Introduction
Orthopoxviruses are a genus of viruses that include variola, vaccinia, cowpox, and monkeypox (mpox) viruses. Smallpox, a highly pathogenic orthopoxvirus, is estimated to have claimed the lives of over 300 million people worldwide but was successfully eradicated in 1977 through an international vaccine campaign led by the World Health Organization (WHO). Mpox is endemic to multiple African countries, including Benin, Cameroon, the Central African Republic, the Democratic Republic of the Congo, Gabon, Ivory Coast, Liberia, Nigeria, the Republic of the Congo, Sierra Leone, and South Sudan [1].
Historically, the transmission of mpox in non-endemic regions has been short-lived and contained within a specific geographic area [Reference Sklenovská and Van Ranst2]. However, the increased prevalence of mpox in humans since the 1980s has been linked to a decrease in vaccine immunity and an increase in viral fitness traits, making it a significant emerging human threat [Reference Sklenovská and Van Ranst2]. In 2022, the World Health Organization (WHO) reported multiple international mpox outbreaks in 20 non-endemic European countries, as well as the United States of America, Canada, Mexico, and much of South America [3]. From May to June 2022, these outbreaks resulted in a total of 780 cases [4]. As of 28 July 2022, the Centers for Disease Control and Prevention (CDC) reported 4907 confirmed cases in the United States, with the total cumulative cases in non-endemic countries exceeding 20,800. By December of 2022, the total reported cumulative cases in non-endemic countries surpassed 80,000 [4]. Figure 1 is a heatmap of global cumulative case counts for the 2022 epidemic as of 17 November 2022. We also include a heatmap of case counts normalized by total country population, shown in Figure 2. In June of 2022, the emergence of mpox in non-endemic countries led the WHO to declare the overall risk of further transmission as ‘moderate’ globally and ‘high’ in the European region. It was hypothesized that mpox mutated to find a new niche in tightly connected sexual networks [Reference Endo, Murayama, Abbott, Ratnayake, Pearson, Edmunds, Fearon and Funk5]. As such, mpox now presents a significant public health threat to non-endemic regions, with some countries, such as the United Kingdom, responding by purchasing large amounts of smallpox vaccines for public distribution.

Figure 1. Cumulative mpox cases for the 2022 epidemic from 1 January 2022, through 17 November 2022. Heatmap constructed from publicly available WHO data (ref. [3], accessed 17 November 2022).

Figure 2. Cumulative mpox cases for the 2022 epidemic from 1 January 2022 through 17 November 2022, normalized by country total population. Heatmap constructed from publicly available WHO data (ref. [3], accessed 17 November 2022). Country population data accessed from WolframAlpha Knowledgebase on 29 November 2022.
Mathematical modelling has proven to be a valuable tool for understanding epidemics and developing intervention strategies [Reference Thompson6, Reference Ciupe and Heffernan7]. Modelling of in-host pathogen dynamics has been critical in advancing our understanding of many pathogens, including HIV, HCV, HBV, HSV, influenza, pneumococcus, and SARS-CoV-2, and has also aided the development of vaccine therapies [Reference Perelson and Ribeiro8–Reference Korosec, Farhang-Sardroodi, Dick, Gholami, Ghaemi, Moyles, Craig, Ooi and Heffernan16]. This review provides an in-depth examination of the current epidemiological understanding of mpox from a modelling perspective, and investigates how modelling studies contribute to mechanistic insight into viral fitness and transmission traits. In Section 2, we briefly cover the history and origins of mpox, and in Section 3, we provide an overview of the current basic knowledge of biology and clinical presentation of human mpox. In Section 4, we critique and review population-level modelling studies, differentiating between studies focused on endemic and non-endemic regions, those considering prior immunity from smallpox vaccines, and animal models. We summarize both pre- and post-2022 modelling parameters, including the reproduction number, force of infection, incubation, and recovery rates, in Table 1.
Table 1. Table of values listing epidemiological parameters for mpox viral dynamics from the literature

a These values are 2022 epidemic specific; all other values are determined from pre-2022 mpox outbreaks.
History and ecology of Mpox
A pox-like disease was first reported in 1959 in cynomolgus monkeys and was thus named ‘monkeypox’ [Reference von Magnus, Andersen, Petersen and Birch-Andersen17]. The disease was found to have similar structural features as orthopoxviruses: rectangular with diameter 200–250 μm [Reference von Magnus, Andersen, Petersen and Birch-Andersen17]. It was also observed to present similarly to variola-vaccinia viruses and exhibited a similar serological relationship [Reference von Magnus, Andersen, Petersen and Birch-Andersen17]. Further studies revealed that mpox led to the formation of intracytoplasmic eosinophilic inclusions (small whitish lesions) and could pass serially in rabbit skin [Reference Arita and Henderson18]. Throughout the 1960s and 1970s, WHO continued to monitor both mpox and smallpox in non-human primates to determine if an animal reservoir existed. In the 1960s, four mpox outbreaks were recorded in animals with no recorded infections in humans [Reference Arita and Henderson18–Reference Peters20]. In 1966, an mpox outbreak occurred in a zoo and was believed to have been caused by two imported anteaters. The 1966 zoo outbreak had a particularly high mortality rate. Despite containment procedures, mpox spread to nearby enclosures, resulting in 23 animal infections and a total of 11 deaths, including 6 out of 10 infected orangutans [Reference Peters20].
Transmission between humans
The first human mpox case was reported in 1970 in a 9-month-old baby in the Democratic Republic of Congo [Reference Breman, Ruti and Steniowski21]. A study of 155 mpox cases in west and central Africa from 1970–1983 estimated only 20% of cases to spread from human-to-human contact, where human mpox cases were primarily suspected to occur from contact with monkeys and squirrels [Reference Arita, Jezek, Khodakevich and Ruti22]. The human-to-human transmission was noted to ‘stop spontaneously’, with attack rates suspected to be 15% amongst smallpox-unvaccinated households and 0.4% amongst vaccinated [Reference Arita, Jezek, Khodakevich and Ruti22]. These were noted to be comparably less than smallpox attack rates amongst the unvaccinated which ranged from 33% to 88% [Reference Rao, Jacob, Kamalakshi and Appaswamy23–Reference Mukherjee, Sarkar and Mitra25]. A study conducted in Zaire between 1980 and 1984 of 214 patients with human mpox found attack rates for household contacts of 7.2% amongst unvaccinated and 0.9% amongst vaccinated [Reference Jezek, Marennikova, Mutumbo, Nakano, Paluku and Szczeniowski26]. In this study, 13% of cases were found amongst vaccinated individuals leading to the hypothesis that the immunity gained from smallpox vaccination was waning [Reference Jezek, Marennikova, Mutumbo, Nakano, Paluku and Szczeniowski26], and further raised a concern that the virus may later become endemic [Reference Arita, Jezek, Khodakevich and Ruti22].
The low attack rates of mpox, and the unchanging secondary attack rates throughout the 1970s through early 1980s amongst the unvaccinated, supported the decision from the Global Commission to cease the smallpox vaccination programme in Central African countries where mpox was now considered endemic [Reference Fine, Jezek, Grab and Dixon27]. Multiple self-contained mpox outbreaks were documented through the early 2000s. Of note, a major outbreak in Nigeria began in September of 2017 and ultimately led to 228 suspected cases [Reference Ogoina, Izibewule, Ogunleye, Ederiane, Anebonam, Neni, Oyeyemi, Etebu and Ihekweazu28]. Human mpox infections in the 2017 Nigeria outbreak were predominantly male, and the outbreak was ultimately contained [Reference Ogoina, Izibewule, Ogunleye, Ederiane, Anebonam, Neni, Oyeyemi, Etebu and Ihekweazu28]. The 2003 mpox outbreak in the USA appeared to be particularly severe in children, where a fifth of paediatric patients developed serious complications resulting in intensive medical intervention, with half of paediatric patients admitted to the ICU [Reference Huhn, Bauer, Yorita, Graham, Sejvar, Likos, Damon, Reynolds and Kuehnert29]. For a detailed review of all pre-2018 human mpox outbreaks, we refer to ref. [Reference Sklenovská and Van Ranst2]. The 2022 international emergence of human–human transmission of mpox in multiple non-endemic countries constitutes a significant shift in viral prevalence.
