In mammals, the placenta is a bidirectional exchange organ that provides metabolic substrates for fetus growth( Reference Bell and Ehrhardt 1 ), and also removes waste products of the fetus. Placental structures can be classified into hemochorial and epitheliochorial types( Reference Barry and Anthony 2 – Reference Ward, Forhead and Wooding 4 ). The placenta of humans, rodents and primates belongs to the former and consists of three fetal cellular layers: the syncytiotrophoblast, villous stromal tissue and capillary endothelium( Reference Sibley, Brownbill and Dilworth 5 ). Other species, such as ruminants, monogastric animals and carnivores, have an epitheliochorial type of placenta, whereas the placenta of ruminants is quite different from that of monogastric animals in anatomy and belongs to cotyledonary and synepitheliochorial placenta( Reference Wooding 6 ) where fetomaternal exchange takes place at discrete sites named placentomes. Each placentome is divided into the maternal portion called uterine caruncle (endometrial depression) and fetal portion named cotyledon (adhesions in group villi caruncle). There is a large amount of evidence showing that the anatomy, physiology, endocrinology, blood flow and vascularity of the first-trimester placenta are different from the placenta of the second and third trimesters( Reference Igwebuike and Ezeasor 7 – Reference Rodesch, Simon and Donner 14 ). Hence, placental functions vary with stages of development and the demands of the fetus.
The placenta has been recognised as playing important roles in nutritional programming of adult diseases( Reference Burton and Fowden 15 ), either via dietary modulation of the placental transport of hormones( Reference LangleyEvans, Phillips and Benediktsson 16 ) or variation in the delivery of key substrates to the developing fetus( Reference Spencer, Dickson and Hennig 17 ). In addition, placental development and function are also related to neonatal behaviour of lambs and goats( Reference Dwyer, Calvert and Farish 18 , Reference Konyali, Tolu and Das 19 ). Therefore, the placenta may be the centre of the response to maternal undernutrition and the transfer of signals of adverse conditions from mother to fetus.
Maternal nutrition during gestation plays an essential role in the regulation of placental development in ruminants. Maternal protein restriction during mid-gestation and late gestation influences the placental structure and organisation in rats and mice( Reference Gao, Yallampalli and Yallampalli 20 – Reference Daniel, Swali and Emes 23 ), whereas protein limitation at early pregnancy alters the placental growth in bovine( Reference Perry, Norman and Owen 24 ). Energy deprivation in pregnant sheep can also affect placental weight( Reference Heasman, Clarke and Firth 25 , Reference Osgerby, Wathes and Howard 26 ) and morphology( Reference Steyn, Hawkins and Saito 27 ) in a stage-dependent manner. High dietary intake during early to mid-pregnancy has been demonstrated to influence the capillary development in the fetal portion of the placenta in adolescent sheep( Reference Redmer, Luther and Milne 28 ). Although studies linking dietary nutrition and placental development have been carried out in bovine, sheep and other mammals, very few studies have addressed the placenta traits and angiogenesis gene expression in gestational goats affected by undernutrition.
Placentome growth in terms of mass and net cellular proliferation reaches a maximum by approximately day 80 of gestation( Reference Ehrhardt and Bell 29 ) (total gestation 150 d for goats), implying that placental size and function in later gestation may be substantially uninfluenced in goats. Therefore, the hypothesis for this study using goats as the experimental animal model was that feed intake restriction at two critical time points during pregnancy differently affects fetal growth, placental traits, angiogenesis gene expression and proteome. Therein, day 65 represents the early stage before the placenta attains the maximal weight, and day 125 corresponds to the period of rapid fetal growth when the placenta does not gain weight( Reference Ehrhardt and Bell 29 , Reference Wallace, Bourke and Aitken 30 ). Our study provides a theoretical basis for the rational feeding and management of pregnant goat periods of feed shortage.
Methods
All procedures, including animal welfare, husbandry and experimental procedures used in this study, were approved by the Animal Care Committee, Institute of Subtropical Agriculture, the Chinese Academy of Sciences, Changsha, China.
Experimental design and sampling
Experimental diets were formulated according to the Feeding Standard of Meat-producing Goats (NY/T816-2004). Ingredients and nutrient compositions of the diets are presented in Table 1. Ration composition included forage and concentrate. Fresh Miscanthus was selected as forage, cut into small pieces and mixed with concentrate before feeding. All goats were fed in two equal amounts daily at 08.00 and 17.00 hours, and had free access to clean water.
