1. Introduction
The Paleoproterozoic era (2.5−1.6 Ga) represents a period in Earth’s history when some of the world’s largest volcanogenic massive sulphide (VMS), sedimentary exhalative (Sedex), Bergslagen-type bedded, stratiform Zn-Pb-Ag mineralization hosted in rhyolitic ash-siltstone (SAS-type)/stratabound volcanic-associated, limestone-skarn (SVALS-type) and Broken Hill-type (BHT) deposits formed (e.g. Flin Flon-Snow lake VMS deposit, Gibson et al. Reference Gibson, Lafrance, Pehrsson, DeWolfe, Gilmore, Simard and Pearson2013; Broken Hill Pb-Zn-Ag deposit, Parr & Plimer, Reference Parr, Plimer, Kirkham, Sinclair, Thorpe and Duke1993; Lappbergert SVALS-type Zn-Pb-Ag-(Cu-Au) deposit, Tiu et al. Reference Tiu, Jansson, Wanhainen and Lilja2021; Rampura Agucha Zn-Pb-Ag Sedex deposit, Mishra & Bernhardt, Reference Mishra and Bernhardt2009).
In the United States, Paleoproterozoic massive sulphide deposits include VMS deposits in the ∼1.81 Ga Penokean Volcanic Belt, Wisconsin (e.g. Moleski et al. Reference Moleski, Boxleiter and Thakurta2019), the largest of which is Crandon (42.9 Mt @ 0.6% Cu, 8.4% Zn, 51 g/t Ag and 1.4 g/t Au), as well as those in the ∼1.74 Ga Jerome VMS district (>100 Mt of Cu-Zn-Pb-Au-Ag), Arizona, and the ∼1.8 to 1.7 Ga massive sulphide belt in south-central Colorado. Massive sulphide deposits in Arizona and Colorado occur in the regional Yavapai tectonic terrane, or the transitional Yavapai–Mazatzal tectonic terrane, that extends from Nevada through to Colorado. The impetus for the study was, in part, based on the observations of Karlstrom et al. (Reference Karlstrom, Harlan, Williams, McLelland, Geissman and Ahall1999) and Burrett and Berry (Reference Burrett and Berry2000) who placed North America adjacent to Australia in the Neoproterozoic supercontinent Rodinia. Such a tectonic reconstruction means that the Willyama Complex in Australia, which contains the supergiant Broken Hill deposit (280 Mt of 10.0% Pb, 8.5% Zn and 148 g/t Ag, Parr & Plimer, Reference Parr, Plimer, Kirkham, Sinclair, Thorpe and Duke1993; Spry & Teale, Reference Spry and Teale2021), connects with the Yavapai belt. Given the enormous size of Broken Hill and the Jerome district, the Paleoproterozoic of Colorado is considered to have potential for the presence of large massive sulphide deposits.
While the aforementioned deposits in Wisconsin and Arizona are unmetamorphosed or were metamorphosed to the lower greenschist facies, some small deposits in Arizona (e.g. Antler and Copper World Cu-Zn-Pb VMS deposits in the Hualapai Mountains; Stensrud & More, Reference Stensrud and More1980; Donnelly et al. Reference Donnelly, Conway and Earhart1987) were metamorphosed to the middle amphibolite facies. Here, we evaluate the genesis of small metamorphosed massive sulphide deposits in south-central Colorado, the largest of which is Sedalia (1.25 Mt @ 3.3% Cu, 5.6% Zn, 23 g/t Ag and 0.3 g/t Au; Heinrich, Reference Heinrich1981). Previous genetic models for the formation of these deposits have been the subject of debate and include VMS (e.g. Drobeck, Reference Drobeck, Epis and Callender1981; Sheridan & Raymond, Reference Sheridan and Raymond1984a; Alers & Shallow, Reference Alers and Shallow1996; Shallow & Alers, Reference Shallow and Alers1996), carbonate-replacement skarn (e.g. Salotti, Reference Salotti1965), BHT (Zephyr Minerals Limited, 2018; https://www.zephyrminerals.com/dawson-section), and most recently, high-temperature fractionation of base and precious metals from peraluminous granitoids (Kleinhans & Swan, Reference Kleinhans and Swan2022).
Although more than 20 Paleoproterozoic sulphide occurrences were identified by Sheridan and Raymond (Reference Sheridan and Raymond1984a) in north-central and south-central Colorado, we primarily focus, from west to east, on the Vulcan-Good Hope, Cinderella, Cotopaxi, Green Mountain, El Plomo, Horseshoe, and Dawson deposits. The last four deposits occur in a linear belt near Cañon City that we refer to as the Dawson-Green Mountain trend (DGMT). In this contribution, we evaluate the geological setting along with the major and trace element studies of various rock types (including metamorphosed hydrothermally altered rocks and granitoids), the compositions of minerals in the sulphide-bearing units and attendant alteration, and the sulphur isotope ratios of sulphides in various deposits. The present contribution complements previous studies on aspects of the geology of these occurrences, including the descriptive geology of the deposits by Sheridan and Raymond (Reference Sheridan and Raymond1984a, Reference Sheridan and Raymond1984b), the genetic relationship of gahnite to sulphide mineralization (Heimann et al. Reference Heimann, Spry and Teale2005), the origin of gedrite-garnet-cordierite rocks at the Evergreen prospect (Heimann et al. Reference Heimann, Spry, Teale and Jacobson2006), and the genetic relationship of nodular sillimanite rocks to sulphide mineralization (Spry et al. Reference Spry, McFadden, Teale, Alers, Shallow and Glenn2022b). The aim of the current study is to evaluate the origin of the various deposits and to provide exploration tools that can potentially be used as guides to further mineralization in regionally metamorphosed rocks in south-central Colorado.
2. Regional geology
The central-southwestern part of the United States is dominated by four crustal terranes/provinces: Wyoming, Mojave, Yavapai and Mazatzal, the first of which is Archean in age (c. 3.5–2.5 Ga: Shaw & Karlstrom, Reference Shaw and Karlstrom1999; Jones et al. Reference Jones, Siddoway and Connelly2010) while the other three provinces are of Proterozoic age and were accreted onto the margin of the Wyoming province. Metamorphosed massive Cu-Zn-Au-(Pb-Ag) deposits extend in a discontinuous belt from the Wet Mountains, in central southern Colorado, to the Independence Mountains, near the Colorado–Wyoming border in a package of metasedimentary, granitoids and bimodal metavolcanic rocks (Fig. 1). These deposits primarily occur in the Yavapai province and the so-called “transition zone” that is ∼ 300 km wide and occurs between the Yavapai and Mazatzal provinces (Fig. 2). The Yavapai province is a coalescence of juvenile arc terranes that formed around 2.0−1.8 Ga, while the Mazatzal Province, with a Nd model age of ∼1.8−1.7 Ga (Bennet & DePaolo, Reference Bennett and DePaolo1987), is considered to be a microcontinent that was accreted onto the southern margin of the Yavapai province during the Mazatzal orogeny at around 1.65−1.60 Ga (Condie, Reference Condie1982; Karlstrom, Reference Karlstrom1998; Karlstrom & Humphreys, Reference Karlstrom and Humphreys1998; Anderson & Cullers, Reference Anderson and Cullers1999; Whitmeyer & Karlstrom, Reference Whitmeyer and Karlstrom2007; Grambling et al. Reference Grambling, Holland, Karlstrom, Gehrels and Pecha2015). The transition zone marks the location of the Yavapai–Mazatzal suture and is characterized by strong deformation (Karlstrom, Reference Karlstrom1998; Karlstrom & Humphreys Reference Karlstrom and Humphreys1998; Shaw & Karlstrom, Reference Shaw and Karlstrom1999). Extensional tectonic regimes are associated with terrane accretion and the development of ductile shear zones. The study area is characterized by isoclinal folds, while the second and third deformation events formed large open folds. The major structures generally trend northwest to north.

Figure 1. General map of Colorado showing the extent of Proterozoic rocks (grey shaded pattern; after Sheridan and Raymond, 1984), terrane boundaries (after Shaw and Karlstrom, Reference Shaw and Karlstrom1999), and location of metamorphosed massive sulphide deposits: 1 Bon Ton, 2 Cinderella, 3 Sedalia, 4 Ace High/Jackpot, 5 Independence, 6 Betty (Lone Chimney), 7 Cotopaxi, 8 Green Mountain, 9 Dawson-Grape Creek trend, 10 Marion, 11 Amethyst, 12 Evergreen hydrothermal alteration zone, Cresswell, F.M.D., and Hosa Lodge, and 13: Caprock. The location of the Mazatzal Deformation Front is derived from Shaw and Karlstrom (Reference Shaw and Karlstrom1999). Figs. 2, 3 are shown as insets.

Figure 2. Regional geologic map of the southwestern United States. Major crustal provinces, transition zones, inferred boundaries and deformation fronts are also delineated (modified after Jones et al. Reference Jones, Siddoway and Connelly2010). An inset map showing the study area (see Fig. 1) is also indicated.
Granitoids are widespread throughout the Proterozoic terrane in Colorado and were emplaced from 1.9 to 1.1 Ga during three episodes (e.g. Bickford et al. Reference Bickford, Cullers, Shuster, Premo, Van Schmus, Grambling and Tewksbury1989; Anderson & Cullers, Reference Anderson and Cullers1999; Siddoway et al. Reference Siddoway, Givot, Bodle and Heizler2000). Major Paleoproterozoic calc-alkaline granitoids were intruded at 1.9 to 1.7 Ga granitic with more peraluminous plutons occurring at ∼1.7 Ga (Anderson & Cullers, Reference Anderson and Cullers1999; Premo & Fanning, Reference Premo and Fanning2000). Granitic plutonism subsequently occurred at ∼ 1.45 to 1.35 Ga with the emplacement of A-type granitic batholiths (e.g. Peterman et al. Reference Peterman, Hedge and Braddocck1968) followed by a later period of A-type intrusions (or ferroan granitoids using the terminology of Frost & Frost, Reference Frost and Frost2011), which was associated with the formation of the Pikes Peak batholith at around 1.1 Ga (Hedge, Reference Hedge1970). Metamorphosed massive sulphide deposits are spatially associated with the first generation of granitoid emplacement around the Gunnison and Salida areas (Sheridan & Raymond, Reference Sheridan and Raymond1984a, Reference Sheridan and Raymond1984b), while the ages of those spatially associated with deposits in the DGMT are unknown due to the absence of geochronological studies. Nonetheless, Pb-Pb isotope ages of galena that were analysed by Bruce Doe in the 1970s and reported in Sheridan and Raymond (Reference Sheridan and Raymond1984a, Reference Sheridan and Raymond1984b) suggest that sulphide mineralization formed between 1.8 and 1.7 Ga. Z. Palmer (unpub. M.S. thesis, Colorado School of Mines, 2019) showed that the small Isabel and Amethyst Cu-Zn deposits in the southern Wet Mountains were spatially and genetically associated with the A-type San Isabel granite (U-Pb age of monazite of 1.37 to 1.13 Ga) and are younger than the other metamorphosed massive sulphide deposits in Colorado.
Rocks in central-southern Colorado were mostly metamorphosed to the middle to upper amphibolite facies, similar to the metamorphic grade observed in Proterozoic rocks of northern Colorado and New Mexico (e.g. Pedrick et al. Reference Pedrick, Karlstrom and Bowring1998; Shaw & Karlstrom, Reference Shaw and Karlstrom1999). However, upper greenschist–lower amphibolite facies conditions were reported by Sheridan et al. (Reference Sheridan, Raymond, Cox, Epis and Callender1981) for the Gunnison district in the west, while upper amphibolite-granulite facies conditions occur in the southern Wet Mountains spatially associated with the Isabel and Amethyst deposits (Sheridan & Raymond, Reference Sheridan and Raymond1984b). Steep gradients between greenschist to upper amphibolite conditions occur in localized areas (e.g. 25 km west of Cañon City; Shaw & Karlstrom, Reference Shaw and Karlstrom1999, Fig. 2, p. 42) and is likely related to pluton-enhanced metamorphism and possible composite effects of superimposed metamorphic events. Although conditions mostly reflect regional metamorphism associated with the Yavapai orogeny (the Colorado orogeny of Sims & Stein, Reference Sims and Stein2003 at 1.78 to 1.69 Ga), localized reheating and superimposed metamorphism (primarily to the amphibolite facies) is also associated with the Mazatzal orogeny (the Berthoud orogeny of Sims & Stein, Reference Sims and Stein2003) at ∼ 1.45 to 1.35 Ga (e.g. Möller et al. Reference Möller, Berndt, Walker and Kelly2022).
3. Analytical methods
3.a. Whole rock analyses
Most bulk rock compositions of samples (n = 53) were analysed by Bureau Veritas, Vancouver, British Columbia using analytical package LF202. Major oxides (n = 11), loss-on-ignition, and the following trace elements (Ag, As, Au, Ba, Be, Bi, Cd, Co, Cs, Cu, Ga, Hf, Hg, Mo, Nb, Ni, Pb, Rb, Sb, Sc, Se, Sn, Sr, Ta, Th, Tl, U, V, W, Y, Zn and Zr; plus the rare earth elements (REEs) (Ce, Dy, Er, Eu, Gd, Ho, La, Lu, Nd, Pr, Sm, Tb, Tm and Yb) were prepared by lithium borate fusion and analysed by inductively coupled plasma-atomic emission spectroscopy (ICP-AES)/inductively coupled plasma mass spectrometry (ICP-MS) and X-ray fluorescence techniques. The detection limits for the oxides are 0.01 wt.%, except for Cr2O3, which is 0.002 wt.%, while those for most of the trace elements using this technique, but not including the REEs, range from 0.1 to 0.5 ppm, except for Ba, Be and Sc, which have detection limits of 1 ppm. The detection limits for Ni and Sn are 20 and 8 ppm, respectively, while the REEs have detection limits ranging from 0.01 to 0.1 ppm. Total sulphur and carbon were analysed with a Leco instrument, with detection limits of 0.02 wt.%. The following trace elements Ag, As, Au, Bi, Cd, Cu, Hg, Mo, Ni, Pb, Sb, Se, Tl and Zn were digested in aqua-regia and analysed by ICP-AES and ICP-MS techniques. The detection limits for these elements varied from 0.1 to 1 ppm, depending on the element.
Fifteen samples were analysed by Actlabs, Ancaster, Ontario. Major oxides (n = 11) plus the same trace elements noted above except, for Cr, Hg and Sc, were analysed by ICP-optical emission spectrometry, instrumental neutron activation and ICP-MS. Sample fusion employed a lithium metaborate/tetraborate technique. The detection limits for the oxides are 0.01 wt.%, except for MnO and TiO2, which are 0.001 wt.%. REEs had detection limits of 0.005 to 0.1 ppm, while the remainder of the elements have the following detection limits: Bi (0.4 ppm), Ag, Cs, and Sb (0.5 ppm), Be, Co, Ga, Ge, Nb, Sc, Sn, W, and Y (1 ppm), Mo, Rb, Sr, and Zr (2 ppm), As, Pb, and V (5 ppm), Cu (10 ppm), and Zn (30 ppm).
3.b. Electron microprobe analyses
Samples were studied petrographically, and the compositions of silicates and oxides were obtained using an ARL-SEMQ electron microprobe in the Department of Geological and Atmospheric Sciences at Iowa State University and a JEOL JXA-8530FPlus Electron Probe Microanalyzer at the University of Minnesota. Analyses of silicates for both electron microprobes were conducted using a 15-kV accelerating voltage with a 20-nA beam current, a 1–2 μm spot size. Mineral standards included hornblende (Si, Al, Mg and Ca), ilmenite (Ti, Fe), albite (Al, Na), spessartine (Al, Mn), pyrope (Si, Mg), K-feldspar (K), gahnite (Zn, Al), tugtupite (Cl) and apatite (F). Quantitative analyses of sulphides were obtained under two sets of conditions for the analyses of sphalerite from the Vulcan, Horseshoe, El Plomo, and Dawson deposits to determine Zn/Cd ratios and for the analyses of galena, pyrrhotite, and argentopyrite from the Horseshoe and El Plomo deposits. Both sets of analyses were performed at the University of Minnesota with the samples of sphalerite for the determination of Zn/Cd ratios using the following analytical conditions: an accelerating voltage of 15 kV, a beam current of 50 nA and a beam diameter of 5 microns. Element compositions were acquired using analysing crystals LIFL for Zn kα, Mn kα, Fe kα, Cu kα, PETL for Cd lα, and PETJ for S kα and Ag lα. The standards were Mn-olivine and synthetic and Mn2SiO4 for Mn, Cu metal for Cu, pyrite for Fe, sphalerite for Zn and S, hessite for Ag, and cadmium sulphide (CdS) for Cd. The on-peak counting time was 10 seconds for Zn kα, Mn kα, Fe kα, Cu kα, S kα, 40 seconds for Ag lα and 60 seconds for Cd lα. The analyses of sulphides from the Horseshoe and El Plomo deposits used the same operating conditions but also analysed for Pb, As, Se, Sb, Au and Bi, using the following standards: galena for Pb and S, sphalerite for Zn and S, indium arsenide for As, as well as native metals for Ag, Au, Bi, Sb and Se. The on-peak counting time was 10 seconds for all elements. The mean atomic number (MAN) background intensity method was used instead of the traditional off-peak background acquisition (Donovan & Tingle, Reference Donovan and Tingle1996; Donovan et al. Reference Donovan, Singer and Armstrong2016). The MAN background intensity data were calibrated, and continuum absorption corrected for Cd lα, Zn kα, Mn kα, Fe kα, Cu kα, S kα and Ag lα. Unknown and standard intensities were corrected for dead time. The Phi-Rho-Z matrix correction algorithm Armstrong/Love Scott (CitZAF) was used along with the mass absorption coefficients dataset FFAST (Chantler et al. Reference Chantler, Olsen, Dragoset, Kishore, Kotochigova and Zucker2005).
3.c. Scanning electron microscopy
Images and energy-dispersive X-ray spectroscopy (EDS) point analyses of sulphides and sulphosalts were obtained on a JEOL JSM-IT100 InTouch scanning electron microscope (SEM) using the backscatter electron composition (BED-C) mode at Iowa State University. The probe current ranged between 65 and 75nA with a low vacuum pressure of approximately 100 Pa and an accelerating voltage of 10–20 kV.
3.d. Sulphur isotope analyses
Pyrite, pyrrhotite, chalcopyrite and sphalerite were separated from samples by hand-picking under a binocular microscope or were drilled out using a Dremel tool with a 1-mm drill tip. We followed the procedure for sulphur isotope analysis as described by Grassineau (Reference Grassineau2006) and Spry et al. (Reference Spry, Mathur, Teale and Godfrey2022a). The mineral concentrates were pulverized in an agate mortar to a powder, which weighed between ∼0.75 and 3.0 mg depending on the composition of the sulphide (chalcopyrite: ∼1.5 mg, galena: ∼3.0 mg, pyrite: ∼0.75 mg and pyrrhotite ∼0.75 mg, sphalerite ∼1.5 mg). The powder was loaded into tin capsules and burned using a Thermo Scientific Flash IRMS IsoLInk elemental analyser. The tin capsules were oxidized at ∼1020 °C and when oxygen was added it flash combusted at 1800 °C (Grassineau, Reference Grassineau2006). The oxygen was added at a rate of 300 ml/minute for 3 seconds. The SO2 gas produced was purified through a gas chromatography column and then introduced via a Conflo IV Universal Interface system into a continuous flow-type dual-inlet ThermoScientific Delta V Series Isotope Ratio mass spectrometer under He flow. The analysis time was ∼ 420 seconds. The δ34SVCDT values were calculated using calibration curves obtained for seven internal standards obtained from the Queen’s Facility for Isotope Research (QFIR) at Queen’s University, Canada, and two internal standards provided by Thermo Scientific (see Spry et al. Reference Spry, Mathur, Teale and Godfrey2022a). These standards are Q-GEMA pyrite = +3.0‰, NBS IAEA-SO-6 barite = −34.1‰, 127 barite = +20.3‰ (±0.3‰), IAEA-SO-5 barite = +0.5‰, MRC pyrite = +0.7‰, QFIR pyrite = −0.5‰, M6801 barite = +12.5‰, and peat = −13.15‰ (±0.3‰), and sulphanilimide = −1.24‰ (±0.2‰). The isotopic values of the standards are relative to the internationally recognized sulphur isotope standard Cañon Diablo troilite (FeS). The analytical precision of the data is ±0.1‰.
4. Local geology of mineral deposits
Although samples were obtained from over 15 massive sulphide prospects, the focus here is on the low metamorphic grade Vulcan-Good Hope deposits (upper greenschist–lower amphibolite facies) to potentially see through the camouflaging effects of metamorphism and associated deformation, as well as the Cinderella-Bon Ton and Cotopaxi deposits and those that are currently the subject of recent exploration activity along the DGMT (Green Mountain, El Plomo, Horseshoe, Dawson), which were metamorphosed to the upper amphibolite facies. The geological characteristics of the massive sulphide deposits are in Table 1. Although nodular sillimanite rocks and gahnite-bearing rocks are spatially associated with several deposits listed in Table 1, they were the focus of studies by Spry et al. (Reference Spry, McFadden, Teale, Alers, Shallow and Glenn2022b) and Heimann et al. (Reference Heimann, Spry and Teale2005), respectively, and will only be discussed here as they relate to the origin of the deposits and their potential as exploration guides to metallic mineralization. Note we use the term ‘gahnite’ here for zinc-bearing spinels regardless of the Zn-Fe-Mg proportion, which was shown by Heimann et al. (Reference Heimann, Spry and Teale2005) to be variable in composition for deposits in Colorado.
Table 1. Summary of geological characteristics of selected metamorphosed massive sulphide deposits, Colorado