Pathogenesis, clinical presentation, and longitudinal within-host dynamics of mpox
The incubation period of human mpox can range from 5 to 21 days [Reference Lum, Torres-Ruesta, Tay, Lin, Lye, Rénia and Ng30], with a typical incubation period of 7–17 days, followed by a prodromal period of 1–4 days [Reference Wilson, Hughes, McCollum and Damon31]. Clinical characteristics of mpox are similar to those of smallpox: enlarged lymph nodes and a rash period that lasts 14–28 days. Distinct from smallpox, mpox often presents with cervical or inguinal lymphadenopathy, suggesting that the immune response to mpox differs from that of smallpox [Reference Wilson, Hughes, McCollum and Damon31]. A detailed list of clinical characteristics, including changes in mpox epidemiology as a function of time, is described in the article by Wilson et al. [Reference Wilson, Hughes, McCollum and Damon31].
A study on non-human primates longitudinally tracked viral shedding and cytokines from both intrabronchial exposure (i.b.) and intravenous inoculation (i.v.) of mpox [Reference Johnson, Dyall, Ragland, Huzella, Byrum, Jett, Claire, Smith, Paragas, Blaney and Jahrling32]. Through tracking mpox viral features over a 36-day window, they found that the time to mean day of lesion exposure increases as a function of decreasing mpox dosage. They further found peak viral load to vary significantly between nasal and oral swabs. Recent clinical human studies in France and Spain have longitudinally tracked cohorts of people over 14 and 57 days [Reference Palich, Burrel, Monsel, Nouchi, Bleibtreu, Seang, Bérot, Brin, Gavaud, Wakim, Godefroy, Fayçal, Tamzali, Grunemwald, Ohayon, Todesco, Leducq, Marot, Calvez, Marcelin and Pourcher33, Reference Suñer, Ubals, Tarín-Vicente, Mendoza, Alemany, Hernández-Rodríguez, Casañ, Descalzo, Ouchi, Marc, Rivero, Coll, Oller, Miguel Cabrera, Vall-Mayans, Dolores Folgueira, Ángeles Melendez, Agud-Dios, Gil-Cruz, Paris de Leon, Ramírez Marinero, Buhiichyk, Galván-Casas, Paredes, Prat, Sala Farre, Bonet-Simó, Farré, Ortiz-Romero, Clotet, García-Patos, Casabona, Guedj, Cardona, Blanco, Marks and Mitjà34]. These studies compare mpox viral load between HIV+ and HIV- individuals and find mpox cycle threshold (Ct) values to decrease significantly for both categories of individuals [Reference Palich, Burrel, Monsel, Nouchi, Bleibtreu, Seang, Bérot, Brin, Gavaud, Wakim, Godefroy, Fayçal, Tamzali, Grunemwald, Ohayon, Todesco, Leducq, Marot, Calvez, Marcelin and Pourcher33, Reference Suñer, Ubals, Tarín-Vicente, Mendoza, Alemany, Hernández-Rodríguez, Casañ, Descalzo, Ouchi, Marc, Rivero, Coll, Oller, Miguel Cabrera, Vall-Mayans, Dolores Folgueira, Ángeles Melendez, Agud-Dios, Gil-Cruz, Paris de Leon, Ramírez Marinero, Buhiichyk, Galván-Casas, Paredes, Prat, Sala Farre, Bonet-Simó, Farré, Ortiz-Romero, Clotet, García-Patos, Casabona, Guedj, Cardona, Blanco, Marks and Mitjà34], and further conclude transmission of mpox to primarily occur through direct body contact rather than through a respiratory route or bodily fluids [Reference Palich, Burrel, Monsel, Nouchi, Bleibtreu, Seang, Bérot, Brin, Gavaud, Wakim, Godefroy, Fayçal, Tamzali, Grunemwald, Ohayon, Todesco, Leducq, Marot, Calvez, Marcelin and Pourcher33].
Serological features that inform us about immune responses can also be used by within-host modelling studies to reveal mechanistic insight into viral traits as well as vaccine dynamics. Interferon-gamma (IFNg) is a cytokine known to play a pivotal role in host defence against pathogens [Reference McNab, Mayer-Barber, Sher, Wack and O’Garra35, Reference Whitmire, Eam, Benning and Whitton36], and is often used to model within-host inflammatory responses and infer cellular-mediated immunity [Reference Moyles, Korosec and Heffernan15, Reference Korosec, Farhang-Sardroodi, Dick, Gholami, Ghaemi, Moyles, Craig, Ooi and Heffernan16]. Immunity from smallpox vaccination has been shown to elicit IFNg, cytotoxic T cell, and neutralizing protein responses in humans that can last over 20 years [Reference Sivapalasingam, Kennedy, Borkowsky, Valentine, Zhan, Pazoles, Paolino, Ennis and Steigbigel37]. The inflammatory cytokine IFNg has been shown to play an important role in protection against mpox in mice, whereby inactivation of the IFNg receptor led to increased sensitivity to mpox [Reference Earl, Americo and Moss38]. Earl et al. [Reference Earl, Americo and Moss38] also report viral titres as a function of time in various major organs, where lungs were found to contain the highest PFU/g for all time points. They also track six cytokines, including IFNg and IL6, as a function of time after injection and find a strong IFNg response in BALB/c mice but not in other types of mice [Reference Earl, Americo and Moss38]. Interestingly, orthopoxvirus have been shown to suppress recognition of viruses by innate cells through suppressing IFN production [Reference Shchelkunov39]. Further, mpox has been found to suppress T-cell activation by triggering a state of T-cell non-responsiveness [Reference Hammarlund, Dasgupta, Pinilla, Norori, Früh and Slifka40]; thus, a within-host model of mpox should take into account CD4 and CD8 suppression dynamics. These longitudinal data serve as a useful starting point for a within-host modelling study of mpox and can be utilized to guide model predictive power and determine practical identifiability in estimated parameters. Lum et al. [Reference Lum, Torres-Ruesta, Tay, Lin, Lye, Rénia and Ng30] provide an in-depth review of the clinical immune features of mpox.
Mpox cross-protective immunity from the smallpox vaccine is known to occur [Reference Lum, Torres-Ruesta, Tay, Lin, Lye, Rénia and Ng30]. For example, prairie dogs vaccinated with the smallpox vaccine and then challenged with mpox were found to mount a significant humoral response. Further, vaccinated humans were found to mount strong cellular and humoral responses as shown in longitudinal data over a 32-day study period [Reference Townsend, Keckler, Patel, Davies, Felgner, Damon and Karem41]. However, longer-term studies find efficacy wanes at an approximate rate of 1.29%/yr [Reference Nguyen, Ajisegiri, Costantino, Chughtai and MacIntyre42]. We refer to Lum et al. [Reference Lum, Torres-Ruesta, Tay, Lin, Lye, Rénia and Ng30] for an in-depth review of mpox clinical immune features.
In the next sections, we review epidemiological modelling efforts of population spread of mpox, distinguishing between human–human, animal–animal, animal–human, and human–animal scenarios. We further cover modelling studies incorporating climate variables, therapeutic strategies (from smallpox vaccine waning and future vaccination outcomes), contact tracing, and isolation measures. Machine learning is emerging as a technology with demonstrated capability for early detection of mpox [Reference Thieme, Zheng, Machiraju, Sadee, Mittermaier, Gertler, Salinas, Srinivasan, Gyawali, Carrillo-Perez, Capodici, Uhlig, Habenicht, Löser, Kohler, Schuessler, Kaul, Gollrad, Ma, Lippert, Billick, Bogoch, Hernandez-Boussard, Geldsetzer and Gevaert43]. However, we do not provide a detailed review of the application of machine learning to mpox; such work can be found in refs. [Reference Chadaga, Prabhu, Sampathila, Nireshwalya, Katta, Tan and Acharya44, Reference Jaradat, Al Mamlook, Almakayeel, Alharbe, Almuflih, Nasayreh, Gharaibeh, Gharaibeh, Gharaibeh and Bzizi45].
Population-level epidemiological models
SIR/SEIR with no immunity
Compartmental modelling techniques have been used extensively to describe the population spread of infectious diseases. Among infectious disease models, the most fundamental and classic model is the Susceptible-Infected-Recovered (SIR) compartmental model developed by Kermack and McKendrick [Reference Kermack and McKendrick46]. In the SIR model, the total population is divided into three subgroups based on the disease status: susceptible (S), infected (I), and recovered (R). S represents the susceptible population that has not yet but may be infected by the disease, I represents the infected population that can transmit the disease, and R represents the population recovered from the infected disease. Two parameters are used in the classic SIR model: the effective contact rate (β) and the recovery rate (γ). β affects the transition from S → I, and γ affects the transition from I → R, and the total population, N, is conserved through time N(t) = S(t) + I(t) + R(t). An example schematic of the SIR model is shown in Figure 4a.