Table 1 Ingredients and composition of the experimental diets (DM basis)

* Per kg of premix contains vitamin A 95 000 IU, vitamin D 17 500 IU, vitamin E 18 000 IU, MgSO4.H2O 119 g, FeSO4.7H2O 2·5 g, CuSO4.5H2O 0·8 g, MnSO4.H2O 3 g, ZnSO4.H2O 5 g, Na2SeO3 10 mg, KI 40 mg, CoCl2.6H2O 30 mg.
In all, twenty-four Xiangdong black goats (a local breed in Liuyang, a city in south China) with similar age (2·0 (sd 0·3) years old) and parity were used as experimental animals. The goats were oestrous-synchronised. A total of twelve twin-bearing pregnancies were designated as early pregnant trial animals (body weight (BW) 31·2 (sd 8·1) kg, trial 1), and the other twelve twin-bearing goats as late-pregnant test animals (BW 36·0 (sd 8·2) kg, trial 2). The nutritional treatment for the early-pregnant trial animals ran from days 26 to 65 after synchronisation, and for the late pregnancy trial animals from days 96 to 125. At the beginning of the test, all the pregnancies had free access to the ration until day 22 (trial 1) or day 92 (trial 2) and then randomly divided into the control group (CG, n 6, 100 % feed) and the restricted group (RG, n 6, 60 % feed of CG), respectively. Each goat was housed in a single cage, adapted to the experimental diets for three days and daily feed intake was calculated.
All the goats were fasted for 12 h before sampling. Maternal blood samples were collected by the jugular vein from both groups into heparinised tubes, kept for 2 h at room temperature and then centrifuged at 3000 g for 10 min at 4°C. The supernatant serum was collected and analysed for nitric oxide (NO) content immediately. The remaining samples were stored at −80°C for subsequent analysis. After blood sampling, goats were weighed, bled and slaughtered. The entire placenta of each goat was weighed. Samples of placental tissue from the area that surrounded the umbilical cord site of attachment to the uterine epithelium of each fetus were collected avoiding blood vessels. Samples were rinsed with cold sterile saline and put on ice. Six placentomes of similar size were obtained for each umbilical cord. Next, uterine caruncle and cotyledon were separated, weighed and counted. Uterine caruncle samples were quickly wrapped with sterilised tinfoil, frozen in liquid nitrogen and stored at −80°C for further analysis. Cotyledon samples in late-pregnant test (trial 2) that were close to 1×1 cm2 were collected and washed with PBS (containing 0·85 % NaCl, 1·4 mm KH2PO4 and 8 mm Na2HPO4, pH=7·4), fixed in 10 % formalin (v/v) for 1 d, embedded in paraffin wax and stored at 4°C until further immunohistochemistry analysis. Placental relative weight was defined as the ratio of placental weight:goat body weight. Relative weight of uterine caruncle or cotyledon was calculated as the ratio of uterine caruncle or cotyledon weight:placental weight. Average weight of uterine caruncle or cotyledon meant the average weight of each uterine caruncle or cotyledon. Density of cotyledon was calculated as the number of cotyledons per gram placental weight.
Serum parameters and nitric oxide determination
Serum vascular endothelial growth factor (VEGF) level was determined using an ELISA kit (Donggeboye Biological Technology Co., Ltd). Total bile acid (TBA), nitric oxide synthase (NOS) and NO contents in serum samples were analysed by colorimetric method of biochemical kits (Nanjing Jiancheng Bioengineering Institute) according to the manufacturer’s instructions. Uterine caruncle and cotyledon tissues were homogenised in a high-speed blender, centrifuged (12 000 rpm/min, 4°C, 1 h) and the supernatants were obtained and then analysed for NO level by nitrate reductase method, same as serum samples. Total protein concentrations of caruncle and cotyledon tissues were detected using the BCA kit (Beyotime Institute of Biotechnology).