Abbreviations after Warr (Reference Warr2021);
* Metamorphosed to the upper amphibolite facies;
** Metamorphosed to the upper greenschist–lower amphibolite facies.
4.a. Vulcan-Good Hope
The Vulcan-Good Hope deposits, which extend for a distance of ∼500 m, were the largest gold producers in the Dubois Greenstone Belt and likely part of the same hydrothermal system. The belt consists of clastic sediments, andesite to basalt flows and tuffs, rhyolite, and chert metamorphosed to the upper greenschist–lower amphibolite facies along with syn- and post-tectonic granitoids (Drobeck, Reference Drobeck, Epis and Callender1981; Sheridan et al. Reference Sheridan, Raymond, Cox, Epis and Callender1981; Hartley, Reference Hartley1983) (Fig. 3). The deposits occur in felsic metavolcanic rocks, which Drobeck (Reference Drobeck, Epis and Callender1981) interpreted as dacite to rhyolite flows, tuffs and tuffaceous sediments interleaved with metabasalt and meta-andesite. Metamorphosed sediments (greywackes, siltites and argillites) are also locally present as is magnetite-bearing quartzite, which occurs along strike from the sulphide zone. The deposits are conformable to sedimentary layering and surrounded by an envelope of quartz-sericite pyrite alteration (Fig. 4a), which in turn is surrounded by a halo of quartz-chlorite alteration (Drobeck, Reference Drobeck, Epis and Callender1981). The dominant sulphides are pyrite, pyrrhotite and sphalerite (Fig. 4b), with chalcopyrite, arsenopyrite and galena occurring in minor amounts. The sulphides show crude banding parallel to the conformable schistosity and sedimentary layering. Of note is the presence of a superimposed Au-Ag-Te-S-Sb-Bi-As-Hg-Se system on the massive sulphides which Drobeck (Reference Drobeck, Epis and Callender1981) suggested may have formed during the Miocene. However, timing relationships are unclear, and it is possible that this superimposition occurred during the Proterozoic. The overprinted mineralization is dominated by tellurium-bearing species in chalcedonic veinlets, which consist of native Te crystals, tellurite, coloradoite, tetradymite, tellurobismutite, petzite, sylvanite and frohbergite. The Vulcan-Good Hope system is the type locality for the relatively rare Cu tellurides (rickardite, weissite, cameronite, spirodonite and vulcanite) and zincmelanterite (Eckel, Reference Eckel1961; Cameron & Threadgold, Reference Cameron and Threadgold1961; Drobeck, Reference Drobeck, Epis and Callender1981; Hartley, Reference Hartley1983).

Figure 3. Geological map of the Gunnison district showing the location of the Vulcan-Good Hope deposits (V), along with the Gunnison (G), Iron Cap (I), Denver City (D) and Yukon-Alaska (Y) deposits (modified after Drobeck Reference Drobeck, Epis and Callender1981).
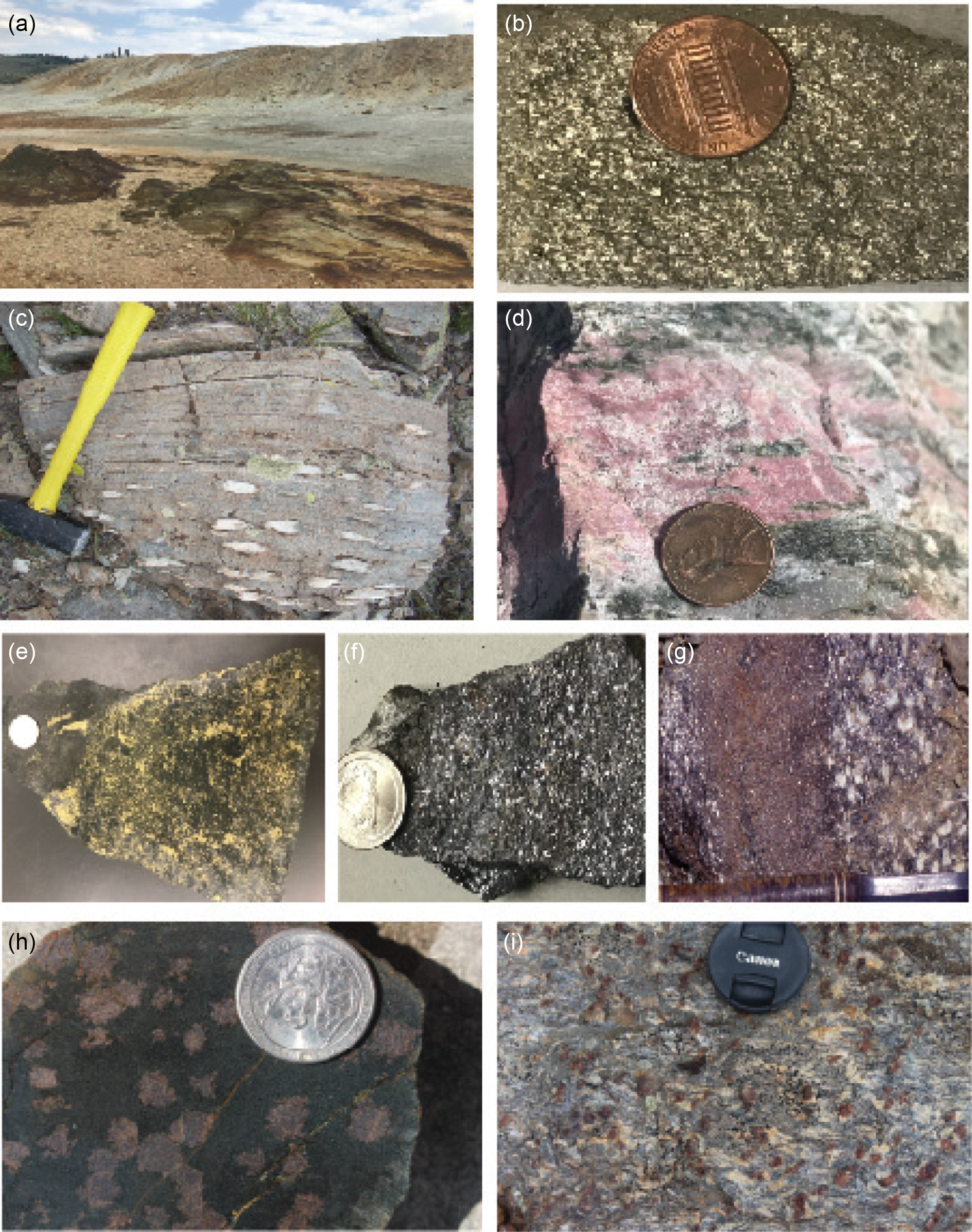
Figure 4. Sulphide samples, host rocks, metamorphosed altered rocks associated with the Vulcan, Bon Ton-Cinderella, Cotopaxi and Green Mountain deposits. (a) View of the tailings pile surrounding the Vulcan deposit. In the foreground are outcrops of oxidized pyrite-bearing quartz-muscovite schists; (b) massive pyrite-pyrrhotite-sphalerite ore from the Vulcan deposit; (c) nodular sillimanite rock grading into quartz-feldspar-biotite gneiss spatially related to the Cinderella deposit; (d) rhodonite-actinolite-quartz alteration spatially related to nodular sillimanite rock surrounding the Cinderella deposit; (e) semi-massive chalcopyrite from the Cotopaxi deposit; (f) massive sphalerite from the Cotopaxi deposit; (g) anthophyllite-cordierite-biotite gahnite rock surrounded by nodular sillimanite rock (Cotopaxi deposit); (h) garnet amphibolite spatially associated with the Green Mountain deposit; and (i) quartz-garnet-plagioclase-biotite rock spatially associated with the Green Mountain deposit. Note that this rock resembles the so-called Potosi Gneiss (a metamorphosed rhyodacite) spatially associated with the supergiant Broken Hill Pb-Zn-Ag deposit, Australia (Stevens & Barrons, Reference Stevens and Barrons2002).
4.b. Cinderella-Bon Ton
The Cinderella deposit consists of Zn-Cu±Pb mineralization and is located 2 km south and along strike from the Bon Ton deposit (D.C. Knight, unpub. MSc thesis, Univ. Manitoba, 1981) (Fig. 5). The Cinderella deposit occurs on the south-east limb of a northeasterly plunging fold, whereas the Bon Ton deposit occurs at the closure of the fold. Isoclinal folds near the Cinderella deposit are evident. The Boulder Creek granite crops out 1.6 to 3.2 km west-northwest of the mineralized horizon.