The epidemiological model framework for mpox has been established over the past few decades, and many models capturing human–human, and animal–human interactions have been explored [Reference Tewinkel47]. Jezek et al. [Reference Jezek, Grab and Dixon48] constructed a stochastic model using the Monte Carlo method to simulate the chain of human-to-human transmission of mpox. The model has been validated and applied to understand the transmission potential of mpox in unvaccinated populations [Reference Jezek, Grab and Dixon48]. Bhunu and Mushayabasa [Reference Bhunu, Mushayabasa and Hyman49] presented a basic SIR compartmental model to examine the transmission dynamics of mpox between humans and non-humans, and Betti et al. [Reference Betti, Farrell and Heffernan50] present a SIR model with additional pair-formation dynamics to account for transmission via prolonged close contact between individuals.
We summarize parameters determined by mpox epidemiological modelling studies in Table 1. For the non-human population, mpox parameters are found to be: 2 yr−1 for the rate of recruitment for susceptibles, a natural death rate of 1.5 yr−1, the death rate due to mpox is given as 0.4 yr−1, and the rate of immunity is given as 0.6 yr−1 [Reference Bhunu and Mushayabasa51]. Pre-2022, for the human population, mpox parameters were found to be: 0.029 yr−1 for recruitment rate of susceptibles, a natural death rate of 0.02 yr−1, the death rate due to mpox of 0.1–0.17 yr−1, and permanent immunity rate of 0.83–0.9 yr−1 [Reference Bhunu and Mushayabasa51]. The animal-only endemic equilibrium is globally asymptotically stable when R 0n > 1 and R 0h < 1. The endemic equilibrium, where mpox infections exist in both the human and non-human populations, was shown to be locally asymptotically stable when R 0h > 1, but close to 1 [Reference Bhunu and Mushayabasa51].
Models with vaccination
The SIR model often oversimplifies complex disease transmission dynamics. For example, the SIR model does not consider the incubation duration, defined as the span of time between when an individual is exposed to a disease and when that individual becomes infected. We refer to Tolles and Luong [Reference Tolles and Luong52] who highlight limitations of the traditional SIR model, including that it results in often over-simplified assumptions about the population dynamics. Thus, most epidemiological work involves SIR-inspired models with more mathematical complexity to account for complex population dynamics. The Susceptible-Exposed-Infected-Recovered (SEIR) model has been widely used to study infectious disease dynamics. In the SEIR model, an exposed compartment (E) is added to the fundamental SIR model, representing individuals who are exposed but have not yet been contagious, such that they experience an incubation period. Mitigation strategies such as vaccination can also be considered. For example, Usman et al. [Reference Usman and Adamu53] developed an SVEIR model (including a vaccinated component) that accounts for a varied incubation period and individual vaccination status. They found that adequate vaccination and treatment policies could dramatically reduce the spread of mpox among humans. Based on mpox parameters prior to year 2017, they conclude that an increase in vaccination control parameters leads to a decrease in the basic reproduction number. Emeka et al. [Reference Emeka, Ounorah, Eguda and Babangida54] also incorporate a vaccine compartment in a population of mpox-susceptible individuals and generally find that mpox outbreaks do not occur in populations of vaccinated individuals.
Building on the work of Usman and Adamu [Reference Usman and Adamu53], Bankuru et al. [Reference Bankuru, Kossol, Hou, Mahmoudi, Rychtář and Taylor55] introduced a simplified SIR model of the mpox dynamics, providing closed-form formulas for equilibrium states of this disease dynamics, allowing for direct calculations of the semi-endemic equilibrium (Figure 3). They showed there exists a semi-endemic equilibrium in which there is no infection in the squirrel population, while the disease still persists in the human population. They found that the optimal vaccination rate amongst humans is about 0.04 vaccine/year, meaning that individuals should be advised to vaccinate approximately once every 25 years. They also found the optimal vaccination rate is about 10 times more sensitive to parameters related to animal hosts than to a corresponding parameter related to humans, thus concluding that more precise information about reservoir hosts is needed [Reference Bankuru, Kossol, Hou, Mahmoudi, Rychtář and Taylor55].

Figure 3. Costs versus vaccination rate with a high rate of the effective human-to-human transmission (βhh = 60). (a) Fully endemic equilibrium and (b) Semi-endemic equilibrium. Reprinted by permission from PeerJ from ref. [Reference Bankuru, Kossol, Hou, Mahmoudi, Rychtář and Taylor55]. Copyright 2020.
As countries such as the UK are purchasing large quantities of vaccines for public dissemination, given that vaccine efficacy has been found to drop at a rate of 1.29% per year [Reference Nguyen, Ajisegiri, Costantino, Chughtai and MacIntyre42], mathematical modelling studies such as that done by Bankuru et al. [Reference Bankuru, Kossol, Hou, Mahmoudi, Rychtář and Taylor55] can be used to inform vaccination rates, as well as which proportion of the population needs to be vaccinated to achieve herd immunity. Another important factor explored by Bankuru et al. [Reference Bankuru, Kossol, Hou, Mahmoudi, Rychtář and Taylor55] is the cost of vaccination. Where cost is defined in a game-theoretic sense, the cost of not vaccinating is given by the product of the cost of infection with the probability of becoming infected. In Figure 3, we include plots of cost as a function of vaccination rate when the human–human transmission rate is high, where Bankuru et al. [Reference Bankuru, Kossol, Hou, Mahmoudi, Rychtář and Taylor55] find that the overall cost of vaccinating is much lower compared to not-vaccinating for most epidemic scenarios.
A combination of historical data and epidemiological modelling was used to estimate the basic reproduction number, R 0, of mpox in the Democratic Republic of the Congo during 1966–1984 to be between 1.46 and 2.17 [Reference Grant, Nguyen and Breban56]. Mpox R 0 was thus significantly less than smallpox which had an estimated range of R 0 of 3.2–6.9 [Reference Gani and Leach57, Reference Costantino, Kunasekaran, Chughtai and MacIntyre58]. Due to the lasting immunity from the smallpox vaccine, mpox was deemed not self-sustainable in human populations in the DRC from 1980–1984 [Reference Grant, Nguyen and Breban56]. However, by the year 2011 estimates show that the immunity from smallpox vaccination against mpox had fallen to 60% in non-endemic countries [Reference Grant, Nguyen and Breban56]. Hence, mpox has long been hypothesized to have increasing potential to emerge as an epidemic in humans in historically non-endemic countries.
Epidemiological modelling studies on the 2022 global outbreak
Population-level human-to-human models of mpox throughout the 2022 epidemic have been largely based on SIR and SEIR frameworks. These modelling studies consider public health mitigation strategies (e.g., quarantine and vaccines), contact tracing, and sexual mixing models. For reference, the current scenario of global cumulative mpox cases by country is shown in Figure 1, and with cases normalized by country population shown in Figure 2. We further include the current global trend as a function of time for 2022, as shown in Figure 4b. We next go through current modelling literature on the 2022 epidemic, and how modelling is bringing further mechanistic insight into mpox dynamics.
