INTRODUCTION
Marine fish species use different reproductive tactics to reduce mortality during their early life stages. Among the tactics employed, spawning may occur at mid depths at the shelf-break during upwelling events, increasing the chances of egg and larval onshore transport (e.g. hakes, Grote et al., Reference Grote, Stenevik, Ekau, Verheye, Lipiński and Hagen2012; Landaeta & Castro, Reference Landaeta and Castro2012). Small pelagic species, such as anchovies and sardines, spawn in surface waters during the austral winter, when the Coriolis effect is driven by northern winds increasing coastal retention (Castro et al., Reference Castro, Salinas and Hernández2000; Cubillos et al., Reference Cubillos, Ruiz, Claramunt, Gacitúa, Núñez, Castro, Riquelme, Alarcón, Oyarzún and Sepúlveda2007). Subsequently, postlarvae that display diel vertical migrations also retain individuals in coastal areas (Parada et al., Reference Parada, Mullon, Roy, Freón, Hutchings and van der Lingen2008; Landaeta & Castro, Reference Landaeta and Castro2013). Rocky intertidal and subtidal species, such as clingfish and triplefin, hatch from benthic egg masses per lunar phase (Contreras et al., Reference Contreras, Landaeta, Plaza, Ojeda and Bustos2013; Palacios-Fuentes et al., Reference Palacios-Fuentes, Landaeta, Jahnsen-Guzmán, Plaza and Ojeda2014) and their larvae are retained in nearshore waters by poorly understood oceanographic processes (Hernández-Miranda et al., Reference Hernández-Miranda, Palma and Ojeda2003; Landaeta et al., Reference Landaeta, Zavala-Muñoz, Palacios-Fuentes, Bustos, Alvarado-Niño, Letelier, Cáceres and Muñoz2015). Marine fish species inhabiting sandy bottoms utilize windward coastal areas as nursery grounds, where breaking waves may disturb large predators and food resources are abundant (Pattrick & Strydom, Reference Pattrick and Strydom2014).
Nearshore environments off central Chile are characterized by a well-mixed water column and spatial homogeneity in autumn-winter and the onset of a thermocline in spring and summer, when winds influence upwelling and sea breezes intensify (Hernández-Miranda et al., Reference Hernández-Miranda, Palma and Ojeda2003; Narváez et al., Reference Narváez, Poulin, Leiva, Hernández, Castilla and Navarrete2004), leading to an increase in chlorophyll-a concentration and invertebrate settlement during spring-summer (Navarrete et al., Reference Navarrete, Broitman, Wieter, Finke, Venegas and Sotomayor2002; Wieters et al., Reference Wieters, Kaplan, Navarrete, Sotomayor, Largier, Nielsen and Véliz2003). Environmental forcing varies at a seasonal scale in coastal marine areas (Huyer, Reference Huyer1983; Mittelstaedt, Reference Mittelstaedt1991), thus it is expected that fish eggs and larvae will be subject to variability in their early life traits (ELT), as an adaptive response to the environment. For example, hake, Merluccius gayi Guichenot, 1848, change their spawning location, egg size and the vertical migration pattern of their larvae between the main (spring) and secondary (early autumn) reproductive seasons (Landaeta & Castro, Reference Landaeta and Castro2012). Among ELT, hatching patterns, growth and survival have crucial importance in determining the recruitment level of year classes in fish populations (Watanabe et al., Reference Watanabe, Ochiai and Fukamichi2014) and may vary at differing temporal and spatial scales (Plaza et al., Reference Plaza, Katayama and Omori2003; Rodríguez-Valentino et al., Reference Rodríguez-Valentino, Landaeta, Castillo-Hidalgo, Bustos, Plaza and Ojeda2015). Additionally, developmental instabilities (i.e. the failure of an individual to produce a consistent phenotype in a given environment; Zakharov & Graham, Reference Zakharov and Graham1992) may also occur, thereby affecting ELT of marine fishes (Somarakis et al., Reference Somarakis, Kostikas, Peristeraki and Tsimenides1997; Lemberget & McCormick, Reference Lemberget and McCormick2009).