Immunohistochemical analysis
Pre-embedded samples were cut into sections of 5-μm thickness using a manual rotary microtome (Leica RM2235; Leica Microsystems) and adhered to positively charged adhesion slides (Beyotime). The following procedures were performed according to the instructions of immunohistochemical kit manufacturer. In brief, slides were dewaxed and rehydrated, and then incubated with primary antibody CD34 (primary antibody from rabbit; Aviva Systems Biology) at 4°C overnight. After washing, slides were incubated with HPR (anti-rabbit from instant IHC kits; Sangon Biotech) at 37°C for 30 min. Negative control was conducted with PBS instead of primary antibody. There was no positive dye presented in the negative control, demonstrating that the antibody we used had reliable specificity. Microvessel density was determined by light microscopy in areas of staining sections containing the greatest number of capillaries and small venules (microvessels) per area. Briefly, the vascular hotspots were identified at low power (100×). Then the areas having the greatest number of CD34 antigen staining microvessels were identified. Individual microvessel counts were observed using a 400× field within the hotspot, and at least five different areas were selected in each section. Any endothelial cell or endothelial cell cluster being positive for CD34 antigen and clearly separate from an adjacent cluster was considered to be a single, countable microvessel( Reference Weidner, Folkman and Pozza 31 ). Results were expressed as the average number of microvessels identified by two experimenters within five 400× fields.
Quantitative real-time PCR
Total RNA was extracted from uterine caruncle and cotyledon tissues using TRIZOL (Invitrogen) according to the manufacturer’s instructions. After genomic DNA was eliminated by digestion with DNase I (Thermo Scientific), the RNA quality and quantity were determined using NanoDrop 2000 (Thermo Scientific), and all RNA samples showed A260/A280 values within the range of 1·9–2·0. In addition, the integrity of collected RNA was analysed with gel electrophoresis. Next, 1 μg of the extracted RNA was reverse-transcribed to synthesise tissue-specific complementary DNA (cDNA) using the PrimeScript™ RT reagent Kit (Takara,) immediately. The prepared cDNA samples were stored at −20°C until subsequent quantitative real-time PCR analysis.
For relative quantification of gene expression, the ABI Prism 7900 HT Fast real-time PCR System (Applied Biosystems) was used. Primers were designed using the Primer 3 plus program, and sequences are listed in Table 2. The specificity of designed primers was checked by online Primer-BLAST (NCBI) and subsequent gel electrophoresis analysis for the proper product size, as well as melt curve analysis during quantitative real-time PCR. The reaction system, thermal profile for all reactions and fluorescence monitoring time were the same as our previous detection( Reference Li, Tong and Yan 32 ). Each real-time reaction was completed with a melting curve analysis to ensure the specificity of the reaction. All the samples were analysed in duplicate, and the relative amount of each specific transcript was obtained after normalisation against the endogenous control β-actin. The relative changes in mRNA expression levels were calculated according to the 2-ΔΔCt method( Reference Livak and Schmittgen 33 ), where ∆∆C t (sample−control)=(C t target gene−C t β-actin) for the sample−(C t target gene−C t β-actin) for the control.
Table 2 Information of primers used in the real-time quantitative PCR

VEGFA, vascular endothelial growth factor A; F, forward; R, reverse; NOS1, nitric oxide synthase 1; NOS2, nitric oxide synthase 2.
Protein lysis, digestion and isobaric tag for relative absolute quantitation proteomics analysis
To extract proteins from placental samples, frozen and ground tissues were incubated in a lysis buffer containing 7 m urea, 2 m thiourea, 100 mm DTT, 5 % SDS( Reference Zhang, Guo and Guo 34 ) and protease inhibitor cocktail (each tablet is sufficient for a volume of 50 ml of extraction solution, Roche Diagnostics Ltd) at 4°C for 1 h, and then insoluble molecules were removed by centrifugation at 40 000 g for 1 h at 4°C. The supernatant was collected, and protein concentration was determined by the RC DC Protein Assay (Bio-Rad Laboratories, Inc.). The samples were stored at −80°C until use.
In-solution digestion of protein and isobaric tag for relative absolute quantitation labelling
Total proteins of placental samples were reduced with 5 mm TRIS-(2-carboxyethyl) phosphine at 60°C and then alkylated with 10 mm methyl methanethiosulfonate (MMTS) for 30 min at room temperature. After the proteins were digested overnight at 37°C with sequencing-grade modified porcine trypsin (Promega), the peptides were dried in a SpeedVac and stored at −80°C until further use. For isobaric tag for relative absolute quantitation (iTRAQ) labelling, the tryptic peptides were reconstituted in iTRAQ reagent buffer, and the four groups of tissue extracts were separately labelled with eight different iTRAQ labelling reagents (for goats in Trial 1: C1:113, C2:114, R1:115, R2:116, R3:117, R4:118, C3:119, C4:121; for goats in Trial 2: C1:113, C2:114, C3:115, C4:116, R1:117, R2:118, R3:119, R4:121), according to the manufacturer’s instructions (Applied Biosystems Inc.). The labelled samples of each trial were then mixed and dried.