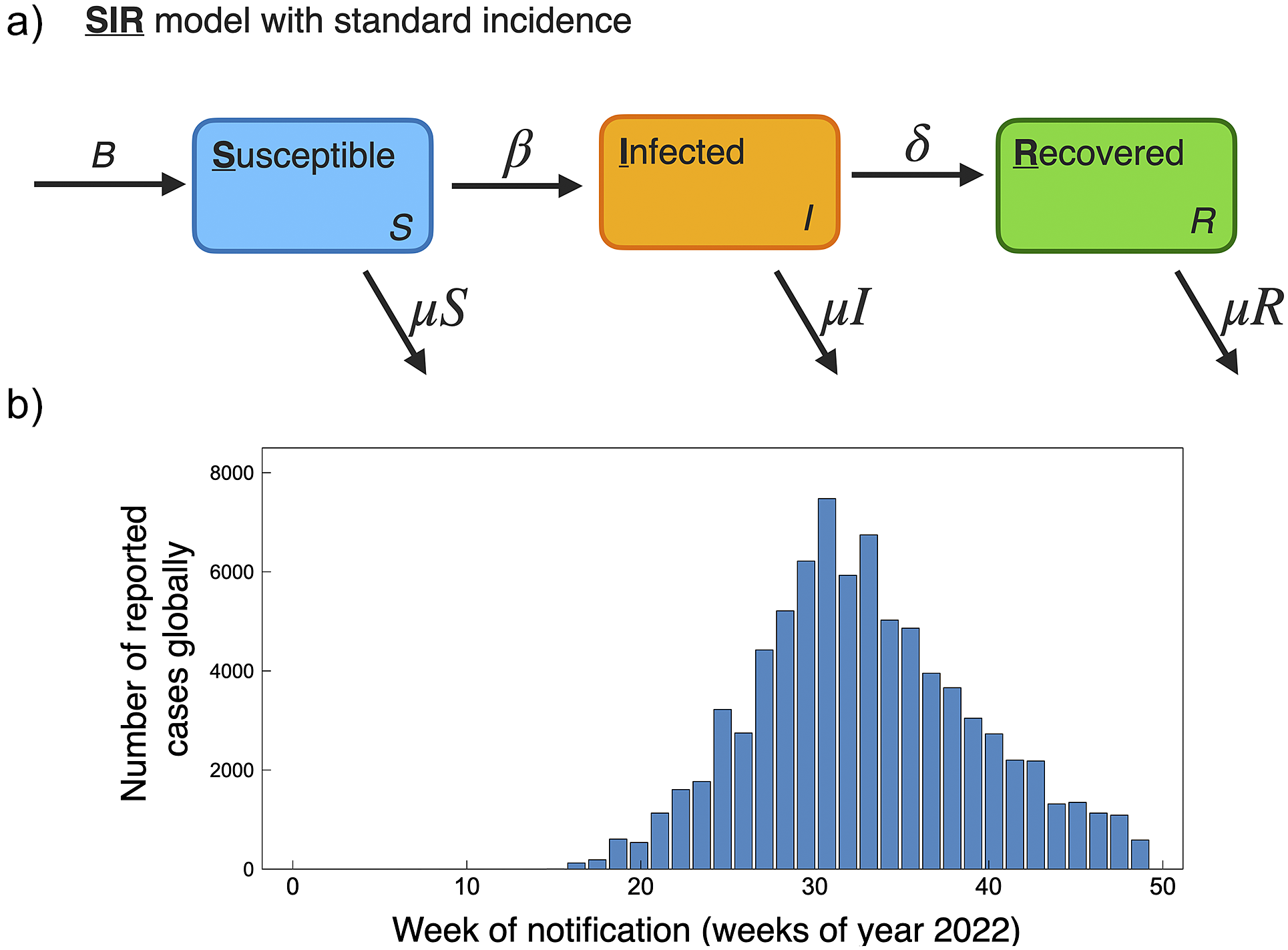
Figure 4. (a) Schematic of basic SIR model with standard incidence, similar as used to model mpox dynamics for the 2022 pandemic [Reference Betti, Farrell and Heffernan50, Reference Al-Raeei59]. (b) Global reported mpox cases as a function of weeks for the year 2022. Data accessed from publicly available WHO data (ref. [3], accessed 17 November2022).
The increase in cases from the 2022 mpox global outbreak has been shown to be strongly associated with close intimate sexual contact [Reference Palich, Burrel, Monsel, Nouchi, Bleibtreu, Seang, Bérot, Brin, Gavaud, Wakim, Godefroy, Fayçal, Tamzali, Grunemwald, Ohayon, Todesco, Leducq, Marot, Calvez, Marcelin and Pourcher33]. For the 2022 epidemic, mpox spread has been predominantly transmitted between men who have sex with men, with one study of 528 diagnosed infections finding 98% of infected persons to be gay or bisexual males [Reference Thornhill, Barkati, Walmsley, Rockstroh, Antinori, Harrison, Palich, Nori, Reeves, Habibi, Apea, Boesecke, Vandekerckhove, Yakubovsky, Sendagorta, Blanco, Florence, Moschese, Maltez, Goorhuis, Pourcher, Migaud, Noe, Pintado, Maggi, Hansen, Hoffmann, Lezama, Mussini, Cattelan, Makofane, Tan, Nozza, Nemeth, Klein and Orkin60]. Data-driven individual-level and population-level modelling studies can be used to outline the importance of public health policies and various mitigation strategies. For example, the model presented by Betti et al. [Reference Betti, Farrell and Heffernan50] presents a novel framework that includes pair formation (accounting for prolonged close contact between individuals) to describe mpox transmission. They show their pair-formation model captures population trends in data with an estimated R 0 of 2.3, and they further predict the occurrence of future waves of infection. Similarly, Bragazzi et al. [Reference Bragazzi, Han, Iyaniwura, Omame, Shausan, Wang, Woldegerima, Wu and Kong61] develop a SEIQR model that includes the sexual behaviour of high-risk individuals and find that R 0 amongst the high-risk population to be ∼1.5, whereas amongst the low-risk population to be as low as 0.007 [Reference Bragazzi, Han, Iyaniwura, Omame, Shausan, Wang, Woldegerima, Wu and Kong61]. Modelling has also been used to disentangle the many factors leading the decline of mpox in the 2022 outbreak. For example, through examining changes in sexual behavioural activity versus vaccination campaigns, studies have found the initial downturn in cases during the 2022 epidemic was largely due to changes in sexual behaviour [Reference Xiridou, Miura, Adam, de Coul, de Wit and Wallinga62, Reference Clay, Asher, Carnes, Copen, Delaney, Payne, Pollock, Mermin, Nakazawa, Still, Mangla and Spicknall63].
Network models have also demonstrated utility towards understanding mpox transmission dynamics. Bisanzio et al. [Reference Bisanzio and Reithinger64] utilize a recently developed individual-based modelling framework [Reference Bisanzio, Reithinger, Alqunaibet, Almudarra, Alsukait, Dong, Zhang, El-Saharty and Herbst65] whereby they simulate the spread of mpox in a network of 50 million susceptible individuals distributed across N cells to represent a population density characteristic of a typical European country of land mass similar to France or Spain. With spread amongst the population driven by an SEIR model, they predict mpox outbreaks lasting 23–37 weeks where mitigation strategies such as contact tracing with isolation followed by vaccination could reduce the median duration of a mpox outbreak by as much as 75%. Another network model by Van Dijck et al. [Reference Van Dijck, Hens, Kenyon and Tsoumanis66] explores the ramifications of undiagnosed mpox cases and predict that if 10% of mpox contacts abstain from sexual activity, this could result in a 35% reduction in cases. Another contact tracing study on the transmission dynamics in the UK predicted the epidemic peak to occur in early July of 2022, and further found that a significant number of cases were caused by pre-symptomatic transmission and determined a mean incubation period of 8.5 days [Reference Ward, Christie, Paton, Cumming and Overton67].
Compartmental and game-theoretic modelling, as well as modelling infection curves with comparably simple logistic functions, has also proven to be beneficial towards revealing mpox population dynamics and the consequences of various mitigation strategies. Mingione et al. [Reference Mingione, Ciccozzi, Falcone and Maruotti68] apply the generalized logistic curve to country-wide data from the top 10 non-endemic countries experiencing mpox outbreaks and find agreement with the literature that containment of the outbreak is feasible over the short term if mitigation strategies are employed. Building on previous work similar to ref. [Reference Bankuru, Kossol, Hou, Mahmoudi, Rychtář and Taylor55], Augsburger et al. [Reference Augsburger, Galanthay, Tarosky, Rychtář and Taylor69] employ an SVEIR-based model to the 2022 global pandemic and further explore vaccination in a game-theoretic framework where individuals consider cost and benefits to vaccination. They find without vaccination mpox prevalence is predicted to be approximately 3.5 cases per 104 individuals, while with optimal voluntary vaccination, prevalence is predicted to be approximately 0.5 cases per 104 individuals. Thus, vaccination is predicted to be a strong mitigative factor in reducing mpox prevalence and minimizing the chances of mpox becoming endemic is historically non-endemic countries. Savinkina et al. [Reference Savinkina, Chitwood, Kwon, Pitzer, Gonsalves and Paltiel70] employ an SEIR-based model, utilizing previously published assumptions on low-risk and high-risk population-level reproduction numbers, and simulate the spread of mpox on college campuses. In their hypothetical analysis, they find the absence of mitigation leads to an 83% chance of sustained transmission.
The population modelling studies of the 2022 global outbreak all agree, based on current data on mpox trends, that the outbreaks occurring in non-endemic countries are generally under control and on a declining trend. This is of course supported by the current global trend in cases; a histogram of global case counts up to 29 November 2022 is shown in Figure 4b. A summary of 2022 mpox mathematical modelling population parameters is provided in Table 1.
Epidemiological modelling studies are important for policy decision-makers when deciding which mitigation strategy or control measures (such as isolation and lockdown measures) to employ. Predictive modelling for future mpox peaks will be important in aiding policy decision-makers. For example, modelling techniques on mpox have been developed to estimate the true number of unreported cases, and further, have shown promise to accurately predict infection cycles [Reference Overton, Abbott, Christie, Cumming, Day, Jones, Paton, Turner and Ward71].