Otoliths represent a practical tool for studying ELT in marine fishes and developmental instability through bilateral symmetry (Palmer & Strobeck, Reference Palmer and Strobeck1986). Otoliths are hard calcium carbonate structures and constitute an integral part of the hearing and equilibrium components in fishes (Gagliano et al., Reference Gagliano, Depczynski, Simpson and Moore2008). Through diel variations in calcium and protein deposition, bipartite structures known as daily growth increments often form at the microstructural level of the otolith (Campana, Reference Campana1984); therefore, otolith microstructure examination may be used to determine growth rates and survival of fish larvae (Brothers et al., Reference Brothers, Mathews and Lasker1976; Bustos et al., Reference Bustos, Landaeta, Palacios-Fuentes, Jahnsen-Guzmán and Balbontín2015). Because otoliths are paired structures, they also offer an opportunity to measure departures from perfect symmetry, especially in fish larvae, since their growth rates are faster, their capability to tolerate stress is lower, and the link between growth performance and fitness is stronger (Zenteno et al., Reference Zenteno, Bustos and Landaeta2014; Díaz-Gil et al., Reference Díaz-Gil, Palmer, Catalán, Alós, Fuiman, García, Gil, Grau, Kang, Maneja, Mohan, Morro, Schaffler, Buttay, Riera-Batle, Tolosa and Morales-Nin2015).
Early life stages of sand-dwelling fishes from eastern boundary currents have been scarcely studied until now, and include the family Dactyloscopidae, known as sand stargazers. The Chilean sand stargazer Sindoscopus australis (Fowler & Bean 1923) is the only dactyloscopid that inhabits cold temperate waters and is known only from coastal waters of Chile at about 23°–36°S where it is found at depths of 2 m or less (Dawson, Reference Dawson1977). There is no information about how many spawning seasons this stargazer has. Adults display paternal care of egg clutches, behind pectoral fins, during embryonic development. Larvae are pelagic, occur in nearshore waters, and grow at slow rates (0.09–0.21 mm day−1), with significant interannual variations (Herrera et al., Reference Herrera, Llanos-Rivera and Landaeta2007; Rodríguez-Valentino et al., Reference Rodríguez-Valentino, Landaeta, Castillo-Hidalgo, Bustos, Plaza and Ojeda2015). Nonetheless, there is no information about variability of the ELT of this species at a seasonal scale.
Therefore, the objective of this study was to compare the seasonal variation (winter vs spring spawned) in the early life traits of Sindoscopus australis, a sand-dwelling fish inhabiting a coastal area of central Chile, using sagittal otolith microstructure and size. It was expected that ELT would vary at seasonal scale, with faster growth rates and fewer developmental instabilities for those larvae hatched during the spring season, when seawater temperature is higher.
MATERIALS AND METHODS
Fieldwork
From mid austral autumn (8 May) to spring (17 December) 2013, 10 nearshore (<500 m offshore) night-time surveys were conducted at El Quisco Bay (EQB, 33°24′S 71°43′W), central Chile, on board an artisanal vessel (Table 1). For each sampling date, eight repetitions of oblique hauls of a Bongo net (60 cm diameter, 300 µm mesh size) with one TSK flowmeter mounted in the frame of the net were performed for 15–20 min from a depth of 20 m to surface, in a zone with maximum depth of 30 m. Seawater volumes filtered by the net ranged from 37.3 to 1453.1 m3 (mean ± 1SD: 142.8 ± 176.1 m3). Subsequently, the nets were washed on board and all zooplankton samples (N = 160) were initially fixed with 5% formalin buffered with sodium borate and preserved in 96% ethanol after 12 h. This procedure has no effect on the microstructure of the otolith (Palacios-Fuentes et al., Reference Palacios-Fuentes, Landaeta, Muñoz, Plaza and Ojeda2012, Reference Palacios-Fuentes, Landaeta, Jahnsen-Guzmán, Plaza and Ojeda2014; Contreras et al., Reference Contreras, Landaeta, Plaza, Ojeda and Bustos2013).
Table 1. Summary of the sampling dates off El Quisco Bay, central Chile.

SD, standard deviation. Abundances expressed as ind. 1000 m−3.
Laboratory work
In the laboratory, all larval fish were separated, counted and identified into the lowest possible taxon. Identification of the sand stargazer larvae, Sindoscopus australis, was based on the criteria described by Herrera et al. (Reference Herrera, Llanos-Rivera and Landaeta2007). Larval abundance was standardized to individuals (ind.) 1000 m−3 using the flowmeter counts (Table 1). The standard length (from the tip of the snout to the base of the hypural bones, SL) was measured to the nearest 0.01 mm using an Olympus SZ-61 stereomicroscope with a Moticam 2500 (5.0 M Pixel) video camera connected to a PC with the Moticam Image Plus 2.0 software. No corrections for shrinkage were carried out on the larval measurements.