Off-line two-dimensional liquid chromatography-MS/MS
The mixed peptides were fractionated by strong cation exchange chromatography on a 20AD HPLC system (Shimadzu) using a polysulfoethyl column (2·1×100 mm, 5 μm, 300 Å; The Nest Group Inc.). In brief, the mixed peptides were desalted with Sep-Pak Cartridge (Waters), diluted with the loading buffer (10 mm KH2PO4 in 25 % acetonitrile, pH 2·8) and loaded onto the column. Buffer A was identical in composition to the loading buffer, and buffer B was same as buffer A except containing 350 mm KCl. Separation was performed using a linear binary gradient of 0–80 % buffer B in buffer A at a flow rate of 200 μl/min for 60 min. The absorbance at 214 and 280 nm was monitored, and a total of twenty strong cation exchange fractions were collected along the gradient.
Each fraction was dried down by the rotary vacuum concentrator, and then dissolved in solvent A (5 % acetonitrile and 0·1 % formic acid) and analysed on TripleTOF 5600 systems (AB SCIEX) in an information-dependent mode. In brief, peptides were separated on a reverse-phase column (ZORBAX 300SB-C18 column, 5 μm, 300 Å, 0·1×15 mm; Micromass) using an Eksigent 1D PLUS system (AB SCIEX) at an analytical flow rate of 300 nl/min. The peptides were separated with a 120-min linear gradient from 5 to 40 % solvent B (0·1 % formic acid/90 % acetonitrile). Survey scans were acquired from 400 to 1500 with up to fifteen precursors selected for MS/MS and dynamic exclusion for 20 s.
Protein identification, grouping, quantitation and bioinformatic analysis
Protein identification, grouping and quantitation were performed using Paragon and Pro Group algorithm in ProteinPilot™ 4·2 (Applied Biosystems). The data analysis parameters were set as follows: (1) Sample Type, iTRAQ 8plex (Peptide Labelled); (2) Cysteine Alkylation, MMTS; (3) Digestion, Trypsin; (4) Instrument, TripleTOF 5600; (5) Special Factors, None; (6) Species, Homo sapiens; (7) ID Focus, Biological modifications; (8) Database, NCBI Capra hircus database (release Nov 2015); (9) Search Effort, Thorough; (10) Max missed cleavages; (11) FDR Analysis, Yes; (12) User-Modified Parameter Files, No; (13) Bias Correction, Auto; (14) Background Correction, Yes. Identified proteins were grouped by the software to minimise redundancy. All peptides used for the calculation of protein ratios were unique to the given protein or proteins within the group, and peptides that were common to other isoforms or proteins of the same family were ignored. A decoy database search strategy was adopted to estimate the false discovery rate (FDR) for peptide identification. For our iTRAQ experiments, a strict unused confidence score cut-off is 1·3, which corresponds to a peptide confidence level of 95 %. With this filter, the corresponding FDR was calculated by searching against a concatenated reversed database. The results were then exported into Microsoft Excel for manual data interpretation.
Functional analysis of differentially expressed proteins identified was conducted using Gene Ontology annotation (http://www.geneontology.org/) and proteins were categorised according to their biological process, molecular function and cellular localisation. The COG database (NCBI) and Ingenuity Pathway Analysis (content version: 17199142) were used to classify these identified proteins and enrich pathways related and networks.
Statistical analysis
The general linear model procedure of SPSS 21 Statistical Software (SPSS Inc.) was used for statistical analysis. Dietary treatment was used as the fixed effect, and goat was the random effect. Fetal weight, placental traits, serum parameters and gene expression data were analysed by covariance analysis using feed intake/(mother goat initial weight)0·75 as the covariate. Data are presented as means and standard deviations. P values<0·05 were considered significant.