Modelling studies clearly highlight the important of mitigation strategies. For example, vaccination campaigns should be organized to reduce population infectivity and further reduce the probability of allowing a more virulent and transmissive mpox strain to emerge. Yuan et al. [Reference Yuan, Tan, Yang, Aruffo, Ogden, Belair, Arino, Heffernan, Watmough, Carabin and Zhu72] consider an SEIR model whereby the population is divided into high and low risk and focus their study on mass gathering scenarios. They find that a broad vaccination campaign is less effective in curbing the spread of mpox than compared to contact tracing, isolation, and vaccination of close contacts. They further posit that the ring vaccination strategy may be inadequate in preventing an outbreak from occurring; however, it does still result in fewer case counts [Reference Yuan, Tan, Yang, Aruffo, Ogden, Belair, Arino, Heffernan, Watmough, Carabin and Zhu72]. They follow up their work with a study to consider the mpox threat to the low-risk population if viral transmissibility increases [Reference Yuan, Tan, Yang, Aruffo, Ogden, Bélair, Heffernan, Arino, Watmough, Carabin and Zhu73]. They conclude that isolation, contact tracing, and quarantine are key mitigation strategies to prevent infection in the event of increased viral transmission into low-risk populations [Reference Yuan, Tan, Yang, Aruffo, Ogden, Bélair, Heffernan, Arino, Watmough, Carabin and Zhu73]. Predictive modelling for future mpox peaks can be an important factor in aiding policy decision-makers. For example, based on Canadian mpox trends, there are predicted to be further peaks occurring on an approximately annual basis [Reference Betti, Farrell and Heffernan50].
As noted, the 2022-mpox strain is predominantly spreading through close intimate contacts [Reference Palich, Burrel, Monsel, Nouchi, Bleibtreu, Seang, Bérot, Brin, Gavaud, Wakim, Godefroy, Fayçal, Tamzali, Grunemwald, Ohayon, Todesco, Leducq, Marot, Calvez, Marcelin and Pourcher33]. However, orthopoxviruses, such as smallpox, are known to transmit via a respiratory route [Reference McCollum and Damon74]. Currently, a respiratory transmission mode is not found to play a major role in the 2022 outbreak [Reference Palich, Burrel, Monsel, Nouchi, Bleibtreu, Seang, Bérot, Brin, Gavaud, Wakim, Godefroy, Fayçal, Tamzali, Grunemwald, Ohayon, Todesco, Leducq, Marot, Calvez, Marcelin and Pourcher33, Reference Suñer, Ubals, Tarín-Vicente, Mendoza, Alemany, Hernández-Rodríguez, Casañ, Descalzo, Ouchi, Marc, Rivero, Coll, Oller, Miguel Cabrera, Vall-Mayans, Dolores Folgueira, Ángeles Melendez, Agud-Dios, Gil-Cruz, Paris de Leon, Ramírez Marinero, Buhiichyk, Galván-Casas, Paredes, Prat, Sala Farre, Bonet-Simó, Farré, Ortiz-Romero, Clotet, García-Patos, Casabona, Guedj, Cardona, Blanco, Marks and Mitjà34]. Thus, modelling studies, such as bottleneck studies [Reference Sigal, Reid and Wahl75], that aim to predict mutation lineages and probabilities of mutant transmission, can play an important role in predicting the potential severity of future mpox mutants. The concern that mpox could mutate to find a respiratory transmission route is warranted. The cost and benefit of mitigation strategies, including the details of how they can be disseminated to the public, can be readily explored through modelling studies to aid public health campaigns should the virus emerge with a more infectious mutant.
Immunity decline hypothesis
The recent 2022 emergence and outbreaks of mpox are still under investigation. One hypothesis for the increase in cases relates to the decline in population cross-immunity provided by the smallpox vaccine [Reference Rimoin, Mulembakani, Johnston, Smith, Kisalu, Kinkela, Blumberg, Thomassen, Pike, Fair, Wolfe, Shongo, Graham, Formenty, Okitolonda, Hensley, Meyer, Wright and Muyembe76, Reference Spath, Brunner-Ziegler, Stamm, Thalhammer, Kundi, Purkhauser and Handisurya77]. In 1980, the WHO declared the eradication of smallpox. Soon afterwards, routine smallpox immunization ended worldwide [78]. Smallpox vaccine has proven to induce humoral and cell-mediated responses against orthopoxviruses [Reference Gilchuk, Gilchuk, Sapparapu, Lampley, Singh, Kose, Blum, Hughes, Satheshkumar, Townsend, Kondas, Reed, Weiner, Olson, Hammarlund, Raue, Slifka, Slaughter, Graham, Edwards, Eisenberg, Cohen, Joyce and Crowe79, Reference Kennedy, Ovsyannikova, Jacobson and Poland80], creating a heterotypic immunity composed of a wide array of antigen receptors [Reference Kumar, Suryadevara, Wolf, Wilson, Di Paolo, Brien and Joyce81] and estimated to have an efficacy of 85% in preventing mpox infection in humans [Reference Grant, Nguyen and Breban56]. Thus, it has been suggested that younger generations not vaccinated against smallpox are vulnerable to mpox infection. This section will discuss the current evidence from mathematical models testing the declining immunity from vaccination in increasing susceptibility to mpox. Data from the Democratic Republic of the Congo (DRC) revealed that individuals born before the official vaccination cessation had a 5.21-fold lower risk of mpox infection than unvaccinated persons [Reference Rimoin, Mulembakani, Johnston, Smith, Kisalu, Kinkela, Blumberg, Thomassen, Pike, Fair, Wolfe, Shongo, Graham, Formenty, Okitolonda, Hensley, Meyer, Wright and Muyembe76, Reference Petersen, Kantele, Koopmans, Asogun, Yinka-Ogunleye, Ihekweazu and Zumla82]. Nguyen et al. [Reference Rao, Petersen, Whitehill, Razeq, Isaacs, Merchlinsky, Campos-Outcalt, Morgan, Damon, Sánchez and Bell83] modelled the declining immunity in Nigeria, accounting for individual-level declining immunity at a rate of 1.29% per year, as well as country-wide declining immunity using weighted regional estimates of smallpox vaccination coverage. They found the increase in unvaccinated and immunologically naive population (90.7% of the total population in Nigeria in 2018), and together with the decline from 85% to 23.1% in efficacy from cross-immunity protection provided by smallpox vaccination, and that the overall population immunity was estimated to be only 2.2% as of 2018 [Reference Nguyen, Ajisegiri, Costantino, Chughtai and MacIntyre42]. Shown in Figure 5a, we include an example of their findings.

Figure 5. (a) Visualization of the relationship between smallpox vaccination and cross-immunity conferred to mpox virus rates at a population (blue) and individual level (red) in Nigeria from 1970 to 2018. Reprinted by permission from Centers for Disease Control and Prevention from ref. [Reference Nguyen, Ajisegiri, Costantino, Chughtai and MacIntyre42]. Copyright 2021. (b) Predicted change of the reproduction number R for MPX as a function of immunity in a population to orthopoxvirus species (provided by smallpox vaccine). Blue circles show a scenario where the vaccination percentage is high, most of the population presents high-level cross-immunity against orthopoxvirus species, and the mpox R value is low. Yellow circles show the scenario where vaccination and cross-immunity rates are low, and mpox R-value increases to >2.5. Reprinted by permission from the World Health Organization from ref. [Reference Grant, Nguyen and Breban56]. Copyright 2020.
The declining immunity from vaccination to smallpox represents an epidemiological threat by increasing the mpox reproduction number. The basic reproduction number, R0, of any infectious disease is dynamic and depends on many variables, including characteristics of the pathogen characteristics and the host. Grant et al. [Reference Grant, Nguyen and Breban56] modelled this relationship with data from the DRC. They determine an mpox reproduction number, R. R is given by R = R 0(1 − ϵp), where ϵ represents the vaccine efficacy, and p the vaccination coverage. Given a current immunity estimate, they determine R could be higher than 2.5 [Reference Grant, Nguyen and Breban56]. We include a plot of their results for R as a function immunity in Figure 5b.
The increase in attack rate over time may be evidence for the immunity decline hypothesis as well. Mpox household attack rates amongst the unvaccinated and vaccinated were reported as 15% and 0.4%, respectively, in 1985 [Reference Arita, Jezek, Khodakevich and Ruti22]. The 2013 outbreak in the DRC, which represented a 600-fold increase in annual infections, was found to have a household attack rate of 50%, where many people who contracted mpox were previous smallpox vaccine recipients [Reference Nolen, Osadebe, Katomba, Likofata, Mukadi, Monroe, Doty, Hughes, Kabamba, Malekani, Bomponda, Lokota, Balilo, Likafi, Lushima, Ilunga, Nkawa, Pukuta, Karhemere, Tamfum, Nguete, Wemakoy, McCollum and Reynolds84].