Figure 5. Geological map of the Cinderella-Bon Ton deposits showing the extensive intermittent nodular sillimanite rock horizon (∼5 km long) that is spatially associated with sulphide mineralization in both deposits, which is hosted in biotite gneiss. Note the presence of metagabbro just to the south of the Cinderella deposit. The figure is modified after Spry et al. (Reference Spry, McFadden, Teale, Alers, Shallow and Glenn2022b).
Sillimanite-quartz-muscovite-microcline gneiss, nodular sillimanite rock (Fig. 4c), biotite-muscovite schist, garnet gneiss, calc-silicate rocks, quartz-biotite-epidote gneiss and amphibolites are spatially associated with the Cinderella prospect. Iron formation occurs near the top of the stratigraphic sequence (D.C. Knight, unpub. MSc thesis, Univ. Manitoba, 1981). J. Ray, B. Ahlers, N. Shriver, K. Hattie and J. Shallow (unpub. report to American Copper and Nickel Company, 1993) reported two mineralized zones Zn-Cu and Pb-Zn-(Cu), which contain variable proportions of sphalerite, galena, chalcopyrite and pyrite, spatially related to nodular sillimanite rock and discontinuous zones of anthophyllite-rich rock. The lithologies at Bon Ton are essentially the same as those at Cinderella with nodular sillimanite rock enveloping mineralization in both locations, where it is up to 400 m wide and extends intermittently for 8 km. It is one of the most, if not the most, extensive nodular sillimanite horizons in Colorado (Spry et al. Reference Spry, McFadden, Teale, Alers, Shallow and Glenn2022b). The ‘nodules’ are composed of quartz and sillimanite with rare microcline, garnet and/or gahnite. Also present at Bon Ton is the spatial association of iron formation and gahnite to the sulphide mineralization (Heimann et al. Reference Heimann, Spry and Teale2005).
Metamorphosed altered rocks at Bon Ton consist of almandine-biotite-cordierite-quartz-gahnite-sphalerite ± chlorite ± muscovite ± pyrite ± chalcopyrite ± pyrrhotite ± plagioclase (andesine) ± sillimanite and gahnite-biotite or phlogopite-quartz-sphalerite-pyrite-chalcopyrite-covellite ± chlorite, while those at Cinderella contain the following assemblages: actinolite-phlogopite-garnet (spessartine)-gahnite, gahnite-anthophyllite-cordierite-phlogopite-tremolite ± clinohumite ± serpentine ± sphalerite ± pyrite, gahnite-chlorite-anthophyllite-cordierite-phlogopite-sillimanite ± pyrite, phlogopite-quartz-garnet-gahnite-chlorite ± anorthite ± sillimanite and garnet-phlogopite-gahnite-quartz ± K-feldspar. In places, rhodonite-actinolite-quartz rocks occur at Cinderella (Fig. 4d).
4.c. Cotopaxi
Cotopaxi is a well-studied Zn-Cu deposit located 1.6 km northwest of the town of Cotopaxi (e.g. Lindgren, Reference Lindgren1908; Salotti, Reference Salotti1965). J. Ray, B. Ahlers, N. Shriver, K. Hattie and J. Shallow (unpub. report to American Copper and Nickel Company, 1993) noted that 1,316 t of Zn, 81 t of Cu, 70 t of Pb, 330 kg of Ag and 5 kg of Au were produced from the deposit since the 1880s. Biotite gneiss, quartz-feldspar biotite gneiss, hornblende gneiss, nodular sillimanite rock, amphibolite and pegmatitic rocks surround the sulphide mineralization in a package of rocks that is spatially associated with gneissic granite of the Boulder Creek batholith (Fig. 6). Salotti (Reference Salotti1965) and Heinrich (Reference Heinrich1981) argued that the granitic gneiss constitutes a xenolith (1.2 km by 0.8 km) that formed as a roof pendant to the Boulder Creek gneissic granite. However, whether it is a xenolith remains uncertain due to the absence of clear contacts between the metasedimentary package and the granite. Porphyroblasts of gahnite up to 3 cm in diameter occur in a variety of rock types (Heimann et al. Reference Heimann, Spry and Teale2005). Although cut by Proterozoic and Laramide faults, there is little evidence of local folding despite the presence of a dominant schistosity in the metasedimentary rocks. The main metallic minerals consist of pyrite, pyrrhotite, chalcopyrite (Fig. 4e), sphalerite (Fig. 4f), gahnite and magnetite with minor amounts of molybdenite.

Figure 6. Geological map of the Cotopaxi deposit showing that the deposit is hosted in metasedimentary rocks and spatially associated with nodular sillimanite rocks and ‘amphibolite’ (anthophyllite-cordierite-biotite-gahnite altered rocks). The figure is modified after Salotti (Reference Salotti1965) and Spry et al. (Reference Spry, McFadden, Teale, Alers, Shallow and Glenn2022b).
At Cotopaxi, a variety of metamorphosed altered rocks and ore-bearing assemblages are associated with sulphides, including anthophyllite-phlogopite-cordierite-gahnite ± rutile, actinolite-forsterite-gahnite-chalcopyrite-galena-sphalerite ± pyrrhotite ± pyrite ± phlogopite ± magnetite ± clinohumite ± ilmenite ± covellite ± molybdenite, quartz-phlogopite-gahnite-sphalerite-galena-magnetite-rutile ± chalcopyrite ± pyrrhotite ± molybdenite, cummingtonite-quartz-biotite-muscovite ± diopside ± olivine ± gahnite ± clinohumite± rutile ± sillimanite ± garnet ± plagioclase, K-feldspar ± zircon ± chalcopyrite ± galena ± sphalerite ± pyrite ± pyrrhotite ± molybdenite ± magnetite ± ilmenite and hornblende-quartz-biotite-ilmenite ± garnet ± plagioclase ± cordierite ± gahnite ± epidote ± chalcopyrite ± sphalerite. These altered rocks locally occur in contact with nodular sillimanite rock (Fig. 4g).
4.d. Dawson-Green Mountain trend
4.d.1. Green Mountain
The Green Mountain Cu-Zn deposit, located in the Wet Mountains approximately 20 km southwest of Cañon City, occurs in migmatitic, quartz-feldspar-biotite and quartz-cordierite-biotite gneisses. Granodiorite of the Boulder Creek intrusion crops out 1.6 km northeast of the mine. G.T. Ririe (unpub. PhD thesis, Univ Iowa, 1981) reported P-T conditions of 550o to 680°C and 4 kbar for rocks near the Green Mountain mine based on the stability of quartz, K-feldspar, Ca-rich plagioclase, hornblende, and minor sillimanite, biotite, almandine, and muscovite. Assuming a pressure of 4 kb, garnet-biotite geothermometry applied by A. Heimann (unpub. MS thesis, Iowa State Univ., 2002) to samples of garnet-biotite schist yields a temperature range of 510–628°C, which overlaps the temperature range estimated by G.T. Ririe (unpub. PhD thesis, Univ. Iowa, 1981).
Although the reserves of the deposit are unknown, a drilling programme by Phelps Dodge between 1979 and 1994 intersected two sulphide horizons; an upper zone of 2.3% Cu and 0.2% Zn over 1.2 to 3.3 m, and a lower zone of 18.1% Cu and 4.3% Zn over 1.5 m, which parallels sedimentary layering and a strong foliation identified by J. Ray, B. Alers, N. Shriver, K. Hattie and J. Shallow (unpub. report to American Copper and Nickel Company, 1993). Host rocks to ore are composed of nodular sillimanite-garnet±cordierite rock, quartz-garnet gneiss, anthophyllite-cordierite-gahnite rock, garnet amphibolite (Fig. 4h), garnet-sillimanite-feldspar gneiss (Fig. 4i), quartz-garnet rock (Fig. 7a), hornblende-quartz-biotite gneiss, quartz-biotite gneiss, garnet-bearing pegmatite, iron formation, biotite gneisses and minor calc-silicate gneiss. The garnet amphibolite is composed of garnet, hornblende, quartz, plagioclase and ilmenite. Metallic minerals consist of pyrite, pyrrhotite, sphalerite, chalcopyrite, gahnite and magnetite, with gangue minerals being dominated by biotite, anthophyllite, cummingtonite and clinopyroxene. Sulphides are generally disseminated in metamorphosed altered rocks consisting of anthophyllite-cummingtonite-gahnite-garnet ± pigeonite ± hornblende, as well as in gabbroic rocks (Fig. 7b).
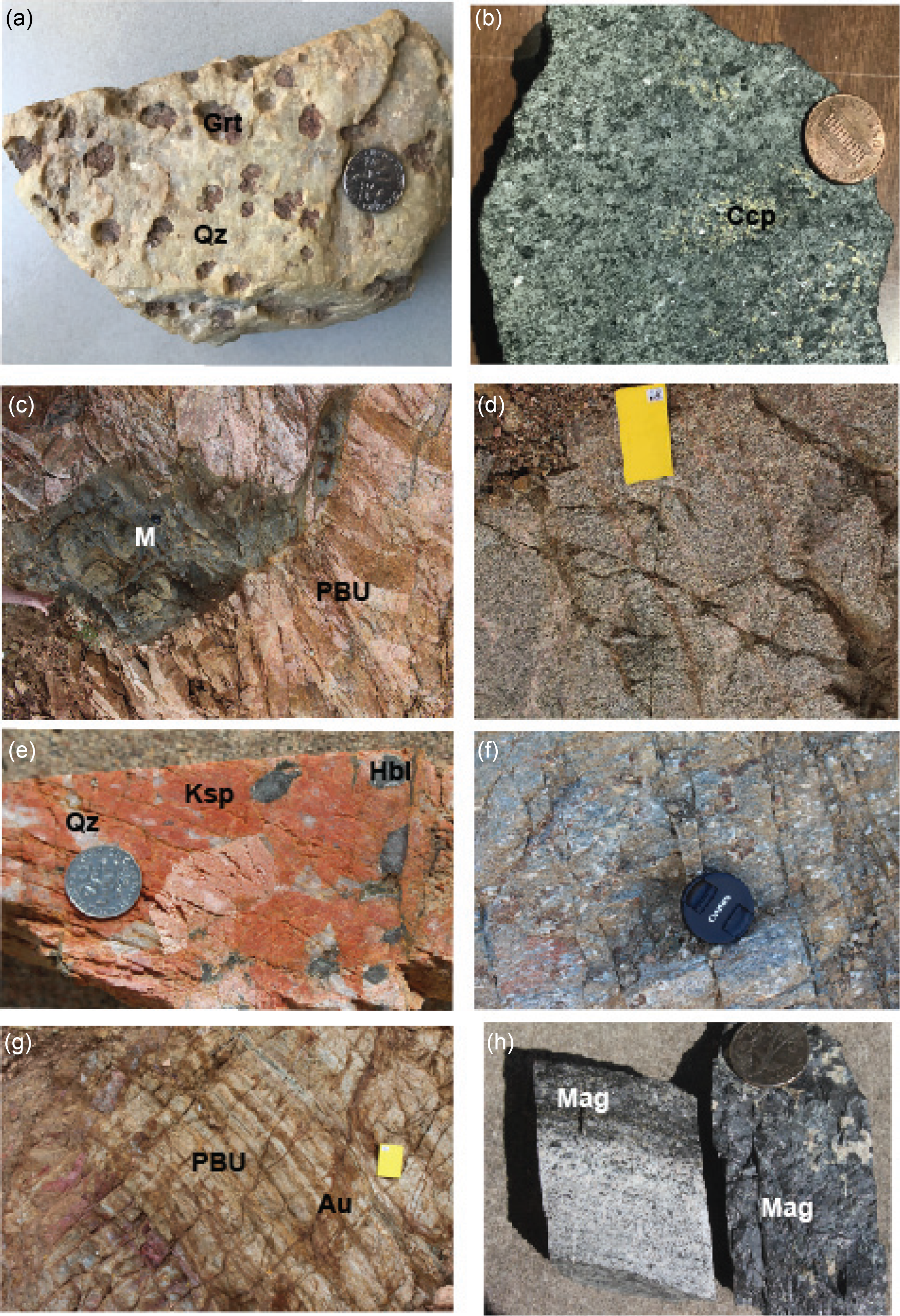
Figure 7. Rock samples from the Green Mountain and Dawson deposits. (a) Quartz (Qz)-garnet (Grt) exhalative/inhalative rock spatially associated with the Green Mountain deposit; (b) metamphibolite containing sphalerite and chalcopyrite (Ccp) from the Green Mountain deposit; (c) block of metasedimentary rock (M) in footwall pink granite (PBU) adjacent to the Dawson deposit. (d) monzodiorite from the hanging wall of the Dawson deposit; (e) coarse-grained alkali granite/pegmatite spatially associated with the Dawson deposit. Minerals are quartz (Qz), K-feldspar (Ksp) and hornblende (Hbl); (f) Quartz-garnet-plagioclase-biotite rock spatially associated with the Dawson deposit. Note this rock resembles that associated with the Green Mountain deposit (see Fig. 4i); (g) Pink banded unit (PBU) with late-stage cross-cutting gold-bearing veins (up to 92 g/t); (h) Banded quartz magnetite (Mag) rock (possible exhalative/inhalative rock) (left) and massive magnetite (right) in core from the Dawson deposit.
4.d.2. Grape Creek trend
At least 30 minor prospects occur in the vicinity of Grape Creek in a linear trend that is the western extension of the Dawson area. The sheared package of metasedimentary rocks (up to ∼120 m wide), consists mainly of quartz-K-feldspar-biotite±cordierite±sillimanite±garnet rocks sandwiched between pink banded granite, which contains amphibolite sills, and biotite-quartz monzodiorite. The two largest prospects, west of Dawson, are Horseshoe and El Plomo, both of which contain massive sulphides in anthophyllite-rich altered rocks. The massive sulphides consist of pyrite, pyrrhotite, chalcopyrite, sphalerite, magnetite and gahnite, with galena present at El Plomo. The presence of this galena reflects a metallic zonation along the Grape Creek trend with more Cu-Zn mineralization to the east and Pb-Zn-Cu to the west. Although galena is a common repository of Ag in ore deposits (e.g. Both & Stumpfl, Reference Both and Stumpfl1987), galena from El Plomo only contains up to 0.17 wt.% Ag and 0.41 wt.% Bi. Instead, Ag at El Plomo is contained in tetrahedrite (up to 4 wt.% Ag) and the rare mineral argentotetrahedrite, which contains up to 34 wt.% Ag (Table 2).
Table 2. Compositions of selected sulphides and sulphosalts

* Argentotetrahedrite.
4.d.3. Dawson
The Dawson deposit occurs at the eastern end of the DGMT, where massive sulphides and gold mineralization are hosted in a narrow package of metasedimentary rocks (up to 50 m wide) adjacent to various granitoids (Fig. 8). A resource of ∼4,250 kg Au was identified at a grade of 5 g/t Au (Zephyr Minerals, https://www.zephyrminerals.com/dawson-section). The metasedimentary package, which extends intermittently for over 5 km between Dawson and the eastern end of the Grape Creek segment, is sheared and faulted and consists of quartz-biotite-garnet (almandine) gneiss and quartz-biotite-muscovite-garnet±sillimanite±cordierite±K-feldspar schists. The metasedimentary rocks also contain minor amounts of sulphides (pyrite, chalcopyrite, pyrrhotite and rare galena), gahnite, apatite and magnetite, with rare native gold (K. Wilson, unpub. report to U.S. Borax Ltd., 1982). To the north of the metasedimentary package is a pink banded gneiss (Fig. 7c) (herein referred to as the pink banded unit), which is composed of pink-orange K-feldspar, quartz, and biotite with trace magnetite, pyrite, and hematite. It has a gradational contact with the metasedimentary package. Biotite-quartz monzonite/diorite (Fig. 7d) occurs on the southern side of the metasedimentary rocks and locally crosscuts it and the pink banded gneiss. The biotite-quartz monzonite/diorite is medium to coarse-grained and contains minor to trace amounts of magnetite, K-feldspar, serpentine, epidote, pyrite, chalcopyrite, amphibole and magnetite. Locally, this rock occurs as inclusions in biotite-K-feldspar gneiss.