The left and right sagittae otoliths were removed from 296 larvae (4.28–18.21 mm SL, Nwinter = 180; Nspring = 116; Figure 1) using dissecting needles. No otoliths were broken during the process. The otoliths were embedded in epoxy resin on a glass slide. The daily age was estimated by counting the number of otolith increments with a Motic BA310 light microscope at 1000× magnification under oil immersion. The longest radius of a sagitta was measured three times and its mean was used for further analysis. The perimeters and areas of the otoliths were then measured once using the Moticam Image Plus 2.0 software.
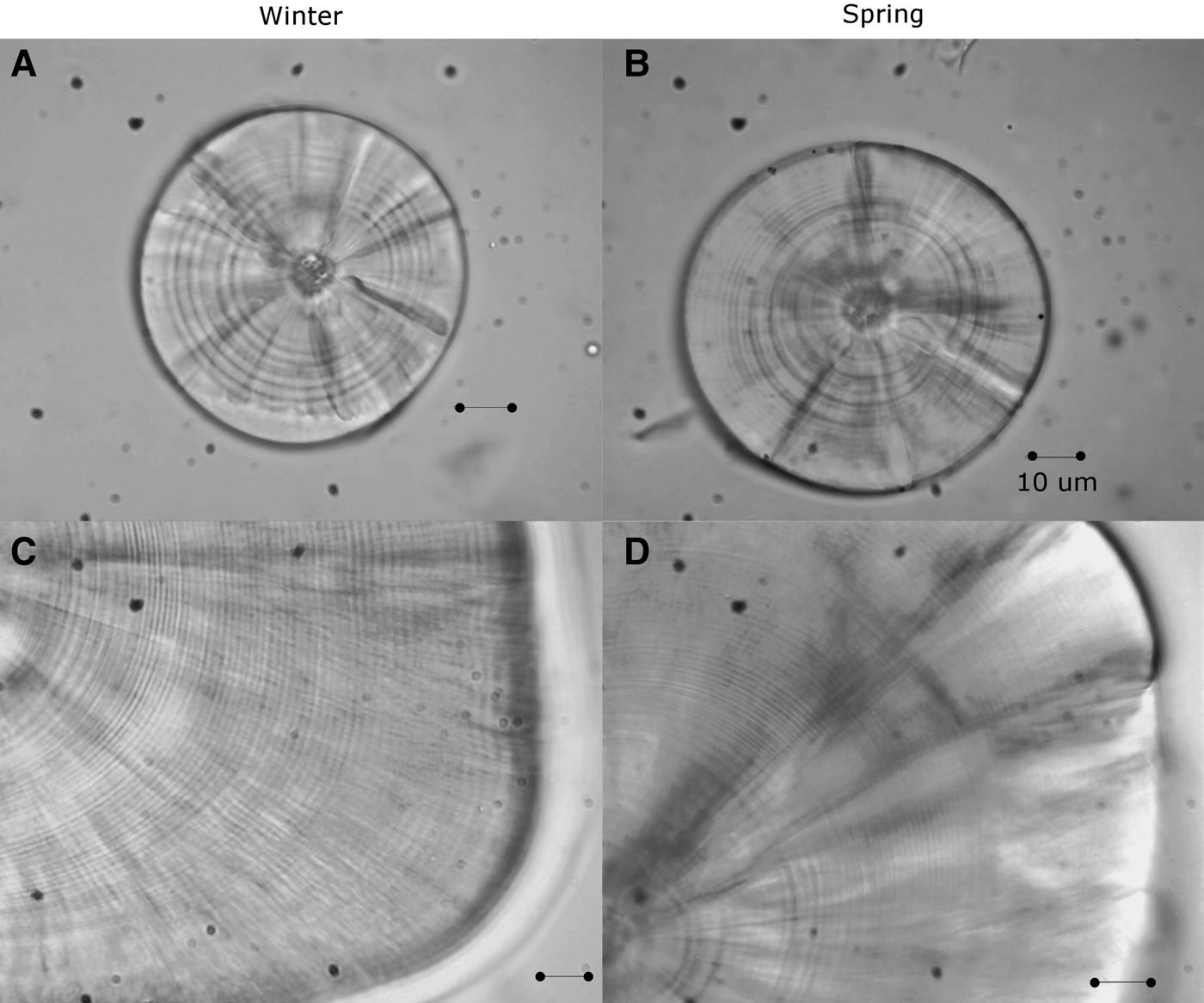
Fig. 1. Sagittal otoliths of larvae of sand stargazer Sindoscopus australis. (A) Larvae of 4.53 mm SL and 9 microincrements; (B) larvae of 4.28 mm SL and 10 microincrements; (C) larvae of 15.89 mm SL and 51 microincrements; (D) larvae of 18.21 mm SL and 68 microincrements. All bars correspond to 10 µm.