Results
Placental traits and fetal weight
During the early pregnancy trial, total weight and relative weight of placenta, uterine caruncle and cotyledon, and fetal weight in RG were increased (P<0·05, Table 3). During late pregnancy (trial 2), the placental weight was increased (P<0·05) in RG, but the weight of fetal goat in RG was decreased (P<0·05), and the capillary density in the cotyledon in RG was also decreased (P<0·01, Fig. 1).
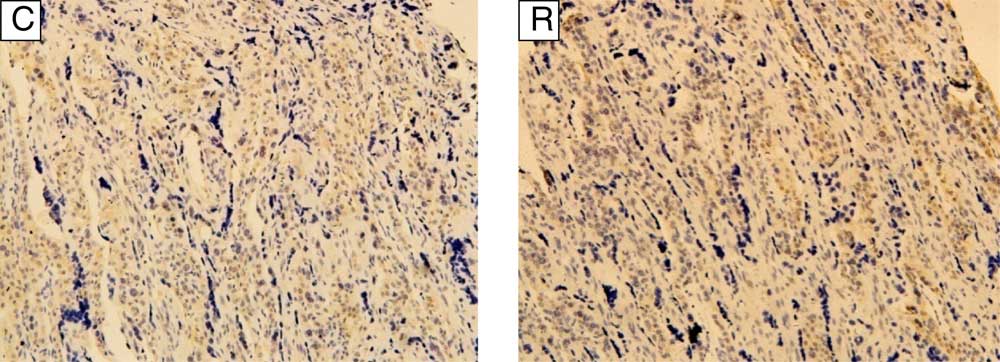
Fig. 1 Distribution of blood vessels in the cotyledon of goats during late pregnancy (400×). CD34 expression (brown) in the placental cotyledon of goats in control (C) group was 38·80 (sd 1·33) villus blood vessels per sight, whereas in restricted (R) group it was 29·06 (sd 1·00) villus blood vessels per sight (P<0·01).
Table 3 Placental traits of pregnant goats and body weight of the fetusesFootnote † (Mean values and standard deviations)

CG, control group; RG, restricted group.
Means: * P<0·05, ** P<0·01.
† n 6: CG and RG.
Serum parameter and placental nitric oxide
During early pregnancy, the content of serum NO in maternal goats in RG was increased (P<0·05, Table 4). The VEGF, TBA, inducible NOS (INOS) and total NOS in the serum, and NO contents in uterine caruncle and cotyledon in RG, were not affected (P>0·05). During late pregnancy, intake restriction had no effect (P>0·05) on the serum parameters and placental NO concentration.
Table 4 Serum parameters and placental nitric oxide (NO) concentration in pregnant goatsFootnote † (Mean values and standard deviations)

CG, control group; RG, restricted group; VEGF, vascular endothelial growth factor; TBA, total bile acid; INOS, inducible nitric oxide synthase; TNOS, total nitric oxide synthase.
Mean: * P<0·05.
† n 6: CG and RG.
Angiogenesis gene expression in the uterine caruncle and cotyledon
As shown in Table 5, the mRNA expression of genes associated with angiogenesis in the uterine caruncle and cotyledon of RG goats, including VEGFA, kinase insert domain receptor, NOS1 and NOS2, were not affected (P>0·05).
Table 5 Expression of genes associated with angiogenesis in the placentome of pregnant goatsFootnote * (Mean values and standard deviations)

CG, control group; RG, restricted group; VEGFA, vascular endothelial growth factor A; KDR, kinase insert domain receptor; NOS1, nitric oxide synthase 1; NOS2, nitric oxide synthase 2.
* n 6: CG and RG.
Protein expression in the cotyledon
A total of fifty differentially expressed proteins were detected in the placental cotyledon during early pregnancy (Table 6), therein twenty-seven proteins were up-regulated and twenty-three proteins were down-regulated. Functional classification (Fig. 2(a)) showed that the differentially expressed proteins were mainly involved in transcription, replication, recombination and repair, post-translational modification, protein turnover, chaperones and signal transduction mechanisms. Further analysis (Fig. 3(a)) showed that most up-regulated proteins were located in extra-cellular exosome, cytoplasm and membrane, and functioned as protein and metal ion binding, hydrolase and oxidoreductase activities and participated in oxidation–reduction, transport and carbohydrate metabolic processes. Meanwhile, most down-regulated proteins were membrane-, cytoplasm- and nucleus-associated, involved in ATP, protein and nueleotide binding, and in charge of regulation of DNA-templated transcription, signal transduction and metabolic process.