Figure 8. Geological map of the Dawson-Grape Creek trend. The Dawson area is subdivided into the Windy Point, Windy Gulch and Dawson segments. The so-called Grape Creek trend contains at least 30 minor prospects, the largest of which are Horseshoe and El Plomo. Note that the mineralized occurrences all occur in a narrow horizon of metamorphosed altered metasedimentary rocks between various granitoids. Part of the mineralized zone and host metasedimentary rocks were sheared and faulted.
Both granitoids (pink banded gneiss and biotite-quartz monzodiorite/diorite) are considered to have formed during the Yavapai orogeny based on granitoid ages, fabrics and mineralogical similarities elsewhere in the northern Wet Mountains (e.g. Siddoway et al. Reference Siddoway, Givot, Bodle and Heizler2000). However, the age of the granitoids spatially associated with Dawson deposit is unknown due to the absence of geochronological studies. Other granitoids include an alkali granite (Fig. 7e) consisting of quartz, K-feldspar, biotite, and lesser amounts of magnetite and plagioclase just east of Dawson at Windy Point in addition to a volumetrically insignificant white coloured leucogranite. Enclaves of metasedimentary rocks occur in the pink banded unit suggesting that the metasedimentary rocks are older than the granitoid (Fig. 7c). Within the metasedimentary package are localized occurrences of quartz-garnet-sillimanite-feldspar gneiss (Fig. 7f) that resembles a rock spatially associated with the Green Mountain prospect (Fig. 4i). The mineralized zone is sheared and banded (Fig. 7g) and locally contains laminated iron formation and massive magnetite (Fig. 7h).
Mafic rocks include amphibolite, metagabbro and andesite dikes. Although uncommon, amphibolite dikes locally cross-cut quartz-garnet-biotite gneiss, while andesite dikes (up to 10 m wide) cut most rocks in the Dawson area. Small outcrops of metagabbro are present and has been intersected in drill core as sill-like bodies, where it contains inclusions of various gneisses (K. Wilson, unpub. report to U.S. Borax Ltd., 1982). It contains varying proportions of biotite, hornblende, plagioclase and chlorite, with trace amounts of pyrite, pyrrhotite and chalcopyrite.
The rocks were folded during three deformation episodes. The first fold event (F1) consists of isoclinal folds that shows a strong penetrative fabric, while F2 folds consist of tight asymmetrical folds that plunge in a S-SW direction. The third fold (F3) event consists of large regional scale horizontal folds.
There are three styles of mineralization at Dawson: polymetallic massive sulphide mineralization, disseminated gold mineralization and a late-stage gold overprint. Massive sulphide mineralization varies in width from a few centimetres to up to ∼ 6 m wide and consists of, in order of abundance, pyrite, pyrrhotite, chalcopyrite and sphalerite with trace amounts of galena and marcasite in a horizon within the metasedimentary rocks. The sulphides vary from disseminated to massive (up to 50% sulphides) (Fig. 9a) within a fragmented amphibole-chlorite-biotite-talc/serpentine-quartz rock. In places, the ore is brecciated and exhibits durchbewegt textures indicating sulphides were present prior to shearing (Fig. 9b). Brecciated fragments can be several centimetres in size with sulphides locally giving the appearance of having flowed around fragments. It is enclosed in a pervasive metamorphosed altered rock that consists of biotite-garnet-anthophyllite-cordierite±gahnite±hornblende± tremolite±magnetite.
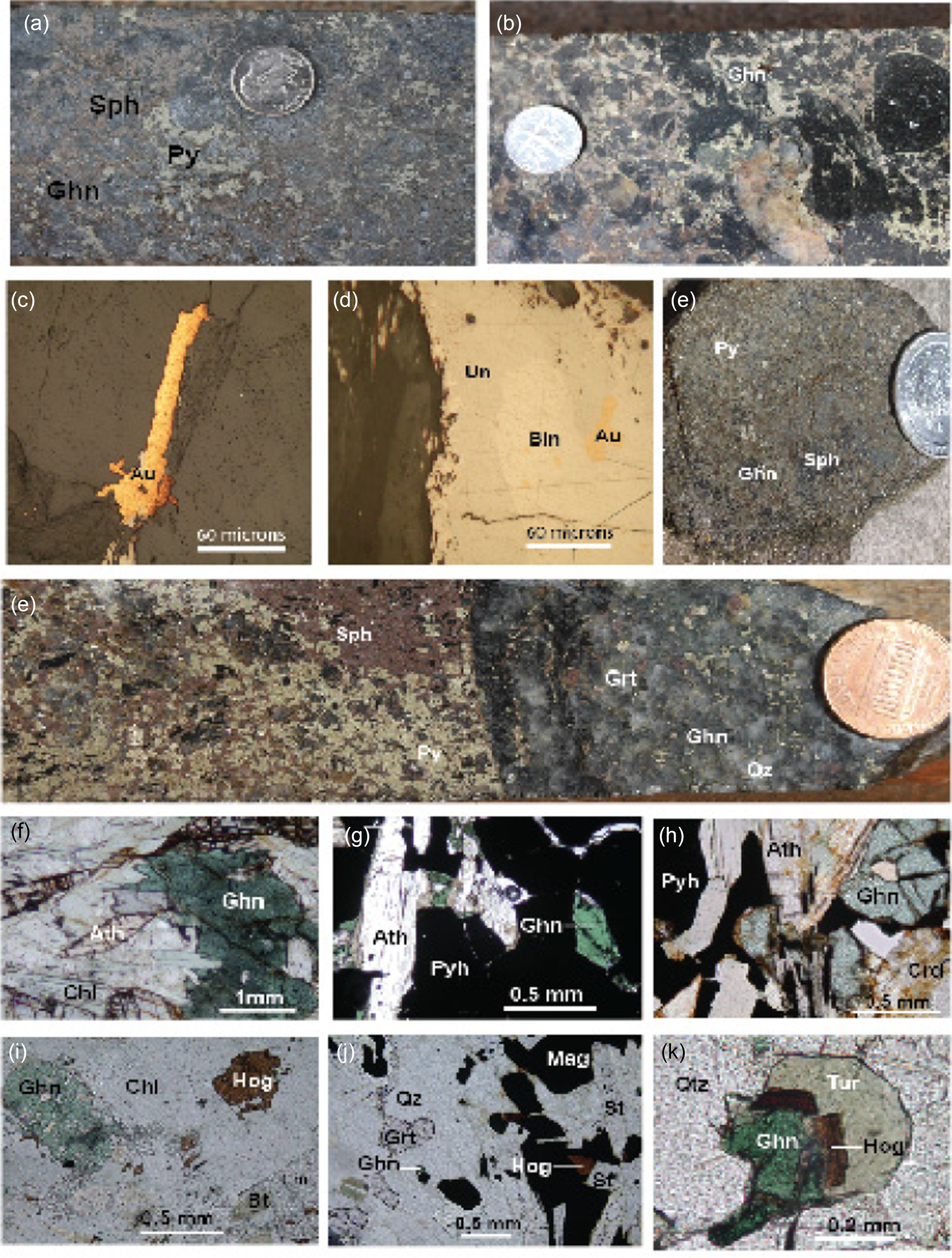
Figure 9. Ore samples and thin section micrographs of gahnite, anthophyllite/gedrite and högbomite-bearing rocks. (a) Massive sulphides containing pyrite (Py), sphalerite (Sph) and gahnite (Ghn) from the Dawson deposit. (b) Coarse gahnite in brecciated pyrite-bearing ore (Dawson deposit). (c) Veinlet of native gold (Au) in fracture in quartz (Qz) (Dawson deposit), reflected plain-polarized light. (d) Native gold (Au) along the contact between bismuthinite (Bin) and an unnamed Bi-Se-S phase (Un), reflected plain-polarized light. (e) Massive sulphides from the Horseshoe prospect consisting of pyrite (Py), sphalerite (Sph) and minr gahnite (Ghn). (f) Drill core showing massive sphalerite (Sp), pyrite (Py) on the left-hand side with quartz (Qz), pyrite (Py), gahnite (Ghn) and garnet (Grt) on the right-hand side. (g) Gahnite-anthophyllite (Ath)-chlorite (Chl) alteration spatially associated with sulphides from the Sedalia deposit. (h) Gahnite in pyrrhotite (Pyh) and in contact with anthophyllite in semi-massive ore (Green Mountain deposit). (i) Gahnite in contact with anthophyllite, pyrrhotite (Pyh) and cordierite (Crd) in disseminated sulphides (Dawson deposit), (plane-polarized light). (j) Högbomite (Hög) in gahnite-chlorite-corundum rock from the Independence deposit (plane-polarized light). (k) Högbomite in magnetite in contact with anthophyllite. Gahnite (Ghn), quartz (Qz) and garnet (Grt) occur in chlorite, plane-polarized light (Dawson deposit). (l) Inclusions of högbomite in contact with gahnite and tourmaline (Tur), plane-polarized light (Dawson deposit).
Gold mineralization occurs in a strongly sheared siliceous garnet-biotite±sillimanite gneiss generally 6 to 7 m stratigraphically below the massive sulphide horizon (assuming stratigraphy is not overturned). However, in places, it occurs in contact with the massive sulphide unit. The gold-bearing unit contains disseminated pyrite and native gold occurs as isolated grains (Fig. 9c) or native gold occurs in silicates with other minor sulphides and Bi sulphosalts.
In the late-stage gold mineralization, E. von Pechmann (unpub. report to Uranerzbergbau GMBH, 1990) reported native gold in thin veinlets of quartz and siderite as well as isolated grains in cracks in garnet, sillimanite, biotite, anthophyllite, and sericite, along with pyrite, chalcopyrite, marcasite, and galena. He also reported the strong association between Au and Bi with the following Bi minerals being identified: hammarite (Bi4Cu2Pb2S9), friedrichite (Cu5Pb5Bi7S18), joseite-B (Bi4Te2S), krupkaite (AgBiSe2) and possibly bohdanowiczite (AgBiSe2). Petrographic and SEM studies here have also identified bismuthinite (Bi2S3) and unnamed phases in the system Bi-Se-S spatially associated with native gold (Fig. 9d). Galena from Dawson contains up to 0.35 wt.% Ag and 0.44 wt.% Bi (Table 2).
5. Results
5.a. Mineral compositions of altered rocks and sulphide zones
In a study of gahnite-bearing rocks spatially associated with massive sulphide deposits in Colorado, Heimann et al. (Reference Heimann, Spry and Teale2005, Reference Heimann, Spry, Teale and Jacobson2006) obtained over 1500 electron microprobe analyses of oxides (gahnite, ilmenite and corundum) along with various silicates (i.e. biotite, amphibole, humite group minerals, garnet, cordierite, staurolite, plagioclase, chlorite, högbomite and pyroxene). Here, we focus on biotite, amphibole, garnet and gahnite, all of which are common in the metamorphosed altered rocks spatially associated with sulphides or as gangue minerals in massive sulphides in the DGMT, along with rare högbomite.
5.a.1. Amphiboles
Orthoamphiboles (gedrite and anthophyllite) and calcic amphiboles (magnesiohornblende, actinolite, tremolite and tschermakite) are common in metamorphosed altered rocks spatially associated with mineralization in the DGMT, where gedrite and anthophyllite with blades that can exceed 1 cm in length are particularly common (Fig. 9i, j). Orthoamphiboles from the Dawson, Green Mountain, El Plomo and Horseshoe deposits consist of anthophyllite and gedrite with Mg/(Mg+Fe) ratios generally ranging between 0.6 and 0.8 (Fig. 10a). These minerals are intergrown with biotite, cordierite, gahnite and disseminated metallic minerals (pyrite, pyrrhotite, chalcopyrite, sphalerite, magnetite and rare galena). Calcic amphiboles occur in the same four locations as the orthoamphiboles, as well as the small Joker prospect, just east of the Horseshoe prospect. They commonly coexist with orthoamphiboles in the same rock but are generally less abundant and are finer grained. The calcic amphiboles show a broad range of compositions and include tremolite, actinolite, magnesiohornblende and tschermakite with Mg/(Mg+Fe) ratios ranging from 0.52 to 0.97 (Fig. 10b). Analyses of amphiboles from a garnet amphibolite from the Green Mountain deposit has a lower Mg content with Mg/(Mg+Fe) ratios ranging from 0.46 to 0.48 (Table 3).

Figure 10. Binary plots of amphibole and biotite compositions from the Dawson-Green Mountain trend as a function of Mg/(Mg+Fe) vs Si. (a) Orthorhombic Mg-Fe-Mn amphiboles ((Ca+Na) <1.00; (Mg, Fe2+, Mn) ≥ 1.00). (b) Calcic amphiboles (Ca ≥ 1.50; (Na+K) <0.05. (c) Biotite compositions as a function of total Al vs Fe/(Fe+Mg).
Table 3. Representative compositions of amphibole and biotite

Ath, anthophyllite; Bt, biotite; GM, Green Mountain; Ged, gedrite; Hbl, hornblende.
5.a.2. Biotite
Biotite in metamorphosed altered rocks from the Dawson, Green Mountain and El Plomo deposits range from phlogopite to siderophyllite with Fe/(Fe+Mg) and total Al (apfu) ratios varying from 0.06 to 0.65 and 2.0 to 4.1, respectively (Fig. 10c). Biotite from the El Plomo deposit plots entirely within the phlogopite field. Halogen (F and Cl) concentrations of biotite show elevated concentrations of F in samples TVD19-22 and TVD19-25 from El Plomo that contain up to 3.24 and 2.13 wt% F, respectively, with up to 1.2 wt% F and 1.3 wt.% F also occurring in biotite from the Green Mountain (TVD19-89), and Dawson (TVD-43) deposits, respectively (Table 3). It should be noted that biotite in samples with the lowest Fe/(Fe+Mg) ratios have the highest concentrations of F, which is consistent with Fe-F avoidance in F-bearing biotite reported previously by, for example, Valley et al. (Reference Valley, Petersen, Essene and Bowman1982), Zaleski et al. (Reference Zaleski, Froese and Gordon1991) and Rosenberg et al. (Reference Rosenberg, Spry, Jacobson and Vokes2000).
5.a.3. Garnet
Garnet-bearing rocks from the Dawson area consist primarily of metamorphosed garnet-biotite-cordierite-gahnite altered rocks and in biotite-sillimanite-garnet schists while those from Green Mountain consist of garnet-bearing amphibolite (TVD19-79), quartz-biotite-garnet-gahnite rock (TVD19-84), and massive (TVD19-85) or laminated (TVD19-81, TVD19-93) garnet-quartz±biotite rocks (Fig. 7a, b). The massive and laminated garnet-bearing rocks resemble quartz garnetites spatially associated with metamorphosed Sedex, BHT and VMS deposits elsewhere (e.g. Spry et al. Reference Spry, Peters and Slack2000). Garnet from the metamorphosed altered rocks associated with the Dawson deposit are almandine-rich (shown as mole% end-member molecule) with lesser amounts of pyrope and minor amounts of the spessartine, grossular and andradite molecules (Alm74–82Prp11–17Sps2–8Grs2–3Adr<1, Fig. 11a, Table 4). These compositions are similar to those previously obtained by K. Wilson (unpub. report to U.S. Borax Company, 1982) from Dawson and by Sheridan and Raymond (Reference Sheridan and Raymond1984b) from the Sedalia deposit who reported garnet with a composition of 78 mole% almandine, 10 mole% pyrope, and smaller quantities of the spessartine and grossular molecules. Garnet from the Dawson deposit is more enriched in the almandine molecule and less enriched in pyrope than garnet in the various garnet-bearing rock types from Green Mountain (Alm67–75Prp16–24Sps3––5Grs3–4Adr<2). A single sample of garnet-bearing amphibolite from the latter deposit is more enriched in the grossular end-member than all the other garnets analysed here (Alm66Prp15Sps3Grs15Adr<2).