Three independent counts were performed on the right and left sagittae. The counts were performed after a prominent hatch mark. When the coefficient of variation (CV = standard deviation/mean × 100) of the increment counts among the 3 readings was <10%, the mode of the three counts was calculated and utilized for the analysis. When CV was >10%, the otolith reading was discarded (Nwinter = 4; Nspring = 1). Once selection of the values was done, a comparison of readings was carried out using a Wilcoxon matched pairs test, testing the hypothesis that the reading of the left sagitta and right sagitta did not differ. The daily periodicity of the growth increments for Sindoscopus australis or other Dactyloscopidae has not been validated; however, several species of the suborder Blennioidei have been validated for daily periodicity in coastal waters of central Chile and elsewhere (Hernández-Miranda et al., Reference Hernández-Miranda, Veas, Espinoza, Thorrold and Ojeda2009; Kohn & Clements, Reference Kohn and Clements2011; Mansur et al., Reference Mansur, Catalán, Plaza, Landaeta and Ojeda2013).
Data analysis
Significant variations in the standardized larval abundance among sampling days and between winter (July–August 2013) and spring (October–December 2013) were compared utilizing a Kruskal–Wallis H test, because data were not normally distributed (Shapiro–Wilk test, W = 0.72, P < 0.001), followed by post-hoc analyses for pairwise comparisons of periods.
Simple linear regressions by least-squares (SL = a + bA) between the micro-increment counts (age, A) and larval lengths (SL) were adjusted, separately for winter and spring dates. In this case, the slope corresponded to the population growth rate, and the intercept corresponded to the estimated hatch size. To compare the population growth rate between seasons, slopes were compared with one-way ANCOVA (Zar, Reference Zar2010). Also, a Gompertz growth model was fitted, with w = W inf e−ke−gt, where W inf = asymptotic size = w 0ek, k = a adimensional parameter, w 0 = size at hatch, and g = instantaneous growth rate at t = t 0. Linear and Gompertz models were compared using the Akaike Information Criterion (AIC).
The hatching days were back-calculated and related to the lunar cycle. To compare distribution patterns, hatch day frequencies for each season were converted to Julian days and then converted to angles (°) by dividing by 365 and then multiplying by 360°, so data could be analysed using circular statistics. Similarly, for each sampling date, the days since new moon (DNM) were counted, and thereby assigned DNM values from 0 to 29 for each date, in which 0 represented the new moon. DNM values were converted to angles (°) by dividing by 29 (the length, in days, of the lunar cycle) and then multiplying by 360°. To assess whether the hatching events showed lunar periodicity, the data were analysed using the Rao's spacing test (Batschelet, Reference Batschelet1981); the null hypothesis that the hatching events would be equally or randomly spaced throughout the lunar cycle was tested for each data set.
The symmetry of sagitta otoliths was analysed following Palmer & Strobeck (Reference Palmer and Strobeck1986), using radius, perimeter and area as morphological traits. To avoid the effect of the trait size on the asymmetry, index R − L/[(R + L)/2] was calculated for each otolith trait. Subsequently, skewness and kurtosis, and the variance (F test) were compared among winter and spring cohorts, and tests for normality were applied as done previously (Shapiro–Wilk test). P < 0.05 was considered significant.
RESULTS
Larval abundance and size distribution during winter and spring 2013
During 2013, larval abundance of Sindoscopus australis varied from 2.06 to 259.28 ind. 1000 m−3 (mean ± SD and median, respectively: 46.01 ± 50.58 ind. 1000 m−3, 25.57 ind. 1000 m−3). Larvae were totally absent in the plankton samples during May and June 2013, and then appeared and increased their abundance to reach the maximum value during October, thereafter diminishing until December (Table 1). There were significant differences in larval abundance among samplings (Kruskal–Wallis test, H = 20.36, P = 0.004), with greater larval abundance in late October than in early August (Table 1). However, larval abundances for winter and spring cohorts were similar (Mann–Whitney U test, U = 748, P = 0.245).
Size distribution of larvae from austral winter and spring were not normally distributed (winter: W = 0.86; P < 0.001; spring: W = 0.955; P < 0.001); larvae collected during winter (range: 4.35–15.89 mm SL) were significantly smaller than those collected in spring (range: 4.28–18.21) (Mann–Whitney test, U = 6761; P < 0.001) (Figure 2).

Fig. 2. Standard length distribution of larval Sindoscopus australis collected during austral winter and spring 2013.