The loss of immunity hypothesis is not mutually exclusive from other re-emergence theories, such as the increased exposure to wildlife, reservoir expansion, globalization, and mutations to mpox fitness traits. These factors represent critical barriers to consider for mpox spillover opportunity [Reference Plowright, Parrish, McCallum, Hudson, Ko, Graham and Lloyd-Smith85]. An increase in the mpox immune-naive population and the risk of exposure create a niche for continued mpox animal-to-human and human-to-human transmission, longer chains of infection, and thus an opportunity for mutation in mpox viral transmission traits. Pre-2022, human-to-human transmission chains have been relatively short-lived, and stochastic models performed in the 1980s based on historical data found mpox to have a low probability to be established in human populations [Reference Jezek, Grab and Dixon48]. However, more recent models have shown that sustained human-to-human transmissions could favour pathogen evolution, creating a potential existence of semi-endemic or fully endemic equilibrium [Reference Grant, Nguyen and Breban56, Reference Reynolds, Carroll and Karem86].
A clustered epidemiological differential model developed by Ali et al. [Reference Ali, Rubin and Sarkar87] considered human behavioural dynamics such as vaccination and drug hesitancy, cooperation, and mobility rate and showed how opination dynamics have a tremendous impact on fatality rates. Furthermore, models on voluntary vaccination have shown the potential control of mpox outbreaks in a semi-endemic equilibrium but not in a fully endemic one [Reference Bankuru, Kossol, Hou, Mahmoudi, Rychtář and Taylor55]. In an endemic equilibrium scenario, deterministic compartmental models showed that isolation of infected individuals, in combination with adequate treatment and vaccination, plays an essential role in the control and eradication of mpox [Reference Usman and Adamu53, Reference Peter, Kumar, Kumari, Oguntolu, Oshinubi and Musa88]. Modelling efforts show vaccination remains a high-potential primary mpox mitigation strategy and should continue to be prioritized in endemic regions [Reference Rimoin, Mulembakani, Johnston, Smith, Kisalu, Kinkela, Blumberg, Thomassen, Pike, Fair, Wolfe, Shongo, Graham, Formenty, Okitolonda, Hensley, Meyer, Wright and Muyembe76]. However, to achieve effective mpox management, a combination of countermeasures needs to be considered. Novel mpox-specific vaccines [Reference Mucker, Golden, Hammerbeck, Kishimori, Royals, Joselyn, Ballantyne, Nalca and Hooper89, Reference Xiao, Zeng, Schante, Joshi, Buchman, Volkin, Middaugh and Isaacs90], treatments [Reference Siegrist, Kinahan, Settecerri, Greene and Santarpia91–Reference Hutson, Kondas, Mauldin, Doty, Grossi, Morgan, Ostergaard, Hughes, Nakazawa, Kling, Martin, Ellison, Carroll, Gallardo-Romero and Olson93], and prophylaxis public health measures [Reference Ali, Rubin and Sarkar87, Reference Stittelaar, Neyts, Naesens, Van Amerongen, Van Lavieren, Holý, Clercq, Niesters, Fries, Maas, Mulder, Van Der Zeijst and Osterhaus94, Reference Melamed, Israely and Paran95] are all under development to mitigate mpox spread.
Effect of reservoirs and wildlife control measures
A report by the WHO in 1968 concluded that mpox transmission between monkeys is ‘infrequent’ and that most likely another animal reservoir existed [Reference Arita and Henderson18]. A definitive mpox virus reservoir host is still unknown and under study. Currently, giant-pouched rats, rope squirrels, and African dormice are posited as the most likely candidates [Reference Bankuru, Kossol, Hou, Mahmoudi, Rychtář and Taylor55, Reference Riopelle, Munster and Port103]. Throughout the 1980s, the animal–animal spread was found with particular prevalence in squirrels of the Funisciurus anerythrus species, where it was shown they sustain mpox viral transmission in areas near human settlements [Reference Khodakevich, Szczeniowski, Disu, Jezek, Marennikova, Nakano and Messinger104]. Squirrel mpox-related death rates and recovery rates were later found to be approximately 17.5 and 12 days, respectively [Reference Falendysz, Lopera, Doty, Nakazawa, Crill, Lorenzsonn, Kalemba, Ronderos, Mejia, Malekani, Karem, Carroll, Osorio and Rocke102] (see Table 1).
During the 2022 global mpox outbreak, it was discovered that human-to-dog transmission is possible, thus raising concerns about further dog-to-dog and dog-to-human transmission [Reference Seang, Burrel, Todesco, Leducq, Monsel, Le Pluart, Cordevant, Pourcher and Palich105]. Culling, the reduction in wild animal populations through selective slaughter, has been employed as a method for wildlife reservoir management and to mitigate the potential of further animal-to-human transmission [Reference Miguel, Grosbois, Caron, Pople, Roche and Donnelly106]. For example, culling has been employed recently during the SARS-CoV-2 pandemic to mitigate further animal-to-animal transmission amongst farmed minks [Reference Pomorska-Mól, Wodarek, Gogulski and Rybska107]. Culling to prevent further mpox spread has been explored through transmission modelling approaches, where it has been found to be ineffective and can lead to the counter-productive outcome of increasing mpox infection. This is because culling results in the sudden removal of mature animals with immunity replaced with juvenile, more susceptible animals, thus increasing the probability of outbreaks [Reference Tchuenche and Bauch108].
Climatic variables influencing mpox transmission: a One Health approach
The One Health approach aims to recognize the strong linkage between the health of humans, animals, plants, and the environment, to develop integrated and sustainable solutions [Reference Adisasmito, Almuhairi, Behravesh, Bilivogui, Bukachi, Casas, Becerra, Charron, Chaudhary and Zanella109]. Given the interconnected coexistence between humans, animals, and the environment, mpox emergence in the context of climate change represents a One Health challenge [Reference Zinsstag, Crump, Schelling, Hattendorf, Maidane, Ali, Muhummed, Umer, Aliyi and Nooh110, Reference Laing, Duffy, Anderson, Antoine-Moussiaux, Aragrande, Beber, Berezowski, Boriani, Canali and Carmo111]. From a One Health perspective, we present current evidence on mathematical modelling connecting climate change impacts on the environment, animals, and humans, to mpox dynamics.
Climate change has altered human–environment systems [112]. The emergence and re-emergence of many infectious diseases are projected to increase due to the negative impact of climate change [113, Reference Mills, Gage and Khan114]. Interactions between the three factors embodied in the epidemiological triangle: the virus (agent), the human (host), and the reservoir (environment) [Reference Lilienfeld, Lilienfeld and Stolley115], have been found to contribute to mpox emergence and expansion. In addition to the decrease in herd immunity caused by the cessation of smallpox vaccination (discussed in detail in Sections 4.2 and 4.4), climatic variables and human behaviour have created an ideal niche for mpox transmission [Reference Alakunle, Moens, Nchinda and Okeke116, Reference Simpson, Heymann, Brown, Edmunds, Elsgaard, Fine, Hochrein, Hoff, Green, Ihekweazu, Jones, Lule, Maclennan, McCollum, Mühlemann, Nightingale, Ogoina, Ogunleye, Petersen, Powell, Quantick, Rimoin, Ulaeato and Wapling117]. In this section, we discuss the current model-based evidence for mpox transmission, emphasizing the influence of climate factors.
The impact on human health from climate change is an emerging topic. There is a consensus on increased adverse climate-related health outcomes such as food insecurity, heath-related mortality and morbidity, mental health damage, or injuries [112]. Impacts on health can include the impairment of the immune system due to direct or indirect effects of climate change.
There has been significant scientific interest in mpox spread within endemic African countries with particular attention to mpox biogeographic barriers [Reference Nakazawa, Mauldin, Emerson, Reynolds, Lash, Gao, Zhao, Li, Muyembe, Kingebeni, Wemakoy, Malekani, Karem, Damon and Carroll121]. Environmental conditions can define the spread and durability of pathogens outside their hosts. Survival models have shown that orthopoxviruses are high-virulence high-survival pathogens, which implies high durability outside their host [Reference Walther and Ewald122]. Seasonal patterns of mpox outbreaks have been observed during the fall season and linked to deforestation and flooding [Reference Alakunle and Okeke123]. Historical evidence suggests that dense and humid lowland tropical forests ecotones are the most favourable ecosystem for zoonotic transmission of mpox [Reference Tiee, Harrigan, Thomassen and Smith120, Reference Nakazawa, Emerson, Carroll, Zhao, Li, Reynolds, Karem, Olson, Lash, Davidson, Smith, Levine, Regnery, Sammons, Frace, Mutasim, Karsani, Muntasir, Babiker, Opoka, Chowdhary and Damon124].