Fig. 2 Functional classification of the differentially expressed proteins in cotyledon of goats in early gestation (a) and late gestation (b).

Fig. 3 Classification of the differentially expressed proteins in cotyledon of goats during early gestation (a) and late gestation (b). , Up;
, down.
Table 6 Differentially expressed proteins in the placental cotyledon of goats during early gestationFootnote *

RG, restricted group; CG, control group.
* This table contains quantitative information for proteins that were at least >1·2 up-regulated or at least<0·8 down-regulated in early pregnant goats with feed intake restriction.
In all, 58 differentially expressed proteins were identified in the placental cotyledon during late pregnancy, including thirty-eight up-regulated proteins and twenty down-regulated proteins (Table 7). These differentially expressed proteins mainly functioned in the areas of energy production and conversion, amino acid transport and metabolism, translation, ribosomal structure and biogenesis and post-translational modification, protein turnover and as chaperones (Fig. 2(b)). Specifically, up-regulated proteins were located in the extra-cellular exosome, cytosol and nucleus, and were involved in Poly(A) RNA and DNA binding, structural constituent of ribosome, inflammatory response and regulation of lipid metabolic process (Fig. 3(b)).
Table 7 Differentially expressed proteins in the placenta cotyledon of goats during late pregnancyFootnote *

RG, restricted group; CG, control group.
* This table contains quantitative information for proteins that were at least >1·2 up-regulated or at least<0·8 down-regulated in late pregnant goats with feed intake restriction.
The top ten enrichment pathways of differentially expressed proteins identified are presented in Fig. 4. During early gestation, signals including glycogen degradation, phosphatidylinositol 3 kinase (PI3K)/non-specific serine/threonine protein kinase (AKT) and G2/M DNA damage checkpoint regulation were affected by intake restriction, whereas EIF2 signalling, regulation of eukaryotic translation initiation factor 4 (eIF4) and p70S6K signalling and mammalian target of rapamycin (mTOR) signalling were affected by feed intake restriction during late pregnancy.

Fig. 4 Enriched pathways of differential expressed proteins in cotyledon of goats during early pregnancy (a) and during late pregnancy (b). mTOR, mammalian target of rapamycin; PI3K, phosphatidylinositol 3 kinase; AKT, non-specific serine/threonine protein kinase; ERK5, mitogen-activated protein kinase 5.
Interactive network analysis of the differentially expressed proteins of placental cotyledon in goats during early gestation showed that the up-regulated proteins, such as EIF4E, collagen family members and AKR1B1, were related to proliferation and fission of trophoblast cell and the placenta angiogenesis (Fig.5(a)). Meanwhile, interactive network analysis of the placental cotyledon during late pregnancy indicated that up-regulated proteins such as mitogen-activated protein kinase 1 (ERK1/2) and 1-phosphatidylinositol 4,5-bisphosphate phosphodiesterase γ-2 (PLCG2) were related to placenta formation, blood flow regulation and embryonic development (Fig. 5(b)). In addition, down-regulated proteins, such as RNA-binding protein 4 isoform X1 (RBM4), Rho-related GTP-binding protein rhoc (RHOC) and voltage-dependent anion-selective channel protein 1 (VDAC1), interacted together to regulate placental trophoblastic cell migration, anion transport, apoptotic process and vascular blood flow regulation.

Fig. 5 Network of protein interactions among the differentially expressed proteins in cytoledon of goats during early pregnancy (a) and during late pregnancy (b).