Larval growth and size-at-age variations
For larvae collected during winter, otolith readings differed between right and left sagittae (Wilcoxon paired test, W = 1072.5, P = 0.02); therefore, only right sagittae were used for further growth analysis.
The parameters of the linear and Gompertz models are given in Table 2. During austral winter, based on simple linear models, the estimated growth rate for larval Sindoscopus australis was 0.145 mm day−1 ± 0.008, while for spring it increased significantly to 0.182 mm day−1 ± 0.008 (homogeneity of slopes test, F = 14.96; P < 0.001; Figure 3). Both linear and Gompertz models estimated hatch sizes that differed between winter (linear = 4.13 mm; Gompertz = 4.73 mm) and spring (linear = 2.99 mm; Gompertz = 2.92 mm). Nonetheless, size-at-age of larvae compared using linear models was similar for both cohorts (winter = 9.09 mm SL; spring = 9.03 mm SL) (one-way ANCOVA, F = 0.15; P = 0.69).
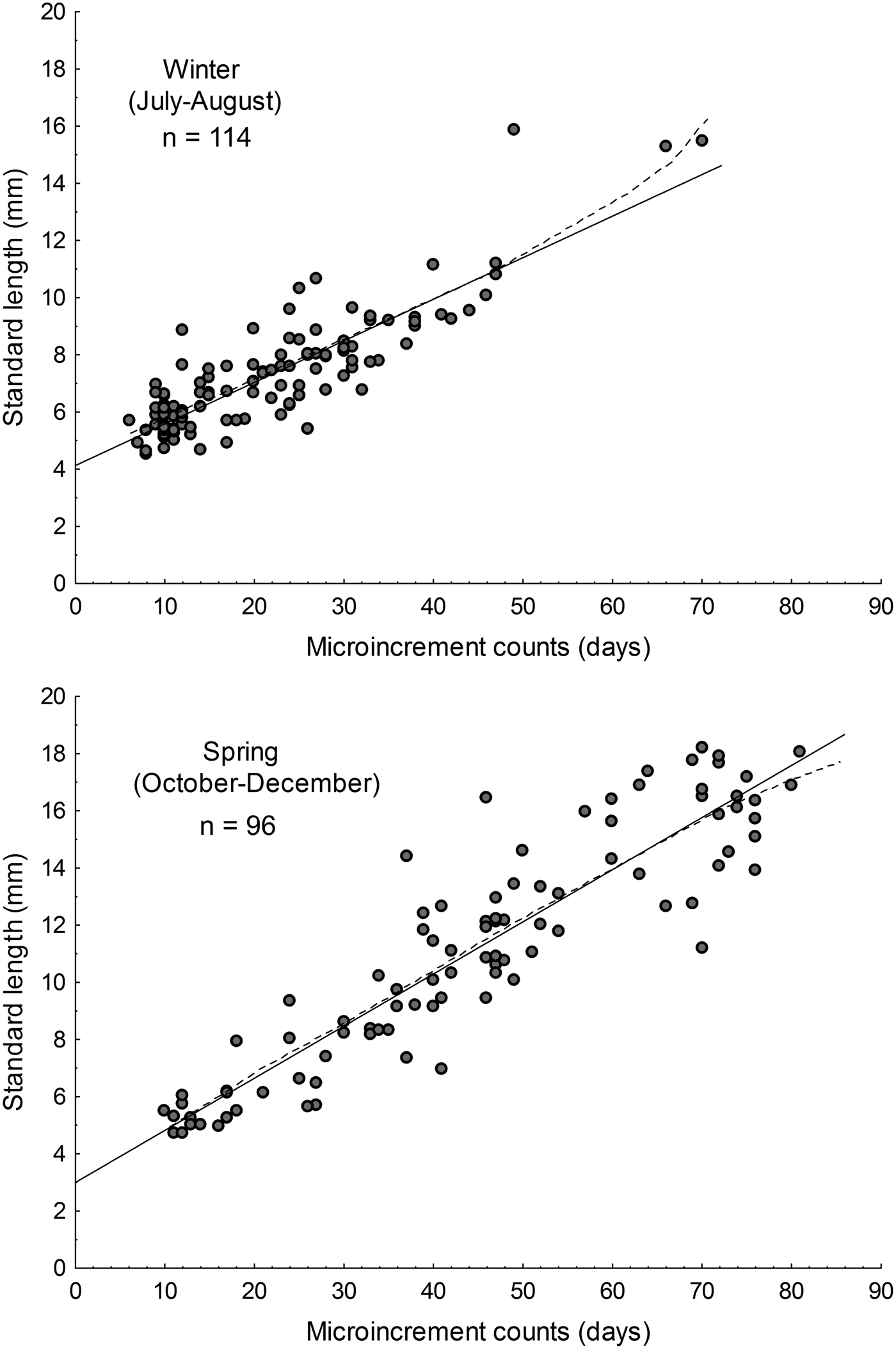
Fig. 3. Larval growth rates of Sindoscopus australis estimated by sagittal microstructure analysis, during (A) winter and (B) spring. Linear regression models showed in solid lines and Gompertz models showed in dotted lines.