Figure 11. Ternary plots of (a) Garnet, (b) Zincian spinels and (c) Högbomite from the Dawson-Green Mountain trend. Garnet is plot in terms of spessartine, grossular + andradite and almandine + pyrope. The zincian spinels are plot terms of the gahnite-hercynite-spinel sensu stricto spinel end-members from the Dawson, Green Mountain and Horseshoe deposits. Numbers correspond to compositional ranges of gahnite from different geological settings as defined by Spry et al. (1986) and Heimann et al. (Reference Heimann, Spry and Teale2005): 1. marbles, 2. metamorphosed massive sulphide deposits and S-poor rocks in Mg-Ca-Al alteration zones, 3. metamorphosed massive sulphide deposits in Fe-Al metasedimentary and metavolcanic rocks, 4. metabauxites, 5.pegmatites, 6. unaltered and hydrothermally altered Fe-Al rich metasedimentary and metavolcanic rocks, and 7. Al-rich granulites. The högbomite compositions are shown for aluminous metasediments, ultramafic rocks, calcic skarns, Fe-Ti deposits and calc-silicate granulites reported by Spry & Petersen (Reference Spry and Petersen1989), quartzofeldspathic gneisses (Grew et al. Reference Grew, Hiroi and Shiraishi1990), metabauxites (Yalçin et al. Reference Yalçin, Schreyer and Medenbach1993; Feenstra Reference Feenstra1997; Ockenga et al. Reference Ockenga, Yalçin, Medenbach and Schreyer1998), cordierite gneisses (Heimann et al. Reference Heimann, Spry, Teale and Jacobson2006), pelites (Rakotonandrasana et al. Reference Rakotonandrasana, Arima, Miyawaki and Rambelson2010) dolomitic marble (Armbruster et al. 1998) and blackwall (Owens et al. Reference Owens, Belkin and Zerolis2013).
Table 4. Average major element compositions of garnet

TVD-3B garnet-biotite rock; TVD-6 quartz-garnet-biotite altered rock; TVD-7 ; TVD-40B garnet-gahnite-biotite-anthophyllite altered rock; TVD-50 laminated biotite-quartz rock; TVD19-79 garnet amphibolite; TVD19-81 garnet-quartz-biotite rock; TVD19-84 quartz-garnet-gahnite rock; TVD19-85 garnet-biotite-quartz rock; TVD19-87 garnet-biotite-quartz rock; TVD19-93 garnet-biotite-quartz rock.
5.a.4. Gahnite
Although some compositional data were obtained for gahnite from the Green Mountain deposit by Heimann et al. (Reference Heimann, Spry and Teale2005), no gahnite compositions have previously been determined from the Horseshoe, El Plomo and Dawson deposits. New compositions of gahnite in the metamorphosed altered rocks spatially associated with the DGMT deposits are discussed here. Table 5 shows the location, lithologies, mineralogy of the samples from which gahnite compositions were obtained, along with the average end-member spinel molecules (gahnite (Ghn), hercynite (Hc), spinel sensu stricto (Spl) and galaxite (Glx)). The compositions of representative gahnite compositions are given in Table 6, while all gahnite data obtained here are in Supplementary Appendix Table S1. Gahnite in sulphide-rich zones consist of approximately >10% visible sulphides (pyrite, pyrrhotite, chalcopyrite and sphalerite), whereas metamorphosed altered rocks in contact with sulphide zones generally contain <10% visible sulphides, with some containing no visible sulphides. In the sulphide zone at Dawson, drill hole DA18-16 (samples TVD-70 to TVD-76), sulphides are spatially associated with gahnite that predominantly occurs in contact with various silicates, including phlogopite, anthophyllite/gedrite, cordierite and muscovite in the absence of quartz. In these samples, gahnite compositions are generally similar (Ghn33–42Hc20–24Spl34–47, Fig. 11b). In sample TVD19-52 in drill hole GC2, a quartz-bearing sulphide specimen has the highest gahnite component of all samples analysed here (Ghn71Hc21Spl8Glx1). In contrast, gahnite in metamorphosed altered rocks from the Dawson deposit are considerably more variable and contain higher proportions of the gahnite and hercynite molecules and lower concentrations of the spinel end-member (Ghn32–55Hc23–40Spl14–27). Two non-sulphide-bearing altered rocks from the Sentinel prospect (immediately west of the main Dawson mineralized zone) contain gahnite with extremely variable compositions (Ghn4–68Hc21–77Spl10–20). Gahnite in a quartz-bearing sulphide-rich rock from the Green Mountain deposit has a similar composition (Ghn69Hc24Spl6Glx1) to that in drill hole GC2 from Dawson. Those in altered rocks that contain similar minerals to those associated with the Dawson deposit have the following compositions (Ghn0.4–55Hc27–67Spl16–43), while those in coarse quartz-garnet rocks show a narrower range of compositions and a generally lower amount of the spinel end-member (Ghn41–58Hc32–45Spl10–18).
Table 5. Gahnite-bearing rocks and spinel sensu stricto end members from the Dawson, El Plomo, Green Mountain, and Horseshoe deposits

Table 6. Representative average compositions of gahnite and högbomite

5.a.5. Högbomite
Högbomite, and its zincian counterpart zincohögbomite, are complex Fe-Mg-Zn-Al-Ti oxides that are composed of spinel and nolanite molecules (Armbruster, Reference Armbruster2002). They occur in various rock types metamorphosed to the upper amphibolite-granulite facies, including aluminous metasediments, ultramafic rocks Fe-Ti deposits, skarns, Fe ores and metamorphosed massive sulphide deposits (e.g. Teale, Reference Teale1980; Spry & Petersen, Reference Spry and Petersen1989; Feenstra Reference Feenstra1997; Rakotonandrasana et al. Reference Rakotonandrasana, Arima, Miyawaki and Rambelson2010; Owens et al. Reference Owens, Belkin and Zerolis2013). In Colorado, Gable and Sims (Reference Gable and Sims1969) reported högbomite in cordierite-bearing gneisses in the central Front Range, where it occurs along the contact between hercynite and magnetite or ilmenite. Ferrohögbomite (between 1.7 and 8.6 wt.% ZnO) was identified by Heimann et al. (Reference Heimann, Spry, Teale and Jacobson2006) from the Evergreen prospect, where it occurs in a corona of cordierite, hercynite, corundum, staurolite, and sillimanite along the contact between ilmenite and magnetite within gedrite-cordierite-garnet gneiss, while Heinrich and Griffitts (Reference Heinrich and Griffitts1948) noted its presence in the Turret mining districts in a corundum-chlorite-spinel rock. We identified högbomite from the Independence deposit in this district where it occurs in samples SAM09-51 and SAM09-53 (up to 0. 5 mm in length) in the metamorphosed alteration zone of the deposit with chlorite, gahnite, corundum, biotite, magnetite and sericitized cordierite. The latter sample also contains coarse garnet. The composition of two ferrohögbomite-bearing samples from the Dawson district (samples TVD-40B and TVD19-17) is given in Table 6 and shown in Fig. 11c. In sample TVD19, ferrohögbomite occurs in contact with magnetite and biotite in strongly altered cordierite that was replaced by sericite and late calcite. The samples contain up to 2.3 and 5.3 wt.% ZnO, respectively. For comparitive purposes, we include a previously unpublished composition of a zinc-bearing ferrohögbomite from the metamorphosed Rio Mazoe base metal district, Mozambique. It contains up to 10.0 wt% ZnO and occurs along the contact between magnetite and zincian spinel, the latter of which contains between 21 and 23 wt.% ZnO (Table 6).
5.a.6. Sulphides
In an attempt to apply the sphalerite geobarometer of Hutchison and Scott (Reference Hutchison and Scott1981) to determine the peak pressures of metamorphism that affected the deposits, sphalerite in contact with pyrite and pyrrhotite was evaluated. However, application of the geobarometer was unsuccessful because pyrrhotite is primarily monoclinic rather than the required hexagonal form. Nevertheless, sphalerite in contact with both pyrite and pyrrhotite or pyrrhotite alone contains between 6.2 and 10.0 wt.% Zn, with more than 95% of values between 6.5 and 8.0 wt.% of Zn regardless of the metamorphic grade of the deposit. Sphalerite coexisting with pyrite only contains between 2.7 and 5.7 wt% Zn, with one anomalous sample from Cotopaxi containing approximately 0.4 wt.% Zn. The Cd content of sphalerite using a count time of 60 seconds on the Cd peak is 1920 to 2680 ppm (Supplementary Appendix Table S2a), while that for the shorter 10 second count time shows a slightly broader range of 240 to 3240 ppm (Supplementary Appendix Table S2b). The Mn content reaches up to 0.34 wt.% Mn but is generally <0.2 wt.% Mn, while the Cu content is even lower (mostly <0.1 wt. Cu).
6. Geochemistry of various rocks in the Dawson-Green Mountain trend
6.a. Composition of granitoids in the Dawson area
Whole rock analyses of four types of granitoids (biotite-quartz monzodiorite/diorite, pink banded unit, leucogranite and alkali granite) adjacent to the Dawson deposit were done to evaluate chemical similarities and differences between the various rock types and to assist in determining their origin (Supplementary Appendix Tables S3a1, S3a2, S3a3, S3b, S3c, S3d1, S3d2). Although the Al2O3 concentrations overlap for the biotite-quartz monzodiorite/diorite (14.4 to 17.4 wt.% Al2O3) and the pink banded unit (11.4 to 15. 1 wt.% Al2O3), these contents are generally higher than those for the alkali granite (12.6 to 13.4 wt.% Al2O3) and leucogranite (9.2 to 14.1 wt.% Al2O3). The silica contents for the last three granitoids range from 68.8 to 80.7 wt.% SiO2, while those for biotite-quartz monzodiorite/monzonite range from 55.1 to 62.7 wt.% SiO2. These concentrations, when combined with values of total alkalis (Na2O+K2O = 3.6 to 6.6 wt.%), show that this granitoid falls in the monzodiorite and diorite fields, while the three other granitoids plot as granites (Fig. 12a). The monzodiorites/diorites have higher concentrations of Co, Cs, Ga, Nb, Ni, P2O5, Sc, TiO2, V, Zn and Zr than the three types of granites (Supplementary Appendix Tables S3a1, S3a2, S3a3). Where the composition of the granitoids are plotted as CaO+Na2O+K2O vs SiO2 the granites plot primarily in the calc-alkalic and calcic fields while most of the monzodiorites/diorites plot in the calc-alkalic field (Fig. 12b, f). These four granitoids can also be considered to be peraluminous ferroan granitoids as indicated in plots of FeOtotal/(FeOtotal+MgO) vs SiO2 wt.% (Fig. 12b) and Al2O3/(Na2O+K2O) vs Al2O3/(CaO+Na2O+K2O; Fig. 12c). Plots of Ta vs Y, and Rb vs Y+Nb support the concept that these granitoids formed in a volcanic arc setting (Fig. 12d–f). Such a setting was proposed previously for the Paleoproterozoic granites in Colorado (e.g. Wobus et al. Reference Wobus, Folley, Wearn and Noblett2001).