Discussion
In the present study, feed intake restriction during early pregnancy allowed both the development of placentae and the growth of fetuses. The high positive correlation between fetal weight and placental and cotyledon weight concurred with previous studies in goats and cows( Reference Konyali, Tolu and Das 19 , Reference Perry, Norman and Owen 24 ). Heasman et al. ( Reference Heasman, Clarke and Firth 25 ) have also observed a higher placental weight with the abundance of placentomes in energy-restricted sheep without difference in fetal birth weight. Our results are partly consistent with those of Steyn et al. ( Reference Steyn, Hawkins and Saito 27 ), who observed an increment of placental growth without differences in fetal weight of sheep that received reduced nutrition during early gestation. The synergetic adaptations in the weight of placenta, uterine caruncle and cotyledon rather than their numbers contributed to the enhanced fetal weight, suggesting that placentome function such as nutrient transport efficiency may be boosted by feed intake restriction at early gestation. Limited feeding promoted the placental weight of goats, but inhibited the growth of fetuses during late pregnancy. Redmer et al. ( Reference Redmer, Milne and Aitken 35 ) have also observed the same phenomenon in adolescent sheep. However, Osgerby et al. ( Reference Osgerby, Wathes and Howard 26 ) have ever reported that the placental weight is reduced in under-fed ewes receiving 70 % of their estimated requirements. The contrary responses in development of placentae and fetuses in late gestation indicate that a combination of factors originating from maternal placental and fetal sources act together to regulate the growth of fetuses. A reduction of blood vessels in the cytoledon of restricted goats was also observed in this study. This inferred that the nutrient transfer rate from mother to fetus had been deceased and could partly explain the cause of intra-uterine growth restriction at this stage.
To further check whether feed intake restriction influenced placental or embryonic growth via maternal humoral factors, we measured VEGF, TAB, NO and NOS in the serum and related angiogenesis genes in the placentome of pregnant goats. The NO radical is synthesised by the action of the enzyme NOS and iNOS becomes a dominant NOS isoform during early pregnancy. It is reported that serum iNOS is greater in intra-uterine growth retardation pregnancies and correlates positively with insulin resistance( Reference Morteza, Abdollahi and Bandarian 36 ), which is helpful to increase nutrition availability in maternal circulation. Fortunately, we found that circulating NO of maternal goats was increased during early pregnancy, accompanied by more NOS2 transcription in uterine caruncle. Previous research has shown that NO production is required for normal embryonic development and works as a signal to stimulate local vasodilation and capillary permeability( Reference Gouge, Marshburn and Gordan 37 ). The NO also plays important roles in female reproduction, ovarian follicular development, secretion of ovarian hormones, implantation, pregnancy maintenance and parturition( Reference Dubey, Tripathi and Singh 38 – Reference Sagar, Prasad and Prasad 40 ). Our results reflected that, during early pregnancy, nutrient restriction induced the embryo producing NO as a messenger molecule to maintain the pregnancy and embryonic growth by increasing placental blood flow and nutrient supply.
In this study, we successfully identified a total of fifty differentially expressed proteins (twenty-seven up-regulated and twenty-three down-regulated) in the placentome of malnourished goats during early gestation. We also discovered that some important functional proteins including AKR1B1, EIF4E and collagen family members, which are related to proliferation and division of trophoblast cells and placental angiogenesis, were up-regulated after IPA network analysis. Increasing amounts of AKR1B1 expression were particularly significant. AKR1B1 belongs to the aldo-keto reductase family and is expressed in bovine endometrium. It works as a candidate enzyme for controlling the sufficient and timely production of PGF2α being responsible for recognition of pregnancy( Reference Madore, Harvey and Parent 41 , Reference Lacroix Pepin, Chapdelaine and Fortier 42 ). Expression levels of collagen, collagen type I, collagen type VI, COL6A1 and COL18A1 were increased in early-pregnant goats experiencing feed intake restriction. All of them belong to the collagen superfamily, which are components of extra-cellular matrix and are involved in the regulation of cell proliferation, differentiation and gene expression. Previous studies have shown that types I, III, IV, V and XVIII can be detected in the human placenta( Reference Rukosuev 43 , Reference Saarela, Ylikarppa and Rehn 44 ). The increase of collagen family members suggested that the synthesis of collagen and the extra-cellular matrix–receptor interaction may be enhanced. EIF4E is located in the centre of the network and participated in the PI3K/AKT pathway, which was strongly influenced by nutrient restriction during early pregnancy. EIF4E has an important role in the regulation of initial stage of the normal synthesis of cap structure-dependent protein and promotes the proliferation and survival of trophoblast cells. EIF4E gene silencing in placental explants can accelerate cell death and inhibit cell cycle( Reference Kitroser, Pomeranz and Shochet 45 ). Up-regulation of EIF4E and PI3K/AKT pathway indicated that the proliferation and survival of placental cells were improved to maintain the development of the placentome.