Table 2. Parameters of the linear and Gompertz growth models for larval Sindoscopus australis.

AIC, Akaike Information Criterion.
Back-calculated hatch days and lunar cycle
Back-calculated hatch days during austral winter were mostly during neap tides, at first or third quarter moon (Figure 4). Moreover, during austral spring most of the hatch period occurred during spring tides, particularly at new moon (Figure 4). Circular statistics indicated that hatching did not follow a uniform distribution throughout the lunar cycle, either for winter (Rao's U = 268.8, P < 0.0001) or for spring (U = 262.8, P < 0.0001).

Fig. 4. Back calculated lunar hatching patterns of Sindoscopus australis off central Chile during (A) winter and (B) spring.
Otolith bilateral symmetry
Sagittal radius showed a leptokurtic distribution during winter and spring season, while the other traits were platykurtic (Table 3, Figure 5). Only the symmetry of perimeter of sagitta otoliths showed normal distribution for winter and spring seasons (winter, Shapiro–Wilk's test, P = 0.576; spring, P = 0.382) and no skewness during winter (Table 3). Because skewness of the traits was positive or zero and negative for winter-spawned and spring-spawned larvae, respectively (Table 3), a genetic basis for directional asymmetry is doubtful, and skewness was caused by exceptional, asymmetric otoliths. When comparing perimeter asymmetry between seasons, winter-spawned individuals showed more variance (0.001) than those from spring-spawned larvae (variance = 0.0006) (F-test, F = 1.505, P = 0.038); therefore, larvae from winter experienced more fluctuating asymmetry than those from spring.
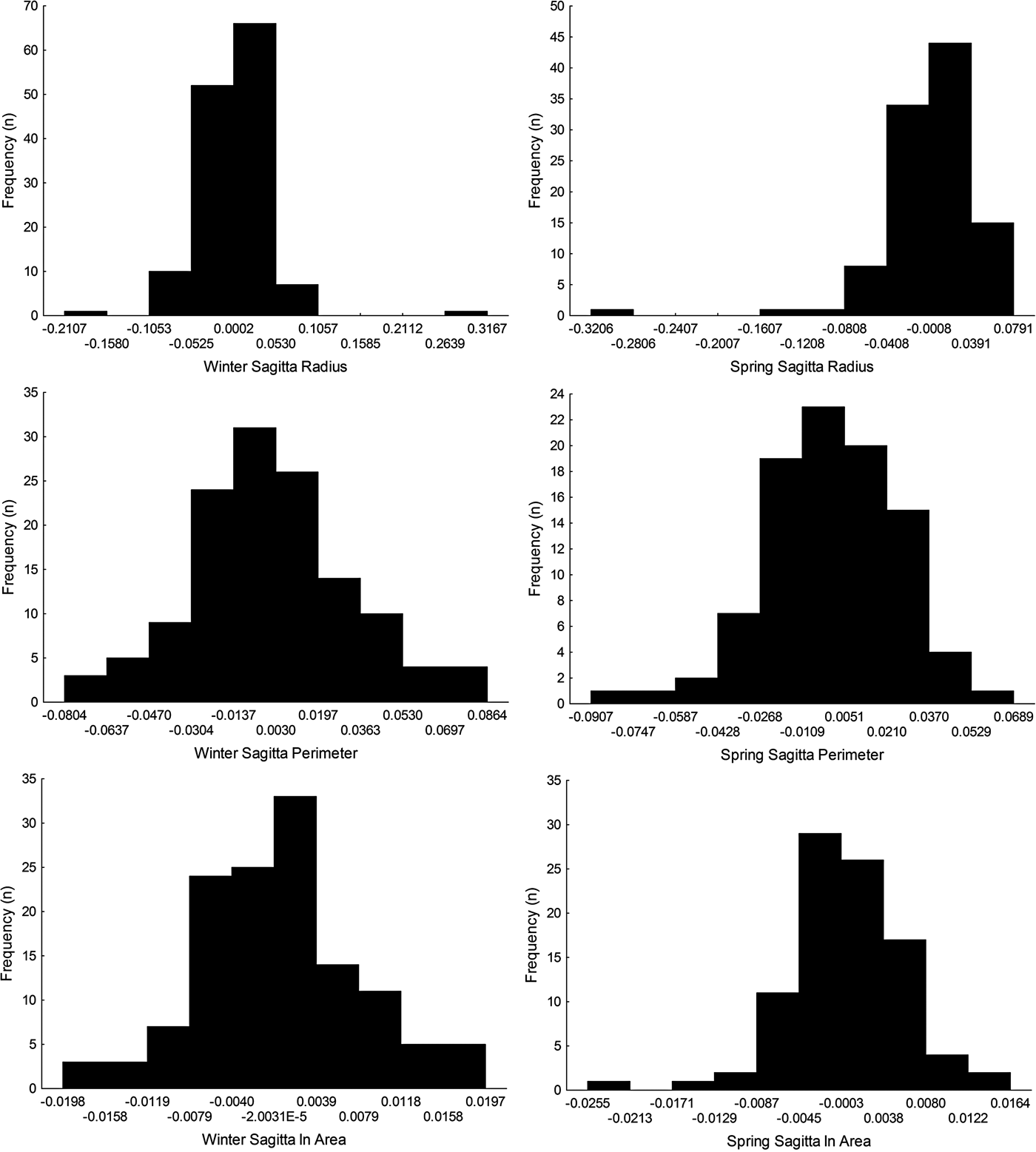
Fig. 5. Left-right symmetry of different otolith traits (radius, perimeter and area) for winter-spawned and spring-spawned cohorts of Sindoscopus australis.
Table 3. Otolith symmetry traits by seasonal cohort.

SE, standard error.
DISCUSSION
Several differences arose in the early life traits of the sea stargazer, Sindoscopus australis, hatched during the austral winter and spring seasons off central Chile. Larval size and growth rates were higher during spring than in winter, but size-at-hatch was smaller for the spring cohort. Hatching pattern also changed from occurring during first and third quarter (i.e. neap tides) during winter to happening during the new moon (spring tides). Finally, only the perimeter of the sagittal otolith showed fluctuating asymmetry, with winter exhibiting more variance than did spring.
