañeda, Schouten, Pätzold, Lucassen, Kasemann, Kuhlmann and Schefuß2016). Erica and fire experienced maxima and minima during this period at Garba Guracha, but consistently decreased towards absolute minima from 5.2–2.5 cal ka BP (Gil-Romera et al., Reference Gil-Romera, Adolf, Benito Blas, Bittner, Johansson, Grady and Lamb2019), while the Botryococcus braunii content (Umer et al., Reference Umer, Lamb, Bonnefille, Lézine, Tiercelin, Gibert, Cazet and Watrin2007) and TOC values drop later at 4.5 cal ka BP (Bittner et al., Reference Bittner, Bliedtner, Grady, Gil-Romera, Martin-Jones, Lemma and Mekonnen2020). This asynchronous proxy response may be related to the differential sensitivity of proxies to environmental change and individual drivers (Castañeda et al., Reference Castañeda, Schouten, Pätzold, Lucassen, Kasemann, Kuhlmann and Schefuß2016). Furthermore, differences in the timing of the response of various biological processes to climatic change make it difficult to assign cause and effect (e.g., perennial plants such as Erica may have declined, but seasonal organic matter production in the lake or transport into the lake could have continued, delaying any decline in TOC to later decades or centuries).
Despite strong variability, the mean trends in Garba Guracha δ18O values indicate a continuous transition from a humid phase before 7 cal ka BP towards a more stable arid phase after 4 cal ka BP. The more positive δ18O values after 4 cal ka BP suggest a dry climate with stronger evaporative enrichment and at least seasonally low lake levels.
In the regional context, contemporaneous decreases in lake levels have been found at north Ethiopian lakes Ziway-Shala and Abhé at ca. 4–4.5 cal ka BP (Gillespie et al., Reference Gillespie, Street-Perrott and Switsur1983; Gasse, Reference Gasse2000; Khalidi et al., Reference Khalidi, Mologni, Ménard, Coudert, Gabriele, Davtian and Cauliez2020). More southern lakes, including Paleolake Suguta, Lake Turkana, and Chew Bahir, show an earlier AHP termination at ca. 5–5.5 cal ka BP (Foerster et al., Reference Foerster, Junginger, Langkamp, Gebru, Asrat, Umer and Lamb2012; Garcin et al., Reference Garcin, Melnick, Strecker, Olago and Tiercelin2012; Junginger et al., Reference Junginger, Roller, Olaka and Trauth2014).
4 cal ka BP to present
The most positive values of δ18Odiatom in the entire record coincide with very positive δ18Ofuc values at 3.5 cal ka BP, suggesting a reduced P/E ratio and a low lake level at Garba Guracha. After 3.5 cal ka BP, the δ18Odiatom and δ18Ofuc values decrease, indicating a higher P/E ratio and increased lake level. In response to wetter conditions at 2.5 cal ka BP, an expanding heathland and more active fires may have occurred (Gil-Romera et al., Reference Gil-Romera, Adolf, Benito Blas, Bittner, Johansson, Grady and Lamb2019). However, we cannot rule out a human origin for these fires, and subsequent re-expansion of Erica at particular areas within the basin because anthropogenically induced changes have been detected at lower altitudes during this period (Bonnefille and Mohammed, Reference Bonnefille and Mohammed1994). Our findings are consistent with those of Gasse and Van Campo (Reference Gasse and Van Campo1994) who found a more humid phase in eastern Africa at ca. 2.5 cal ka BP. The diatom record of Lake Ashenge also shows a humid phase between 2.5–1.5 cal ka BP (Machado et al., Reference Machado, Pérez-González and Benito1998). Moreover, a data comparison presented by Lezine et al. (Reference Lezine, Bassinot and Peterschmitt2014) indicates increased humid conditions at the Horn of Africa between 3.5–1.5 cal ka BP.