Prior to the 2022 outbreak, mathematical models concluded that continued mpox human–human population spread required continued zoonotic reservoir exposure to maintain chains of transmission [Reference Fine, Jezek, Grab and Dixon27]. Therefore, much attention has been paid to mpox reservoirs; however, there is no clear consensus on the natural or definitive reservoir as of the time of writing [Reference Alakunle, Moens, Nchinda and Okeke116, Reference Parker, Nuara, Buller and Schultz125]. It is known that environmental conditions can affect the transmission of mpox between animals [Reference Brown and Leggat126]. Having an unknown primary reservoir for mpox limits a model’s accuracy in the prediction of the impact of climate variables on the animal–animal and animal–human dynamics [Reference Reynolds, Doty, McCollum, Olson and Nakazawa127]. Multivariate analyses of historical data have demonstrated that mpox can co-occur on several species in an unanticipated manner [Reference Tiee, Harrigan, Thomassen and Smith120, Reference Fuller, Thomassen, Mulembakani, Johnston, Lloyd-Smith, Kisalu, Lutete, Blumberg, Fair, Wolfe, Shongo, Formenty, Meyer, Wright, Muyembe, Buermann, Saatchi, Okitolonda, Hensley, Smith and Rimoin128]. Additionally, ecological niche modelling techniques have been used to model the climate and spatial distribution of mpox [Reference Peterson, Papeş, Reynolds, Perry, Hanson, Regnery, Hutson, Muizniek, Damon and Carroll118, Reference Fuller, Thomassen, Mulembakani, Johnston, Lloyd-Smith, Kisalu, Lutete, Blumberg, Fair, Wolfe, Shongo, Formenty, Meyer, Wright, Muyembe, Buermann, Saatchi, Okitolonda, Hensley, Smith and Rimoin128, Reference Mandja, Brembilla, Handschumacher, Bompangue, Gonzalez, Muyembe and Mauny129], where these modelling studies emphasized the critical role of ecosystem variation on reservoir distribution (shown in Figure 6).

Figure 6. (a) Two-dimensional representation (annual mean temperature and annual mean precipitation) of ecological niche models developed for two mpox reservoir species cricetomys gambianus (white diamonds) and cricetomys emini (grey diamonds) across tropical sub-Saharan Africa. Reprinted by permission from Oxford University Press from ref. [Reference Peterson, Papeş, Reynolds, Perry, Hanson, Regnery, Hutson, Muizniek, Damon and Carroll118]. Copyright 2006. (b) Observed and predicted human mpox occurrence under present and future climate conditions with reservoir species as predictor variables in Central and Western Africa. The average projected change in occurrence probability for eight climate change scenarios for 2050 (middle) and 2080 (bottom). Reprinted and modified by permission from PLOS One from ref. [Reference Thomassen, Fuller, Asefi-Najafabady, Shiplacoff, Mulembakani, Blumberg, Johnston, Kisalu, Kinkela, Fair, Wolfe, Shongo, LeBreton, Meyer, Wright, Muyembe, Buermann, Okitolonda, Hensley, Lloyd-Smith, Smith and Rimoin119]. Copyright 2013. (c) mpox prevalence detected in dried museum specimens of potential mpox reservoir species, with an underlying layer representing tree cover, with darker greens corresponding to high cover percentages. Reprinted by permission from The Royal Society Publishing from ref. [Reference Tiee, Harrigan, Thomassen and Smith120]. Copyright 2018.
Understanding mpox spatial ecology is essential to predict future outbreaks under climate change conditions. Spatial and probabilistic models have been used to study mpox occurrence, particularly in Africa (see, e.g., Figure 6b,c). Including climatic variables has been demonstrated to be critical in the spatial analysis of mpox at a local scale and regional scale [Reference Thomassen, Fuller, Asefi-Najafabady, Shiplacoff, Mulembakani, Blumberg, Johnston, Kisalu, Kinkela, Fair, Wolfe, Shongo, LeBreton, Meyer, Wright, Muyembe, Buermann, Okitolonda, Hensley, Lloyd-Smith, Smith and Rimoin119, Reference Rogers and Randolph130]. Climatic variables such as temperature and precipitation seasonality are reservoir species predictors, meaning that a small change in those variables could also change the reservoir dynamics and thus animal–human transmission probability [Reference Peterson, Papeş, Reynolds, Perry, Hanson, Regnery, Hutson, Muizniek, Damon and Carroll118, Reference Thomassen, Fuller, Asefi-Najafabady, Shiplacoff, Mulembakani, Blumberg, Johnston, Kisalu, Kinkela, Fair, Wolfe, Shongo, LeBreton, Meyer, Wright, Muyembe, Buermann, Okitolonda, Hensley, Lloyd-Smith, Smith and Rimoin119]. Furthermore, climatic and ecosystem variables can increase habitat suitability for potential mpox reservoirs and, by extension, more frequent wildlife–human contact [Reference Nakazawa, Emerson, Carroll, Zhao, Li, Reynolds, Karem, Olson, Lash, Davidson, Smith, Levine, Regnery, Sammons, Frace, Mutasim, Karsani, Muntasir, Babiker, Opoka, Chowdhary and Damon124, Reference Fuller, Thomassen, Mulembakani, Johnston, Lloyd-Smith, Kisalu, Lutete, Blumberg, Fair, Wolfe, Shongo, Formenty, Meyer, Wright, Muyembe, Buermann, Saatchi, Okitolonda, Hensley, Smith and Rimoin128]. Other extreme weather events, such as droughts [131], can force carrying mpox species to move closer to human settlements [Reference Thomassen, Fuller, Asefi-Najafabady, Shiplacoff, Mulembakani, Blumberg, Johnston, Kisalu, Kinkela, Fair, Wolfe, Shongo, LeBreton, Meyer, Wright, Muyembe, Buermann, Okitolonda, Hensley, Lloyd-Smith, Smith and Rimoin119]. Future research predicting shifts in reservoir species should also focus on how this dynamic is affected by environmental changes. We propose that models should include the viral dynamic considerations of interrupting or increasing wildlife–human interaction frequency under climate change scenarios.
Wastewater-based epidemiology to monitor mpox levels
Wastewater-based epidemiology (WBE) is a population-level biomarker surveillance method to analyse wastewater for either chemicals or pathogens [Reference Choi, Tscharke, Donner, O’Brien, Grant, Kaserzon, Mackie, O’Malley, Crosbie and Thomas132, Reference Lorenzo and Picó133]. WBE has been shown to be able to estimate mpox population trends through time; however, key gaps in our understanding of the application of WBE to mpox have been highlighted, namely, the current lack of a 100% inclusive WBE methodology and limits cross-reactivity with non-targeted species [Reference Tiwari, Adhikari, Kaya, Islam, Malla, Sherchan, Al-Mustapha, Kumar, Aggarwal and Bhattacharya134]. Towards applying WBE to mpox, Chen and Bibby [Reference Chen and Bibby135] developed a Monte Carlo approach to estimate the probability of detecting mpox DNA in wastewater. They determine that United States wastewater treatment plants may be able to detect 7 infections out of 100,000 people based on previously reported daily shedding rates.