Temporal variation of the early life traits may be because of the main environmental mesoscale process occurring along Chile: upwelling events. During austral winter, north winds dominate the area, producing an Ekman layer moving onshore, and retaining early life stages of marine fishes, such as anchovies and sardines (Castro et al., Reference Castro, Salinas and Hernández2000; Cubillos et al., Reference Cubillos, Ruiz, Claramunt, Gacitúa, Núñez, Castro, Riquelme, Alarcón, Oyarzún and Sepúlveda2007). Food availability, i.e. phytoplankton and microzooplankton are less abundant then, but it enables adequate feeding for shorefish larvae (Llanos et al., Reference Llanos, Herrera and Bernal1996; Llanos-Rivera et al., Reference Llanos-Rivera, Herrera and Bernal2004). Seawater temperature is also lower during winter time off central Chile (12.7°C in July off El Quisco, Narváez et al., Reference Narváez, Poulin, Leiva, Hernández, Castilla and Navarrete2004), but increases rapidly during spring and summer (14.5°C in November, Hernández-Miranda et al., Reference Hernández-Miranda, Palma and Ojeda2003; Narváez et al., Reference Narváez, Poulin, Leiva, Hernández, Castilla and Navarrete2004). Recently, Tapia et al. (Reference Tapia, Largier, Castillo, Wieters and Navarrete2015) recorded the sea temperature at El Quisco as 13.02 ± 1.22°C (mean ± SD), varying between 10.93 (July) and 18.12°C (February).
A seasonal increase in sea temperature may trigger an increase in the growth rate experienced by fish larvae, and also increases swimming capabilities and larval duration (Amara et al., Reference Amara, Desaunay and Lagardere1994; Johannessen et al., Reference Johannessen, Blom and Folkvord2000; Green & Fisher, Reference Green and Fisher2004). However, there are species-specific responses to seasonal habitat temperature variations; while round herring Etrumeus teres have a positive correlation between growth rate and habitat temperature, growth rate of sardines, Sardinops melanostictus, in the same area is independent of the seawater temperature (Watanabe et al., Reference Watanabe, Ochiai and Fukamichi2014). Furthermore, seasonal variations in larval growth history may be independent of temperature, as in the coral reef fish, Thalassoma bifasciatum (Searcy & Sponaugle, Reference Searcy and Sponaugle2000). In this study, Sindoscopus australis displayed variations in its growth rate between winter-hatched and spring-hatched larvae, but the environmental reasons for this remain unknown.