Figure 12. Discrimination diagrams of granitoids (biotite-quartz monzonite/diorite, alkali granite, pink banded unit and leucogranite) spatially related to the Dawson deposit. (a) Plot of Na2O+K2O vs SiO2 for intrusive rocks (after Le Bas et al. Reference Le Bas, Le Maitre, Steckeisen and Zanettin1986). (b) FeOtotal/(FeOtotal+MgO) vs SiO2 weight % (after Frost & Frost (Reference Frost and Frost2011). (c) A/NK [Al2O3/(Na2O+K2O)]molar vs A/CNK [Al2O3/(CaO+Na2O+K2O)]molar (after Maniar & Piccoli, Reference Maniar and Piccoli1989). (d) Ta vs Yb discrimination plot for syn-collision (syn-COLG), volcanic arc (VAG), within plate (WPG) and normal and anomalous ocean ridge (ORG) granites (after Pearce et al. Reference Pearce, Harris and Tindle1984). (e) Rb vs Y+Nb for the same granitoids in 10d. (f) Na2O+K2O-CaO (so-called MALI index of Frost & Frost, Reference Frost and Frost2008).
Chondrite-normalized REE plots of the pink banded unit (Supplementary Appendix Fig. S1a) and monzodiorites/diorites (Supplementary Appendix Fig. S1b) show light rare earth element (LREE) enrichment, heavy rare earth element (HREE) depletion and negative Eu anomalies. The overall REE content is generally higher for monzodiorites/diorites compared to pink banded unit granitoids with the latter having flatter HREE signatures. Although leucogranites (Supplementary Appendix Fig. S1c) and alkali granites show LREE enrichment (Supplementary Appendix Fig. S1d), the HREE patterns are more complicated for these two types of granitoids as alkali granite samples TVD19-6 and TVD19-7 show arcuate HREE patterns (with negative Eu anomalies), while sample TVD19-3 shows a small positive Eu anomaly. All but one (TVD19-8) of the five leucogranite samples show positive Eu anomalies whereas samples WP27WR, WP28WR and WP30WR exhibit an increase in chondrite-normalized HREE patterns from Gd to Lu (Supplementary Appendix Fig. S1d).
6.b. Geochemistry of other least altered rocks in the Dawson-Green Mountain trend
In addition to granitoids spatially related to the Dawson deposit, various gneisses (quartz-K-feldspar-biotite gneiss from Cotopaxi, paragneiss from Dawson, Green Mountain and Horseshoe, orthogneiss from Green Mountain), quartz garnetite from Green Mountain, and amphibolite from Green Mountain and Dawson were also analysed (Supplementary Appendix Tables S3b, S3c, S3d1, S3d2).
The most common lithology adjacent to the Cotopaxi deposit is a rock that Salotti (Reference Salotti1965) referred to as ‘biotite gneiss’ largely because biotite is the most common mafic mineral. However, the rock essentially consists of quartz, microcline and oligoclase with minor biotite, muscovite, garnet, titanite, gahnite, magnetite and zircon. The magnetite occurs as isolated subhedral to euhedral grains surrounded by a circular moat of feldspar. Whole rock composition of this rock shows that it contains 77 to 84 wt. SiO2, 8 to 11 wt% Al2O3 and 4 to 5 wt.% K2O, with low amounts of Na2O (0.3 to 1.7 wt%) and low total Fe2O3+MgO (2.12 to 3.73 wt%) (Supplementary Appendix Table 3b). The analysed rocks contain up to 469 ppm Cu, 46 ppm Pb and 1292 ppm Zn, the last of which reflects the presence of gahnite, and between 2131 and 4547 ppm Ba. Although it is likely this rock represents a metapelite, the other possibility is that the protolith consisted of volcaniclastics largely derived from a rhyolitic source rock (e.g. rhyolitic tuff). These two alternatives are hard to distinguish given the high metamorphic grade and the similarity of chondrite-normalized REE patterns (light HREE-enriched, HREE depletion and a negative Eu anomaly, Supplementary Appendix Fig. S2a) which are characteristic of both metamorphosed pelitic rocks (e.g. Slack & Stevens, Reference Slack and Stevens1994) and rhyolites (e.g. Streck, Reference Streck2014).
The precursors to most of the gneisses from the Dawson and Green Mountain deposits is unclear due to the effects of metamorphism and the lack of volcanic features. Whether they are metasedimentary rocks or have an igneous origin is debatable. They vary from cordierite-plagioclase-quartz-biotite gneiss (TVD19-58), to more quartz-rich varieties that contain mostly garnet, biotite and sillimanite (TVD19-15, TVD19-81, AHCO-16). These rocks also contain trace magnetite-pyrite±pyrrhotite. The cordierite-rich gneiss (>70 cordierite) is silica-poor (52 wt.% SiO2) and the most enriched in Fe2O3, MgO, Na2O, K2O, MnO, TiO2, V and Rb, relative to the other three samples which contain between 71 and 87 wt.% SiO2 (Supplementary Appendix Table S3b, Supplementary Appendix Fig. S2b). These four rocks contain between 106 and 845 ppm Ba, which is considerably less Ba than in the biotite gneiss from Cotopaxi. These rocks also contain 14 to 64 ppm Cu, 6 to 14 ppm Pb and 30 to 336 ppm Zn. Like the biotite gneiss from Cotopaxi, chondrite-normalized REE patterns for samples of gneisses from Dawson and Green Mountain are light HREE-enriched and HREE-depleted and show a negative Eu anomaly (Supplementary Appendix Fig. S2b).
A rock that is locally found in the Green Mountain and Dawson areas is a quartzo-feldspathic garnet-rich gneiss (Fig. 4i) that superficially resembles the so-called Potosi Gneiss intimately associated with the Broken Hill deposit, Australia, which Stevens & Barrons (Reference Stevens and Barrons2002) considered to be a metamorphosed rhyodacite. Two samples from Green Mountain contain 72 and 75 wt.% SiO2, 11 and 13 wt.% Al2O3, 9 wt.% Fe2O3, <2 wt.% K2O + Na2O and 2 and 3 wt.% MgO (Supplementary Appendix Table S3b). Both samples are also generally depleted in Cu (14 and 34 ppm), Pb (5 and 6 ppm) and Zn (39 and 80 ppm) and show LREE-enriched, HREE-depleted and prominent negative Eu anomalies chondrite-normalized REE patterns (Supplementary Appendix Fig. S2c).
Quartz-garnet rocks spatially associated with the Green Mountain deposit resemble exhalative quartz garnetites (meta-exhalites) spatially associated with metamorphosed massive sulphide deposits elsewhere in the world (e.g. Spry et al. Reference Spry, Peters and Slack2000, Reference Spry, Heimann, Messerly and Houk2007). The rocks at Green Mountain generally consist of garnet and quartz with minor to moderate amounts of biotite in some samples (Fig. 7a). The quartz to garnet ratio is generally high with garnet reaching up to 1 cm in diameter. These rocks contain 74 to 86 wt.% SiO2, 5 to 10 wt.% Al2O3, 7 to 10 wt.% Fe2O3 generally <1 wt.% K2O + Na2O (0.3 to 1.7 wt.%) and 1 to 2 wt.% MgO (Supplementary Appendix Table S3c). They also contain up to 339 ppm Cu and 96 ppm Zn but are depleted in Pb (<4 ppm). Chondrite-normalized REE patterns for the quartz garnetites from Green Mountain are light HREE-depleted and HREE-enriched and show a negative Eu anomaly (Supplementary Appendix Fig. S2d).
At Green Mountain, garnet amphibolite is deformed and consists of coarse garnet (up to ∼1 cm) in a matrix of plagioclase, hornblende, quartz and minor magnetite (Fig. 4h). However, there are also garnet-free amphibolites at Green Mountain and the Dawson deposit. For example, sample EHB-20-AMPH from Green Mountain contains the same minerals as the garnet amphibolite (minus garnet), but it also contains disseminated chalcopyrite, pyrite, and sphalerite within hornblende and along grain boundaries, which are the dominant metallic minerals in the deposit. These amphibolites contain 43 to 54 wt.% SiO2, 11 to 16 wt.% Al2O3, 3 to 18 wt.% Fe2O3, 0.7 to 3.0 wt.% K2O + Na2O and 3 to 12 wt.% MgO (Supplementary Appendix Table S3c). Of note is that amphibolite from Green Mountain contains considerably higher concentrations of Fe2O3 (16 to 18 wt.%) than those from the Dawson deposit, which contain between 4 and 5 wt.% Fe2O3.
6.c. Geochemistry of metamorphosed altered rocks associated with mineralization
Samples of metamorphosed altered rocks, mainly gahnite-bearing, were collected from the El Plomo, Horseshoe, Cinderella, Cotopaxi and Green Mountain deposits to complement previous studies by Spry et al. (Reference Spry, McFadden, Teale, Alers, Shallow and Glenn2022b) on nodular sillimanite rocks, which they considered to represent zones of metamorphosed hydrothermal alteration. In general, these gahnite-bearing altered rocks are dominated by orthoamphiboles and phlogopite, but they also contain various combinations of the following minerals: clinoamphibole, cordierite, quartz, chlorite, disseminated sulphides, chlorite, zircon, epidote and allanite. Chondrite-normalized REE plots of these rocks mostly show an enrichment in LREEs and flat HREEs with negative Eu anomalies (Supplementary Appendix Fig. S2f). The REE patterns resemble those for metasedimentary rocks (Supplementary Appendix Fig. S2b), quartzofeldspathic garnet-rich gneisses (Supplementary Appendix Fig. S2c) and amphibolites (Supplementary Appendix Fig. S2e). Sample TVD19-66 shows the lowest total amount of REEs and exhibits a weak positive Eu anomaly. It also contains the highest Ca content (23.6 wt.% CaO) and among the lowest concentrations of Fe (3.6 wt.% Fe2O3) of the metamorphosed altered rocks (Supplementary Appendix Table 3d2).
7. Sulphur isotope studies
7.a. Sampling methods and results
Samples were collected from drill core (Dawson, El Plomo), surface dumps (El Plomo, Horseshoe, Vulcan, Good Hope) and archive collections from the US Geological Survey (Copper King, Denver City), and museum collections of the Denver Museum of Nature and Science (Betty, Bon Ton, Sedalia, Cotopaxi). The sulphur isotope data are listed in Table 7 and shown in Fig. 13. Values of δ34S (n = 86) of sulphides in different massive sulphide deposits in central-southern Colorado are generally similar and centred around 0–+2‰ with a range of δ34S = −3.29 to +6.45‰. Isotopic values for the individual minerals are δ34S = −0.90 to +6.45‰ for pyrite (n = 56), +2.51 to +3.83‰ for pyrrhotite (n = 4), −1.97 to +3.40‰ for chalcopyrite (n = 5), −3.29 to +5.85‰ for sphalerite (n = 18) and −0.90 to +1.16 for galena (n = 3). We have divided the isotopes into three geographic regions: western (Good Hope, Vulcan and Denver City), central (Betty, Bon Ton, Sedalia, Copper King and Cotopaxi) and eastern deposits (Dawson, El Plomo and Horseshoe). Values of δ34S of −0.24 to +0.48‰ for pyrite from Good Hope (n = 5) are within the range of isotope values of −0.90 to +2.07‰ (n = 20) for pyrite from the Vulcan deposit. Single isotopic values for sphalerite and galena from the Vulcan deposit are −0.60‰ and +1.62‰, respectively, while sphalerite from the Denver City deposit ranges from −0.69 to +0.26‰ (n = 3). The eastern deposits generally exhibit higher δ34S values than the western deposits: Dawson (δ34S = +1.27 to +3.69‰ for pyrite, n = 6; δ34S = +3.27 to +3.47‰ for pyrrhotite, n = 2); El Plomo (δ34S = +1.12 to +2.42‰ for pyrite, n = 5; δ34S = +1.52 to +1.76‰ for sphalerite, n = 3; δ34S = −0.40‰ for galena; n = 1); and Horseshoe (δ34S = +0.77 to +3.23‰ for pyrite, n = 18; δ34S = +2.92 to +3.40‰ for chalcopyrite, n = 2; δ34S = +2.93‰ for galena; n = 1). Sulphides from Cotopaxi mostly plot in the negative range (δ34S = −3.29 to −1.23‰, n = 9), with the one pyrite measurement plotting at a much higher, anomalous, positive value (δ34S = +6.45‰). In this context, it should be noted that G.T. Ririe (unpub. PhD thesis, Univ. Iowa, 1981) obtained five isotopic compositions of sulphides from Paleoproterozoic massive sulphide deposits in central Colorado with the lightest values also being from the Cotopaxi deposit (δ34S = −4.4‰ and −2.9‰ for galena and pyrite, respectively). The other three values obtained by G.T. Ririe (unpub. PhD thesis, Univ Iowa, 1981) are δ34S = +0.1‰ for chalcopyrite from Green Mountain, −0.4‰ for pyrrhotite from an unidentified ‘Grape Creek area mine’, and +1.1‰ for pyrite from the Marion deposit in the southern Wet Mountains. The isotopic values obtained by him overlap those obtained in the present study.
Table 7. Sulphur isotope compositions of sulphides from Proterozoic massive sulphide deposits, Colorado

Ccp, chalcopyrite; Gn, galena; Po, pyrrhotite; Py, pyrite; Sp, sphalerite.

Figure 13. Sulphur isotopic compositions of sulphides (chalcopyrite, galena, pyrite, pyrrhotite and sphalerite) from metamorphosed massive sulphide deposits, Colorado.
8. Discussion
8.a. Comparison of metamorphosed massive sulphide deposits in Colorado
To see through the camouflaging effects of metamorphism and deformation, deposits in the Gunnison area are the best to evaluate the genesis of ore deposits as they were least deformed and subject to the lowest metamorphic grade. There are at least eight deposits in the Gunnison area (White Iron, Headlight, Anaconda, Ironcap, Good Hope-Vulcan, Midland, Denver City and Yukon). The Gunnison deposits occur in an east-west trending belt over a distance of ∼50 km, with all but the Yukon deposit occurring in the Dubois Greenstone Belt. As pointed by Drobeck (Reference Drobeck, Epis and Callender1981) and Sheridan et al. (Reference Sheridan, Raymond, Cox, Epis and Callender1981), the deposits are hosted in metamorphosed bimodal felsic and mafic igneous rocks along with metamorphosed epiclastic and pyroclastic rocks and spatially related iron formation (e.g. Vulcan-Good Hope mine). Drobeck (Reference Drobeck, Epis and Callender1981) suggests the protoliths of the metamorphosed felsic rocks include rhyolite, quartz latite and dacite, while precursors to the mafic rocks were likely basalts and andesites (now hornblende schists and amphibolite). Although there are granitoids throughout the Dubois Greenstone Belt, they do not host sulphide mineralization. Instead, the deposits are elongate and commonly occur along the contacts between mafic and felsic igneous rocks.
In the Sedalia mine area, which was metamorphosed to lower amphibolite facies, bimodal mafic and felsic metavolcanic rocks (U-Pb age of 1728 ±5 Ma, Boardman & Bickford, Reference Boardman and Bickford1982) were identified by Boardman (Reference Boardman1986) who described quartz-feldspar volcaniclastics, basaltic volcanics (basalt porphyry) and volcaniclastics, and slightly younger gabbro/diabase intrusive sheets. Phenocrysts and flattened pumice fragments occur in some rocks. A granite pluton (1671 ±5 Ma, J. Hankins, unpub. BS thesis Carleton College, 1981) located ∼ 7 km north of the Salida deposit is younger than the volcaniclastic rocks. The dominant rock type associated with the Sedalia deposit is feldspathic gneiss, which Sheridan and Raymond (Reference Sheridan and Raymond1984b) suggested was originally flows or tuffs with the composition of rhyodacite. Garnet-cordierite-anthophyllite rocks are the most common host to mineralization. Of note is also the presence of porphyroblastic andalusite biotite-quartz gneiss/schist, in which the porphyroblasts of andalusite are up to 4 cm in length. This rock type may be the lower-metamorphic grade equivalent of nodular sillimanite rocks that Spry et al. (Reference Spry, McFadden, Teale, Alers, Shallow and Glenn2022b) considered to be metamorphosed zones of hydrothermal alteration. Similar andalusite-rich rocks occur in the footwall alteration of the Kanmantoo Cu deposit, South Australia (Pollock et al. Reference Pollock, Spry, Tott, Koenig, Both and Ogierman2018).
Despite the difficulties in separating primary geological features from features that are associated with the effects of metamorphism, the studies of, for example, Drobeck (Reference Drobeck, Epis and Callender1981), Sheridan and Raymond (Reference Sheridan and Raymond1984a, Reference Sheridan and Raymond1984b) and Boardman (Reference Boardman1986) show the presence of bimodal volcanic rocks spatially associated with mineralization in the lower grade metamorphic rocks. At higher metamorphic grades (upper amphibolite facies), the presence of primary volcanic textures is difficult or almost impossible to discern given the strong deformation that affected these rocks and the spatially related ore deposits. Despite the high proportion of granitoids in the vicinity of the Cotopaxi deposits and along the DGMT, these deposits occur in hydrothermally altered metasedimentary rocks (both clastic and likely volcaniclastic) and not in granitoids. Moreover, the genetic relationship to granitoids is speculative at best given there are no geochronological studies done on them in the DGMT. On the other hand, there is geochemical and textural evidence for the presence of amphibolite and gabbros in the sequence of rocks below the Dawson deposit, which was metamorphosed to the upper amphibolite facies, along with likely rhyodacites (i.e. precursors to the quartzo-feldspathic garnet-rich gneiss). Such rocks are also found adjacent to the Green Mountain deposit along with garnet amphibolite. The presence of visible sphalerite, chalcopyrite and pyrrhotite in metagabbro near Green Mountain raises the question as to whether sulphides were synchronous with the formation of the gabbro or whether the sulphides replaced it after the gabbro formed. Regardless, there is an intimate relationship among volcanic rocks, including volcaniclastic rocks, metamorphosed clastic rocks and sulphide mineralization in all the deposits studied here.
8.b. Genesis of metamorphosed massive sulphide deposits in central Colorado compared to other massive sulphide deposits
The origin of massive sulphide deposits in Colorado is controversial with four genetic models having been proposed VMS (e.g. Drobeck, Reference Drobeck, Epis and Callender1981; Sheridan & Raymond, Reference Sheridan and Raymond1984a), skarn (e.g. Salotti, Reference Salotti1965, Heinrich Reference Heinrich1981), BHT (https://www.zephyrminerals.com/el-plomo-section) and peraluminous granitoids-hosted deposits (Kleinhans & Swan, Reference Kleinhans and Swan2022). In this evaluation, we address aspects that relate to the geological, mineralogical, and geochemical features identified here and data reported in previously published studies. These features are summarized in Table 8 and discussed below.
Table 8. Comparison of Paleoproterozoic depoits in Colorado with VMS, BHT, Skarn, and PISZHG deposits*