Towards a within-host model for mpox
The goal of within-host modelling is to represent the complex physiological processes of a disease, or therapeutic, within the body with mathematical models [Reference Ciupe and Heffernan7]. Within-host mathematical models are developed under biological principles and then fit longitudinal serological data to estimate various aspects of physiological dynamical outcomes. Modelling of in-host pathogen dynamics has proven critical towards furthering our understanding of HIV, HCV, HBV, HSV, influenza, and SARS-CoV-2 as well as aiding the development of vaccine therapies [Reference Perelson and Ribeiro8–Reference Néant, Lingas, Le Hingrat, Ghosn, Engelmann, Lepiller, Gaymard, Ferré, Hartard, Plantier, Thibault, Marlet, Montes, Bouiller, Lescure, Timsit, Faure, Poissy, Chidiac, Raffi, Kimmoun, Etienne, Richard, Tattevin bb, Garot cc, Le, Bachelet, Tardivon, Duval, Yazdanpanah, Mentré, Laouénan, Visseaux and Guedj12,Reference Farhang-sardroodi, Korosec, Gholami, Craig, Moyles, Ghaemi, Ooi and Heffernan14–Reference Korosec, Farhang-Sardroodi, Dick, Gholami, Ghaemi, Moyles, Craig, Ooi and Heffernan16,Reference Lin, Law, Korosec, Zhou, Koh, Ghaemi, Samaan, Ooi, Matveev, Yue, Gingras, Estacio, Buchholz, Cheatley, Mohammadi, Kaul, Pavinski, Mubareka, McGeer, Leis, Heffernan and Ostrowski136–Reference Padmanabhan, Desikan and Dixit139]. Following the development and fitting of a model to serological data, structural and practical identifiability methods are then employed to assess model reproducibility and reliability [Reference Sher, Niederer, Mirams, Kirpichnikova, Allen, Pathmanathan, Gavaghan, van der Graaf and Noble140]. Within-host models have been used extensively to estimate properties of disease dynamics, thus contributing to our understanding of the disease progression at the within-host scale [Reference Néant, Lingas, Le Hingrat, Ghosn, Engelmann, Lepiller, Gaymard, Ferré, Hartard, Plantier, Thibault, Marlet, Montes, Bouiller, Lescure, Timsit, Faure, Poissy, Chidiac, Raffi, Kimmoun, Etienne, Richard, Tattevin bb, Garot cc, Le, Bachelet, Tardivon, Duval, Yazdanpanah, Mentré, Laouénan, Visseaux and Guedj12,Reference Sigal, Reid and Wahl75,Reference Korosec, Betti, David, Ooi, Moyles, Wahl and Heffernan137,Reference Goyal, Cardozo-Ojeda and Schiffer141–Reference Beauchemin, Miura and Iwami146].
At the time of writing, there is a noticeable lack of within-host mechanistic modelling studies of mpox, with few within-host studies for any orthopoxvirus. The work of Ogunjimi et al. [Reference Ogunjimi, Van Den, Meysman, Heynderickx, Bergs, Jansen, Leuridan, Vorsters, Goossens, Laukens, Cools, Tendeloo, Hens, Van Damme, Smits and Beutels147], who model the CD4 trajectories of human chickenpox, to the best of our knowledge, is the only published orthopoxvirus within-host modelling work. The mpox longitudinal clinical studies outlined in Section 3 provides an overview of current knowledge of mpox serological parameters required to fit to a typical within-host model and should serve as a strong starting point for such a study.
Future directions and concluding thoughts
Mathematical modelling provides a cost-effective and non-invasive methodology for gaining actionable insights into viral dynamics and therapeutic responses at the population and within-host levels. At the within-host level, mathematical modelling utilizes serology-based diagnostics to understand disease transmission dynamics, including viral reproduction numbers, viral load clearance, and cell recovery, to understand the timescales of disease transmission.
However, such studies on mpox are currently limited. At the population level, mathematical modelling leverages population metrics, such as contact tracing data, cumulative case counts, and wastewater surveillance, to predict outbreak characteristics such as recovery rates, transmission, virulence, and reproduction numbers. Although the current mpox epidemic case counts are declining, models predict future waves to occur annually [Reference Betti, Farrell and Heffernan50]. Therefore, modelling efforts can assist in the allocation of public health resources to mitigate the future spread of infection, such as identifying when and whom to target in vaccine or education campaigns.
The burden of human infectious disease remains high in many countries, with recent outbreaks of emerging and re-emerging pathogens referred to as the ‘new era of infectious disease’ [Reference Baker, Mahmud, Miller, Rajeev, Rasambainarivo, Rice, Takahashi, Tatem, Wagner, Wang, Wesolowski, Jessica and Metcalf148]. Climate change is causing significant changes in natural ecosystems worldwide [112]. More than half of infectious diseases affecting human populations having been aggravated by climate hazards through pathways such as bringing pathogens closer to people or causing favourable changes to viral fitness traits [Reference Mora, McKenzie, Gaw, Dean, Hammerstein, Knudson, Setter, Smith, Webster, Patz and Franklin149]. Mathematical models of infectious diseases that consider climatic variables, such as accomplished for diseases such as influenza virus [Reference Liu, Tan, Sun, Hou, Fu and Wu150], West Nile virus [Reference Davis, Vincent, Hildreth, Kightlinger, Carlson and Wimberly151], SARS-CoV2 [Reference James, Salomon, Buckee and Menzies152], and Malaria [Reference Ssempiira, Kissa, Nambuusi, Mukooyo, Opigo, Makumbi, Kasasa and Vounatsou153], have demonstrated utility for policymakers in planning public health prevention and responses strategies [Reference Hassell, Newbold, Dobson, Linton, Franklinos, Zimmerman and Lohan154]. This review revealed that the practice of including climatic variables in the mathematical modelling of mpox still needs further exploration. For example, current modelling evidence suggests that climate variables can significantly impact mpox transmission and pathogenesis by affecting the reservoir–human contact environment [Reference Peterson, Papeş, Reynolds, Perry, Hanson, Regnery, Hutson, Muizniek, Damon and Carroll118–Reference Tiee, Harrigan, Thomassen and Smith120]. Therefore, it is crucial to consider climatic variables at the local, regional, and global scales in future mpox mathematical modelling studies to better understand its complex dynamics with potential reservoirs and potential impacts on human populations.
The emergence of mpox as a global threat in 2022 has resulted in over 80,000 cases in non-endemic countries as of 17 November 2022. As mpox has gained global attention, it is becoming increasingly important to conduct higher resolution studies that report regular case counts and longitudinal serological measures, such as IgGs, and CD4/CD8 responses, which can be utilized in mathematical modelling approaches to gain deeper insight into viral dynamics and predictive power. An interdisciplinary work between clinicians and mathematicians can better inform timescales of clinical data acquisition to gain the optimal information on disease dynamics from limited data sets [Reference Moyles, Korosec and Heffernan15]. To date, no within-host modelling studies of mpox have been carried out to our knowledge.
Efforts to quantify an immunological correlation of protection in humans against mpox have been reported [Reference Townsend, Keckler, Patel, Davies, Felgner, Damon and Karem41]. However, a robust correlate of protection against the 2022 strain still needs to be discovered [Reference Lum, Torres-Ruesta, Tay, Lin, Lye, Rénia and Ng30]. Mathematical approaches can leverage serological studies to correlate humoral and cellular longitudinal responses with case severity or vaccine efficacy, similar to what has been done for SARS-CoV-2 [Reference Korosec, Farhang-Sardroodi, Dick, Gholami, Ghaemi, Moyles, Craig, Ooi and Heffernan16]. It is also important to understand differences in within-host dynamics amongst cohorts containing various comorbidities, notably high-risk individuals co-infected with syphilis or HIV [Reference Bížová, Veselý, Trojánek and Rob155]. Longitudinal studies working to understand the risks of vaccination in these vulnerable populations need to be included. As has become evident throughout the SARS-CoV-2 pandemic, many long-term consequences of SARS-CoV-2 can present as neurological or psychiatric [Reference Taquet, Geddes, Husain, Luciano and Harrison156], cardiovascular [Reference Xie, Xu, Bowe and Al-Aly157], and various immunological dysfunctions [Reference Phetsouphanh, Darley, Wilson, Howe, Munier, Patel, Juno, Burrell, Kent, Dore, Kelleher and Matthews158]. Longitudinal studies to identify and understand the extent of these potential long-term consequences for moderate and severe mpox cases will become increasingly important. Mathematical modelling can help predict the proportion of individuals expected to suffer from long-term consequences of mpox infection and inform public health policy decisions.
Data availability statement
Sources for all plotted data have been referenced within the figure captions. Data are available from Chapin S. Korosec, Email: chapinSkorosec@gmail.com.
Acknowledgements
We thank OMNI-REUNIS (One Health Modelling Network for Emerging Infections) for facilitating the collaboration that lead to this work through the 2022 Health-A-Thon program. C.S.K. acknowledges the support of NSERC postdoctoral funding. This research is supported by an NSERC Discovery Grant for J.M.H.
Author contribution
Conceptualization: M.B-M., Y.Y., B.J., A.K, C.S.K.; Data curation: M.B-M., Y.Y., C.S.K.; Supervision: C.S.K.; Visualization: M.B-M., Y.Y., C.S.K.; Writing – original draft: M.B-M., Y.Y., B.J., A.K., C.S.K.; Writing – review & editing: M.B-M., Y.Y., B.J., A.K., Z.A.B., H.H.C., S.Y., I.R.M., J.M.H., C.S.K.
Financial support
C.S.K. acknowledges NSERC postdoctoral funding.
Competing interest
The authors declare that they have no competing interests.