Variations in the size-at-hatch and size-at-age estimated by growth models indicate that seasonal variations within populations may be caused by a parental effect on the egg size-offspring fitness function (Einum & Fleming, Reference Einum and Fleming2002). Intrapopulation variation in egg size is larger in fish with demersal eggs and larvae, such as the sand stargazer, than in those with pelagic eggs and/or larvae; selection for variable egg sizes within populations should exist if maternal (or parental) phenotype can influence offspring environment, as is likely the case in species with demersal eggs and larvae (Einum & Fleming, Reference Einum and Fleming2002), and probably in adult S. australis.
Many marine organisms may synchronize the release of gametes or larvae with respect to photoperiod, tides, tidal amplitude or lunar cycles (Morgan & Christy, Reference Morgan and Christy1994). The synchrony of larval production with lunar cycle is an adaptation to maximize settlement of planktonic juveniles (Robertson et al., Reference Robertson, Petersen and Brawn1990). Nonetheless, Sindoscopus australis varied from having a hatching period synchronized to neap tides in winter to hatching events near new moon during spring. Hatching during neap tides during winter may increase larval retention in coastal areas, because of weaker tidal currents (Contreras et al., Reference Contreras, Landaeta, Plaza, Ojeda and Bustos2013). Reproductive synchrony at new moon during spring might be beneficial because hatched larvae encounter favourable tides for transport away from rocky reef-based predators (Foster, Reference Foster1987). The timing of larval release seems to be plastic at several timescales (Plaza et al., Reference Plaza, Katayama and Omori2003; Rodríguez-Valentino et al., Reference Rodríguez-Valentino, Landaeta, Castillo-Hidalgo, Bustos, Plaza and Ojeda2015; this study) and evolved because of predation on the adults, so eggs and larvae abundances not only vary between phases of each cycle, but also differ among cycles depending upon species-specific life history traits (Morgan & Christy, Reference Morgan and Christy1994).
Only the perimeter of the sagittae otoliths of Sindoscopus australis showed fluctuating asymmetry; other trait measurements departed from normality. Sagittal perimeter showed larger variance during winter compared with spring. However, inconsistencies in the response of the asymmetry among otolith traits (radius, perimeter, area) does not imply a higher developmental instability, or lower fitness, during the winter season (Díaz-Gil et al., Reference Díaz-Gil, Palmer, Catalán, Alós, Fuiman, García, Gil, Grau, Kang, Maneja, Mohan, Morro, Schaffler, Buttay, Riera-Batle, Tolosa and Morales-Nin2015). A non-normal distribution of right minus left measurements of otolith traits could be caused by the presence of some outliers (i.e. otoliths with two primordia). Also, the eyes of Dactylopscopidae shift from lateral to dorsolateral position (Watson, Reference Watson, Patzner, Gonçalves, Hastings and Kapoor2009), probably causing changes in the shape of the sagitta otoliths located in the otic capsules. During transformation, otoliths of several marine fish species develop accessory growth centres (AGC) (Jearld et al., Reference Jearld, Sass and Davis1993; Brown et al., Reference Brown, Busby and Mier2001), which may increase the asymmetry between otoliths. Moreover, the evidence for directional asymmetry in radius and area is weak, because skewness direction varied between seasons, suggesting a lack of genetic basis for this variability (Graham et al., Reference Graham, Freeman and Emlen1993).
Therefore, the Chilean sand stargazer displays differences in its ELT in response to individual hatching times. Larger larvae are initially hatched during winter at neap tides, and experienced slower growth rates and greater developmental instabilities; while during spring, hatching occurred during spring tides, especially around new moon, producing small larvae with faster growth rates. These trade-offs may be adaptations to increased chances of survival and settlement in sandy bottoms during periods of contrasting environmental conditions in nearshore waters.
ACKNOWLEDGEMENTS
We want to thank the field support of Pámela Palacios-Fuentes, Jorge E. Contreras and Francisca Zavala-Muñoz on board the artisanal vessel at El Quisco Bay, central Chile. Comments of two anonymous reviewers improved an early version of the manuscript.
FINANCIAL SUPPORT
The samples utilized during this research were obtained by a grant FONDECYT 1120868, adjudicated to Dr Gabriela Muñoz (Universidad de Valparaíso) as PI and MFL as co-I. GCH was partially funded by grants CIMAR C20F 14-09 and FONDECYT 1150296, adjudicated to MFL as PI.