During late pregnancy, fifty-eight differentially expressed proteins were identified in the placentome, comprising thirty-eight up-regulated and twenty down-regulated proteins. Specifically, up-regulated proteins ERK1/2 and PLCG2 were found to be related to placental formation, blood flow regulation and embryonic development. Down-regulated proteins RHOC, RBM4a and VDAC1 were related to trophoblast migration and vascular regulation according to the IPA network. One potential function of ERK1/2 on the development of placentae may be direct action. Previous research showed that in the condition of intra-uterine growth restriction induced by dexamethasone, abnormal placental and embryonic development may be associated with reduced Akt and ERK1/2 activities, manifesting as cell survival inhibition or apoptosis( Reference Ozmen, Unek and Kipmen-Korgun 46 ). Another possible regulatory manner of ERK1/2 on the development of placentae might be via blood vessels and blood flow; Wu et al. ( Reference Wu, Komori and Qin 47 ) have founded that ERK inhibitor U0126 significantly attenuates the vasodilator response induced by spinal cord stimulation. Zheng et al. ( Reference Zheng, Wen and Song 48 ) have also noted that activation of the ERK1/2-mitogen-activated protein kinase 3/1 pathway is critical for fibroblast growth factor 2 and VEGF-stimulated ovine fetoplacental endothelial cell proliferation. PLCG2 is involved in leptin, ERK/MAPK, p70S6K, melatonin and chemokine pathways, and its abundance was increased by nutrient restriction in this study. It has been reported that PLCG2 is an important regulator of embryonic cerebral cortices( Reference Rout and Dhossche 49 ) and osteoclast( Reference Epple, Cremasco and Zhang 50 ) development by mediating integrin receptor signalling. RHOC expression in the cotyledon of malnourished goats was inhibited in this study. As a member of the Rho family of small G proteins, RHOC and RHOA are required for epidermal growth factor-mediated trophoblast cell migration and obligatory for epidermal growth factor action( Reference Han, Li and Hu 51 ). RBM4a usually participates in translation suppression during muscle cell differentiation( Reference Lin and Tarn 52 ). Its role in placental development needs to be revealed in the future. VDAC1 is involved in calcium metabolism of the placenta and regulates the balance of mitochondrial Ca2+. VDAC1 is selectively localised in the basal membrane fraction of human placenta, and its expression in the placenta from pregnancies with fetal growth restriction is up-regulated by hypoxia( Reference Oh, Hwang and Lee 53 ). Less abundances of RHOC and VDAC1 in cotyledon of under-fed goats during late pregnancy indicated that trophoblast cell migration, vascular nutrient exchange rate and calcium ion homoeostasis might have been disrupted.
Conclusion
Feed intake restriction during early gestation regulated placental and fetal development, increased serum NO production and regulated glycogen degradation, PI3K/AKT and cell cycle signalling transduction pathways, as well as functional proteins EIF4E, collagen family members and AKR1B1, in the placentome of goats. Nutrient restriction during late gestation increased placental weight, but induced fetal growth restriction. Decreased blood vessel density in cotyledon, differential signalling pathways of EIF2, regulation of eIF4 and p70S6K, mTOR, leptin and ERK/MAPK, and related key proteins ERK1/2, PLCG2, RHOC, VDAC1 and RBM4 may be associated with placental function. It is apparent that effects of nutrient restriction on goat placental traits and proteome, and fetal development, are stage-specific. The regulatory mechanism of differentially expressed proteins and related pathways identified by proteomics needs to be evaluated in the future. Because goats are twin-bearing animals, nutrient management during pregnancy is crucial and further knowledge of the effects of feed restriction will enrich the potential for fetal programming in mammals.
Acknowledgements
This work was jointly supported by the National Natural Science Foundation of China (grant no. 31402105, 31760678), CAS Visiting Professorship for Senior &Young International Scientists (grant no. 2013Y2GA0010, 2017VBA0026) and Youth Innovation Team Project of ISA, CAS (2017QNCXTD–ZCS). The supporting agency had no role in the design or conduct of the study; collection, analysis or interpretation of the data; or the preparation and approval of the manuscript.
Q. Y. and Z. T. designed the study. J. X. was in charge of data collection. X. W., D. S. and Q. Y. analysed the data and wrote the paper. All authors were involved in the interpretation of the data, critically reviewed the manuscript and approved the final version. Q. Y. and Z. T. were responsible for the manuscript’s contents.
None of the authors has any conflicts of interest to declare.