* VMS, volcanogenic massive sulphide, BHT, Broken Hill type; PISZG, peraluminous-intrusive shear zone-hosted gold.
† Iron formation, quartz garnetite, and quartz-gahnite rocks.
√ = yes; X = no; √X = most commonly yes but no at some deposits; X√ = most commonly no but yes in some deposits.
8.b.1 Skarn model
Heinrich (Reference Heinrich1981) recognized three types of sulphide deposits in metamorphic rocks in central Colorado, W, Cu-W, and Cu-Zn, and proposed that all three types are skarn deposits. The W and Cu-W deposits are primarily associated with meta-carbonate horizons with various combinations of the following minerals: vesuvianite, grossular, diopside, epidote, scapolite, tremolite, calcite, titanite, zoisite and clinozoistite (among others) forming the skarn assemblage. However, the Cu-Zn deposits studied by Heinrich (Reference Heinrich1981) and here contain sphalerite and/or gahnite, and nearly all deposits are not hosted in metamorphosed calcite/dolomite horizons or calc-silicate gneisses, exceptions being three small Cu-Zn±Pb occurrences (Cresswell, Hosa Lodge, F.M.D.) in Jefferson County that occur in calc-silicate-quartz-microcline biotite gneiss (Eckel, Reference Eckel1997). Gahnite at the Creswell mine occurs sillimanite-rutile-topaz-rich gneiss, which is a likely exhalative unit and not a skarn (Marsh & Sheridan, Reference Marsh and Sheridan1976; Heimann et al. Reference Heimann, Spry, Teale and Jacobson2006). Furthermore, Salotti (Reference Salotti1965) in identifying the Cotopaxi deposit as a skarn deposit noted that the sulphide mineralization is hosted in amphibolite. However, the amphibolite Salotti (Reference Salotti1965) described consists of anthophyllite-cordierite-biotite-gahnite±clinohumite±garnet±diopiside alteration. Other minor hornblende-plagioclase amphibolites occur in the vicinity of the deposit but do not host ore. Salotti (Reference Salotti1965) also pointed out that the granite spatially associated with the Cotopaxi deposit is post-mineralization and is a ‘typical Pikes Peak granite’ which formed around 1.1 Ga. There is no granite genetically related to the mineralization, and there are no typical endoskarns or exoskarns spatially related to the massive sulphides.
8.b.2. Granite-hosted model
Kleinhans and Swan (Reference Kleinhans and Swan2022) recently proposed that the Cu-Zn±Pb deposits are high-temperature hydrothermalite fractionates of granitoid intrusive rocks and further argued that such a model was also responsible for the formation of the Broken Hill Pb-Zn-Ag deposit, Australia. Geochemical studies here support the idea of Kleinhans and Swan (Reference Kleinhans and Swan2022) that peraluminous granites are spatially associated with the Dawson deposit, but they state that the sulphide mineralization is hosted by a biotite aplite. This terminology has genetic implications, since an aplite is a fine-grained igneous rock primarily composed of quartz, alkali feldspar, and plagioclase with small amounts of muscovite and biotite. Instead, the so-called aplite they identified is a sheared anthophyllite-biotite-garnet-cordierite-feldspar gahnite rock. Such a rock resembles metamorphosed altered rocks spatially associated with all the massive sulphide deposits studied here that were metamorphosed to the upper amphibolite facies. Moreover, Cu-Zn deposits in Colorado occur in metasedimentary and metavolcanic rocks and not in granitoids. Accordingly, the granite-related hydrothermalite model of Kleinhans and Swan (Reference Kleinhans and Swan2022) is tenuous at best, especially when the ages of the granitoids spatially related to the Dawson deposit, which was the focus of their study, are unknown. Furthermore, the granitoids spatially related to the Sedalia and the Cotopaxi deposit are younger than the sulphide mineralization, which further supports the concept that sulphide mineralization is genetically unrelated to the formation of granitoids (Salotti, Reference Salotti1965; Sheridan & Raymond, Reference Sheridan and Raymond1984b).
In supporting their genetic model, Kleinhans and Swan (Reference Kleinhans and Swan2022) suggested that the giant BHT Pb-Zn-Ag deposit was an example of a high-temperature hydrothermalite fractionate that formed from granitoids. Peraluminous granites occur in the Willyama Supergroup that hosts the Broken Hill Pb-Zn-Ag deposit with the peraluminous Rasp Ridge Granite Gneiss having the same age as the mineralization. However, this metamorphosed granitoid, which is a quartz-K feldspar-plagioclase-biotite gneiss, occurs stratigraphically below the deposit and contains no sulphide mineralization (Page et al. Reference Page, Stevens and Gibson2005). Instead, sulphide mineralization occurs in metasedimentary rocks associated with the Hores Gneiss, a regionally extensive, metamorphosed volcaniclastic unit that is stratigraphically equivalent to the orebody. The immediate host to ore is the Potosi Gneiss, a garnet-biotite-rich quartzofeldspathic gneiss that has a rhyodacite composition, which contains volcanic quartz and feldspar phenocrysts, and rip-up clasts of sediment (Stevens & Barrons, Reference Stevens and Barrons2002). Therefore, there is no evidence that the Broken Hill deposit formed from magmato-hydrothermal, high-temperature hydrothermalites. Their proposal also does not recognize the fact that there are hundreds of small BHT Pb-Zn deposits in the Willyama Supergroup that show no spatial relationship to granitoids.
8.b.3. Broken Hill-type (BHT) model
BHT deposits are among the largest metamorphosed massive sulphide deposits in the world with Broken Hill, Australia, being the biggest and Cannington being one of the highest grade silver deposits (38 Mt @ 500g/t Ag, 11.1% Pb and 4.4% Zn). Spry and Teale (Reference Spry and Teale2021) suggested the major characteristics of BHT deposit are: 1. high Pb+Zn+Ag values, 2. metamorphosed from greenschist to granulite facies, 3. sulphur-poor mineralogy (e.g. gahnite, plumbian feldspar, löllingite, pyrrhotite rather than pyrite); 4. a spatial association of sulphides to bimodal (felsic and mafic) volcanic rocks, oxidized metasedimentary rocks, and stratabound gahnite- and garnet-bearing rocks and iron formations; 5. stacked orebodies with characteristic Pb:Zn:Ag ratios and skarn-like Fe-Mn-Ca-F gangue assemblages, and the presence of minor Cu, Au, Bi, As, and Sb; 6. restriction of deposits to rocks of 1.70 to 1.67 Ga age within the Diamantina Orogen; 7. spatial association to continental rifts; and 8. no genetic relationship to granitoids. Of the metamorphosed massive sulphide deposits in Colorado only the Green Mountain and El Plomo deposits share some characteristics with BHT deposits, but they also show significant differences. Like BHT deposits, Green Mountain is spatially associated with iron formation, gahnite-rich rocks, quartz garnetite, garnet amphibolite and a garnet-biotite-rich quartzofeldspathic gneiss, the last of which shows mineralogical and geochemical characteristics that resemble the Potosi Gneiss at Broken Hill. REE patterns for the quartz garnetite at Green Mountain contrasts to quartz garnetites from elsewhere in the world including Broken Hill, which are light HREE-enriched and HREE-depleted (e.g. Spry et al. Reference Spry, Peters and Slack2000). Instead, the patterns of quartz garnet rocks from Green Mountain (see Supplementary Appendix Fig. S2d) more closely resemble the chondrite-normalized patterns of individual garnet analyses reported in the literature (e.g. Lottermoser, Reference Lottermoser1988; Schwandt et al. Reference Schwandt, Papike, Shearer and Brearley1993; Spry et al. Reference Spry, Peters and Slack2000). This is likely due to garnet essentially contributing nearly all of the REEs to the whole rock composition with quartz and other minor to trace minerals providing only a small contribution.
Garnet amphibolite from Green Mountain was analysed because it mineralogically and texturally resembles garnet amphibolite spatially associated with the Broken Hill deposit, Australia (e.g. Raveggi et al. Reference Raveggi, Giles, Foden and Raetz2007) and the King Zn VMS deposit, Western Australia (Hollis et al. Reference Hollis, Podmore, James, Doran, Menuge, Yeats and Wyche2019). The high Fe2O3 and TiO2 concentrations suggest that these rocks are ferrobasalts that were recognized previously by Byerly et al. (Reference Byerly, Melson and Vogt1976) from the Galapagos spreading centre. They are rare in nature but characteristic of the so-called high Fe-Ti basaltic rocks (now garnet amphibolite) associated with the Broken Hill deposit. The REE patterns of garnet-amphibolite from Green Mountain and Dawson show slightly enriched HREEs and slightly depleted LREEs with moderate to prominent negative Eu anomalies, which are similar to those from Broken Hill (Supplementary Appendix Fig. S2e).
However, important differences show that Green Mountain is a Cu-Zn deposit with only minor Pb and Ag values, and it does not show stacked orebodies or skarn-like Fe-Mn-Ca-F gangue assemblages. Instead, sulphide mineralization at Green Mountain is hosted in anthophyllite-cordierite-gahnite-almandine garnet altered rocks. Like El Plomo and other massive sulphide deposits in Colorado, Green Mountain formed in a volcanic arc setting rather than being associated with continental rifting. Although there is uncertainty with the age of sulphide mineralization in Colorado, Pb-Pb isotope ages (Sheridan & Raymond Reference Sheridan and Raymond1984a, Reference Sheridan and Raymond1984b) suggest that sulphide mineralization is older (1.8−1.7 Ga) than BHT deposits in the Diamantina Orogen (Stevens et al. Reference Stevens, Page and Crooks2008). In evaluating the prospectivity of the DGMT for BHT deposits, Zephyr Minerals (https://www.zephyrminerals.com/el-plomo-section) proposed that there was base metal zoning along the DGMT with El Plomo prospect showing high grades of Pb, Zn, and Ag in drill holes GC-8 and GC-9. For example, drill hole GC-9 contains up to 10.2% Zn, 0.03% Pb, and 5.9 g/t Ag, over 2.5 m and 0.02% Zn, 2.61% Pb, and 179 g/t Ag, over 0.7 m (unpub. Report to US Borax, 1981). While the Ag and Zn grades rival those of Broken Hill, they are over intersections of <3 m with the high Ag grades correlating with the higher grades of Pb. The deposits are dominated by pyrite and pyrrhotite rather than sphalerite and galena. Furthermore, El Plomo shows no stacked orebodies and is not spatially associated with Fe-Mn-Ca-F gangue. Instead sulphide mineralization primarily occurs in in anthophyllite-cordierite-gahnite-almandine garnet altered rocks similar to those associated with other massive sulphide deposits metamorphosed to the upper amphibolite facies elsewhere (e.g. Montauban, Quebec, Bernier et al. Reference Bernier, Pouliot and MacLean1987; Geco, Ontario, Zaleski et al. Reference Zaleski, Froese and Gordon1991; King, Western Australia, Hollis et al. Reference Hollis, Podmore, James, Doran, Menuge, Yeats and Wyche2019). While some deposits along the DGMT show some geological characteristics similar to BHT deposits, including sulphur isotope compositions of sulphides centred on 0 per mil, nearly all other deposits in Colorado including Dawson and Horseshoe along the DGMT show little resemblance to BHT deposits (Spry et al. 2021).
8.b.4. Volcanogenic massive sulphide model
The geochemical, mineralogical and geochemical features for deposits in Colorado given in Table 8 are most supportive of a VMS model. Such features include: 1. the spatial association of sulphides with bimodal felsic and mafic igneous rocks within packages of metasedimentary/metavolcaniclastic rocks. Although best observed in the least metamorphosed massive sulphide districts Gunnison (upper greenschist–lower amphibolite facies) and in the vicinity of the Sedalia district (lower amphibolite facies), such rocks also host mineralization metamorphosed to the upper amphibolite facies, even where the local terrane (e.g. Dawson) is dominated by granitoids. For example, meta-amphibolites and metagabbros are commonly associated with mineralization along with the presence of garnet-biotite-rich quartzofeldspathic gneiss that are likely metarhyodacites. 2. Sulphide mineralization is commonly associated with nodular sillimanite rocks (andalusite-rich rocks at the Sedalia deposit) or anthophyllite/gedrite-cordierite-biotite-garnet-gahnite rocks both of which are zones of metamorphosed hydrothermal alteration (e.g. Spry et al. Reference Spry, McFadden, Teale, Alers, Shallow and Glenn2022b). Deposits in the Gunnison area are spatially associated with quartz-muscovite±carbonate (e.g. Denver City) or chlorite-muscovite alteration (e.g. Ironcap, Vulcan). Orthoamphibole-cordierite rocks generally result from a reaction between chlorite and muscovite which is a common assemblage in the alteration zones of deposits in the lower grade Gunnison district. Note there is no evidence of footwall stockwork alteration zones below deposits, instead the alteration appears to be more stratabound similar to that associated with the Scuddles VMS deposit, Australia (Large, Reference Large1992). 3. A spatial association of some deposits to meta-exhalite/meta-inhalites of Spry et al. (Reference Spry, Peters and Slack2000). Such rocks include iron formation (e.g. Vulcan-Good Hope, Green Mountain, Dawson), gahnite-bearing schists (e.g. Sedalia), quartz-garnetite (Green Mountain) and sulphide-bearing metachert (e.g. Gunnison district). 4. Stratiform nature of massive sulphide horizons (e.g. Dawson). 5. At Dawson, sulphide mineralization is hosted in metasedimentary/metavolcaniclastic rocks, which occur as inclusions in granitoids. This implies these rocks and contained mineralization are older than the granitoids.
The presence of elevated concentrations of accessory elements such as Au, Ag, As, Bi, Hg, Sb, Se, and Te at Vulcan-Good Hope, Au, Ag, Bi, As, and Sb at Green Mountain, and Au, Bi, Se, and Te at Dawson are not unusual for VMS deposits (e.g. Barrie & Hannington, Reference Barrie and Hannington1999). In the Good-Hope and Dawson systems, minerals containing these elements cross-cut earlier formed massive sulphides, while Au, Ag, Bi, As and Sb are elevated in the massive sulphide horizon at Green Mountain. Although Drobeck (Reference Drobeck, Epis and Callender1981) raised the possibility that veins containing minerals in the system Au-Ag-Te-Se at Vulcan-Good Hope may be Miocene in age (although the age of the mineralization is unknown), Kleinhans and Swan (Reference Kleinhans and Swan2022) show that the Au-Te-Se-Bi assemblage at Dawson is associated with pink peraluminous granite, implying that these elements are Protererozoic in age, similar to that of the base metal mineralization. Given that Au, Ag, Bi, As and Sb are elevated in the highly deformed massive sulphides at Green Mountain begs the question as to whether these trace elements, along with the presence of minerals in the system Bi-Se-Te-S at Dawson, were the products of remobilization of the massive sulphides rather than being derived from the peraluminous granite. It should be noted that while this zone of bonanza gold in this assemblage parallels the massive sulphide at Dawson, it also occurs in contact with it locally. Evidence for the remobilization of gold in metamorphosed VMS deposits was reported previously from the Mobrun Cu-Zn (Larocque et al. Reference Larocque, Hodgson, Carbri and Jackman1995), Boliden Au-Cu-As (Wagner et al. Reference Wagner, Klemd, Wenzel and Mattsson2007) and Falun Zn-Pb-Cu-(Au-Ag) deposits (Kampmann et al. Reference Kampmann, Jansson, Stephens, Olin, Gilbert and Wanheinan2018). Of particular relevance is that the Falun deposit is spatially associated with various cordierite-anthophyllite altered rocks and that remobilization of the massive sulphides generated quartz veins containing elevated concentrations of Pb, Bi, Se and Au and the presence of Pb-Bi sulphosalts and native gold. These elements were likely liberated from pyrite (Kampmann et al. Reference Kampmann, Jansson, Stephens, Olin, Gilbert and Wanheinan2018). The association of cordierite-anthophyllite rocks to sulphide mineralization at Dawson and Green Mountain, the dominance of pyrite as the sulphide in both deposits and the presence of a trace element signature similar to that observed at Falun suggest that pyrite was also the likely host to these elements in the Colorado deposits, Similarly, such a liberation of trace elements from pyrite to generate elevated Au, Ag, As, Bi, Hg, Sb, Se and Te contents in cross-cutting quartz veins in Vulcan-Good Hope deposit is also likely.
One aspect of the deposits in the Grape Creek-Dawson trend is the spatial association with a deformation zone (the so-called Dawson shear zone of Kleinhans & Swan, Reference Kleinhans and Swan2022), which runs through or parallel to several deposits. The brecciated nature of the ore at Dawson, for example, implies that the ore was present prior to shearing. The question remains whether the location of the deformation zone which primarily occurs in the metasedimentary/metavolcaniclastic rocks is due to a competency contrast between the more easily deformed metasedimentary/metavolcaniclastic rocks and the surrounding granitoids, which dominate the lithologies between El Plomo and Dawson. The more than 30 minor prospects along the linear trend may simply be reflecting multiple hydrothermal vent sites as opposed to being derived from magmatic-hydrothermal fluids in a later formed shear zone.
The alteration box plot of Large et al. (Reference Large, Gemmell, Paulick and Huston2001) has been applied here to samples from the deposits with the aim of seeing through the camouflaging effects of metamorphism to identify the nature and intensity of the precursor alteration (Fig. 14). The box plot has been used previously to evaluate hydrothermal alteration associated with metamorphosed and relatively unmetamorphosed massive sulphide deposits (e.g. Theart et al. Reference Theart, Ghavami-Riabi, Mouri and Gräser2010, Frank et al. Reference Frank, Spry, Raat, Allen, Jansson and Ripa2019, Hollis et al. Reference Hollis, Podmore, James, Doran, Menuge, Yeats and Wyche2019). The chlorite-carbonate-pyrite index (CCPI = 100(MgO+FeOtotal)/(MgO+FeOtotal+Na2O+K2O) of Large et al. (Reference Large, Gemmell, Paulick and Huston2001) is plotted against the Ishikawa alteration index (AI = [100(MgO+K2O)/(MgO+K2O+Na2O+CaO)] with the least altered volcanic rocks plotting towards the centre of the diagram. Assuming a spatial relationship of mineralization to volcanic rocks, which was noted previously, samples from Green Mountain and Cotopaxi suggest the precursor alteration minerals were chlorite, sericite and pyrite. Such an alteration assemblage also likely affected the garnet-bearing quartzo-feldspathic gneisses from Green Mountain. Precursors to altered rocks from Horseshoe and Cinderella most probably contained chlorite, and sericite, while meta-amphibolite were likely weakly to moderately altered basaltic/andesitic rocks. Such a precursor assemblage is commonly associated with VMS deposits.