During the last 1.5 cal ka BP, decreasing δ18Ofuc and δ18Odiatom values in Garba Guracha support a shift towards increased dryness at Lake Tana (documented by Marshall et al., Reference Marshall, Lamb, Huws, Davies, Bates, Bloemendal, Boyle, Leng, Umer and Bryant2011). A minimum in δ18Ofuc at 0.5 cal ka BP may coincide with the timing of the Little Ice Age (Grove, Reference Grove2004).
CONCLUSIONS
In eastern Africa, and especially in the Horn of Africa region, the relative scarcity of high-altitude climate proxy reconstructions inhibits understanding of regional climate change and ecosystem reaction during the Holocene and earlier. The continuous Garba Guracha archive with a high-resolution chronology allows us to present and interpret the first high-altitude (>3500 m asl) δ18Ofuc and δ18Odiatom records from the Horn of Africa. Because of the small catchment area of Garba Guracha, the sugar biomarker fucose is primarily of aquatic origin. The similar trends of δ18Ofuc and δ18Odiatom results therefore indicate that δ18Ofuc is a valuable proxy for δ18Olake water.
Besides possible variation in the source effect, we conclude that in the case of reconstructed δ18Olake water record, the effect of evaporative enrichment has to be considered to gain a better understanding of eastern African climate change. We conclude that, in general, precipitation increased at the beginning of the Holocene, leading to a primarily open lake system between ca. 10 and ca. 8 cal ka BP, with lowest δ18Olake water values due to increased precipitation and reduced evaporative enrichment (lower P/E). When the lake was open and overflowing, the δ18Olake water was less affected by evaporative enrichment, so the evaporative signal did not overprint the precipitation source and amount effects in the δ18Olake water values.
At ca. 7 cal ka BP, a continuous shift towards drier conditions began, indicating a change towards a predominantly closed lake system. Small minima, representing phases of lower P/E, occurred at 10.8 cal ka BP, 10.2 cal ka BP, 3.5 cal ka BP, and 0.5 cal ka BP. At the temporal resolution of the Graba Guracha record, the 4.5 cal ka BP and 8.2 cal ka BP drought phases seen in eastern African lake records are not strongly imprinted in our isotope records and may point to a buffered and/or reduced response at high altitudes. Despite differences in apparent intensity, reconstructed dry and wet phases are broadly coincident with Rift Valley records, indicating similar climate controls. The Rift Valley records are influenced by eastward migration of the Congo Air Boundary, allowing Atlantic moisture to enter the region. However, the Garba Guracha record shows no evidence that the influence of Congo Basin air masses extended farther eastwards into the Bale Mountains in the past. Further studies are needed to disentangle the source and amount effects and evaporative enrichment in stable isotope records from eastern Africa.
Supplementary Material
The supplementary material for this article can be found at https://doi.org/10.1017/qua.2021.26.
Acknowledgments
We are grateful to the project coordination, the Philipps University Marburg, University of Addis Ababa, the Frankfurt Zoological Society, the Ethiopian Wolf Conservation Programme, the Bale Mountains National Park, and the related staff members, especially Katinka Thielsen and Mekbib Fekadu for their logistic assistance during our fieldwork. We thank the Ethiopian Wildlife Conservation Authority for permitting our research in the Bale Mountains National Park. We thank the team of the Soil Biogeochemistry Department at Martin Luther University Halle Saale for support during lab work, in particular Marianne Benesch and Heike Maennike. We thank Dr. Miguel Sevillia-Callejo for creating the maps in Figure 1 and 2. We are grateful to two anonymous reviewers for their helpful and detailed work improving the publication.
Author Contributions
LB, DG, GGR, HFL, and MZ collected the samples. LB and MZ developed the concept. EL, MW, and HM analyzed and interpreted the diatom data. TB, BG, MZ, and LB analyzed and interpreted the sugar biomarker data. LB led the manuscript writing with contributions and feedback from all authors. MZ acquired the funding and supervised the work.
Financial Support
This research was funded by the German Research Council (DFG) in the framework of the joint Ethio-European DFG Research Unit 2358 “The Mountain Exile Hypothesis. How humans benefited from and re-shaped African high-altitude ecosystems during Quaternary climate changes.”
Conflicts of Interest
The authors declare that they have no conflict of interest.