Figure 14. Chlorite-carbonate-pyrite index (CCPI) vs Ishikawa alteration index (AI) alteration box plot of Large et al. (Reference Large, Gemmell, Paulick and Huston2001) for samples from the Dawson-Green Mountain trend (modified after Large et al. Reference Large, Gemmell, Paulick and Huston2001). Central box indicates least altered samples for felsic, intermediate and mafic rocks, while the arrows show the alteration trend.
The relatively narrow range of sulphur isotopic compositions (δ34S = −3.3 to +6.5‰) for the metamorphosed massive sulphide deposits in Colorado (Fig. 13), which is centred around +1‰ suggests a magmatic contribution of sulphur to the deposits. Although it should be noted here that such an isotopic range does not preclude the previously mentioned genetic models, we suggest here that sulphur was likely leached from precursor clastic sedimentary or volcaniclastic piles. Metasedimentary/metavolcaniclastic rocks are free of organic material (i.e. graphite) suggesting that isotopic fractionation was not the result of bacteriogenic sulphate reduction. Instead, thermochemical sulphate reduction (TSR) is the most likely cause of the isotopic fractionation assuming equilibrium among the sulphides (pyrite, pyrrhotite, chalcopyrite and locally galena). It is also a reasonable assumption that sulphides were in equilibrium prior to metamorphism since banded sulphides in metamorphosed ore deposits at, for example, Vulcan are probably the result of syngenetic processes rather than due to metamorphism or deformation (Dudley & Bruekner, Reference Dudley, Bruekner and Christie2022). Isotopic variations are likely due to small differences in physicochemical conditions (i.e. T, fO2, pH, ionic concentration of the ore fluid (I) and δ34SΣS; Ohmoto, Reference Ohmoto1972), rather than to differences in lithological composition. Due to the effects of metamorphism, the T, fO2, pH, I and δ34SΣS of the pre-metamorphic ore-forming hydrothermal fluid can only be estimated. Based on the mineralogy of the ore and the likely precursors to the metamorphosed altered rocks, some approximations can be made when considering the systems Cu-Fe-S-O and K-Al-Si-O-H, which are relevant to the massive sulphide systems in Colorado. Based on the solubility of chalcopyrite and its common presence in nearly all of the massive sulphide deposits, we assume a minimum temperature of the ore fluid of ∼270 oC (e.g. Large, Reference Large1992). The presence of the alteration assemblages muscovite-quartz-pyrite and chlorite-muscovite quartz at Vulcan, along with precursors to the alteration assemblage gedrite/anthophyllite-cordierite-bearing rocks at most deposits, suggests that, based on the system K-Al-Si-O-H, the ore fluid is largely confined to the muscovite stability field. Pyrite-pyrrhotite is the most common assemblage in the system Fe-S-O with pyrite-pyrrhotite-magnetite being present in some deposits (e.g. Dawson, Green Mountain, El Plomo). In a plot of logfO2-pH (Fig. 15), the sulphur isotope compositions for pyrite (given that it is the most common sulphide analysed herein) overlap the stability fields of muscovite, pyrite-pyrrhotite and pyrite-pyrrhotite-magnetite would require T = ∼350 oC, I = ∼1 m NaCl (approximate seawater salinity), a total dissolved S content of ∼0.1 moles/kg H2O and δ34SΣS = +1‰. Varying these parameters significantly does not allow an overlap between the sulphur isotope compositions with the aforementioned stability fields. For example, if it is assumed that the S in solution was derived from the isotopic reduction of sulphate in the global ocean, which at around that time (i.e. ∼1.8 to 1.7 Ga), was δ34S= ∼20‰ (Canfield & Farquar, Reference Canfield and Farquar2009), or that the ore-forming fluid had a T of <300 oC, the sulphur isotope values observed here for pyrite do not overlap the stability fields of muscovite and members of the system Fe-S-O. The assumptions made here are approximate at best and do not allow for changes as fluid temperatures decreased or the likely possibility of some interaction of the hydrothermal fluid with seawater. The most likely source of sulphur are the volcanic rocks (either mafic or felsic or both) with possible leaching of sulphur from the spatially associated sedimentary/volcaniclastic rocks surrounding the deposits.

Figure 15. A logfO2-pH diagram for massive sulphides from Colorado. Sulphur isotope contours for sphalerite are drawn for δ34S= +1‰ and T = 350 oC. Minerals in the system Fe-S-O are shown for ΣS = 0.1 moles/kg H2O as red dashed lines. The shaded region shows the approximate range of conditions for δ34S of sphalerite over fO2-pH range indicated (primarily along the pyrrhotite-magnetite join. Note that the shaded area starts at the pyrite-magnetite-pyrrhotite triple point to accommodate the rare presence of primary pyrite. Modified after Ohmoto (Reference Ohmoto1972).
As a further attempt to determine the genesis of the deposits, we evaluated the Zn/Cd ratios of sphalerite. Wen et al. (Reference Wen, Zhu, Zhang, Cloquet, Fan and Fu2016) proposed that the composition of sphalerite can be used as a way to classify different types of Pb-Zn deposits and pointed out that the Cd content of sphalerite is dependent on T, pH, the nature and concentration of ligands in the ore fluid that bonds to Zn and Cd, and the total sulphur in solution. Wen et al. classified Pb-Zn deposits into three groups: low temperature (i.e. MVT deposits), high temperature (i.e. porphyry, magmatic-hydrothermal, skarn and VMS deposits) and exhalative systems (i.e. Sedex and seafloor hydrothermal sulphides). The classification scheme was based on Cd concentrations and Zn/Cd ratios in sphalerite as well as a plot of Cd isotopes vs. Zn/Cd ratios. Although the metamorphosed massive sulphide deposits studied here are mostly Cu-Zn deposits, galena is a minor sulphide in nearly all deposits and is common in the El Plomo deposit and locally in the Cinderella deposit. While Zn/Cd ratios have been used in the classification of Pb-Zn deposits by Wen et al. (Reference Wen, Zhu, Zhang, Cloquet, Fan and Fu2016), the Zn/Cd ratios of sphalerite in the Vulcan, Horseshoe, El Plomo and Dawson deposits fall within the overlap region of VMS and Sedex deposits identified by Spry et al. (Reference Spry, Mathur, Teale and Godfrey2022a) (Supplementary Appendix Fig. S3, Supplementary Table Appendix S2a). The reason for this is unclear. However, it may be reflecting both VMS and Sedex characteristics since the VMS deposits are hosted in metasedimentary/metavolcaniclastic rocks.
8.c. Exploration guides to metamorphosed massive sulphide deposits in Colorado
Previous studies by Spry and Scott (Reference Spry and Scott1986), Spry and Petersen (Reference Spry and Petersen1989), Heimann et al. (Reference Heimann, Spry and Teale2005), and Spry et al. (Reference Spry, Peters and Slack2000, Reference Spry, Mathur, Teale and Godfrey2022a) show that the presence of iron formations, nodular sillimanite rocks, orthoamphibole-cordierite-bearing rocks, quartz garnetite, and gahnite- and högbomite-bearing rocks can be used as potential exploration guides to metamorphosed massive sulphide deposits. Willner et al. (Reference Willner, Schreyer and Moore1990) also considered that topaz- and rutile-bearing gneisses can be used as exploration guides to sulphide deposits. Such rocks were identified by Heimann et al. (Reference Heimann, Spry, Teale and Jacobson2006) in the Evergreen area (along with orthoamphibole-cordierite garnet rocks) and elsewhere in Colorado by Marsh & Sheridan (Reference Marsh and Sheridan1976), Fisher et al. (Reference Fisher, Kleinhans and Fisher2022) and Kleinhans et al. (Reference Kleinhans, Alers, James, Piper and Fisher2022). All of these rocks have been observed in Colorado and serve as pathfinders to ore. Moreover, the composition of zincian spinels and zincian högbomite have compositions that are compatible with these minerals having formed by desulphidation reactions in most locations supporting the concept that their presence may be indicative of nearby sulphides (see Spry, Reference Spry2000; Heiman et al. Reference Heimann, Spry and Teale2005).
9. Conclusions
The main conclusions of the present study are as follows:
-
1. Semimassive to massive sulphide deposits in central Colorado occur in a volcanic arc setting that were subsequently metamorphosed anywhere from the upper greenschist to upper amphibolite-granulite facies. They likely formed around 1.8 to 1.7 Ga. Our preferred genetic model is for the deposits to be VMS deposits with bimodal-mafic deposits occurring in the Gunnison area and mafic-siliclastic deposits dominating other locations in Colorado including the DGMT. This is due to the dominance of felsic and mafic igneous rocks at Gunnison and the presence of metamorphosed volcaniclastic rocks with a predominance of metamafic intrusive rocks (amphibolite and gabbro) associated with other deposits. There appears to be no genetic relationship of sulphide deposits to granitoids even though they are spatially related to most deposits.
-
2. Although Zn/Cd ratios of sphalerite do not distinguish among the previously proposed genetic models, sulphur isotope compositions are consistent with a magmatic source of sulphur that reflects TSR, rather than biogenic reduction processes. These isotopic compositions, when coupled with the nature of the precursor minerals in the metamorphosed altered rocks and gangue minerals, along with those in the system Fe-S-O, suggest the following approximate conditions of ore formation: T = ∼350 oC, I = ∼1 m NaCl, a total dissolved S content of ∼0.1 moles/kg H2O and δ34SΣS = +1‰.
-
3. Zincian spinels occur in various rocks types in Colorado including non-sulphide bearing metasiliclastic and metavolcaniclastic rocks, but they are mostly found in sulphide-bearing metamorphosed altered rocks or in sulphide-rich assemblages. The new data obtained here are consistent with the highly variable compositions reported previously by Heimann et al. (Reference Heimann, Spry and Teale2005) from other deposits in Colorado. Where the sulphide-bearing metamorphosed altered rock contains a paucity of magnesian silicates, the spinel is dominated by the gahnite molecule, which is dictated by temperature and the buffering capacity of sphalerite and Fe sulphides. However, where the rock contains an abundance of Mg-rich silicates (e.g. biotite, ortho- and calcic-amphiboles, chlorite, cordierite) and disseminated sulphides, the composition of the zincian spinel has a higher proportion of spinel sensu stricto molecule since the Mg component of zincian spinels is not buffered by fS2.
-
4. The distribution of nodular sillimanite rocks, gahnite-bearing rocks and rutile-topaz rocks constitutes zones of metamorphosed stratabound hydrothermal alteration, while iron formations and quartz garnetite serve as exhalites/inhalites. They, along with metamorphosed altered rocks dominated by orthoamphiboles, cordierite and gahnite±högbomite, serve as exploration guides to metamorphosed massive sulphide deposits in Colorado and elsewhere in the world.
Supplementary material
The supplementary material for this article can be found at https://doi.org/10.1017/S0016756823000407
Acknowledgements
This study was financially supported by Zephyr Minerals and by grants to EB from the Rocky Mountains Association of Geologists Foundation and the Society of Economic Geologists Foundation. Discussions with Will Felderhoff, Mark Graves and Loren Komperdo of Zephyr Minerals along with Trevor Van Dyke, Stan Keith and Monte Swan about the geology of the Dawson-El Plomo-Green Mountain deposits, are greatly appreciated. Evelyne Leduc (QFIR) is thanked for providing sulphur isotope standards, while James Hagadorn and Nicole Neu-Yagle (Denver Museum of Nature and Science) and Karen Kelley (US Geological Survey) kindly provided samples for the sulphur isotope study. Justin Glenn assisted with drafting of some figures. The reviews of Bertus Smith and an anonymous reviewer are greatly appreciated and improved the quality of the manuscript. Tim Johnson is also thanked for his comments and editorial handling of the paper.