Accumulating evidence suggests that long-chain PUFA (LC-PUFA) plays an important role in promoting brain growth and development( Reference Cheatham, Colombo and Carlson 1 ). DHA derived from α-linolenic acid (ALA; n-3) as well as arachidonic acid (ARA) derived from linoleic acid (LA; n-6) are now surfacing as the key modulators of fetal growth and brain development. The brain is highly enriched with LC-PUFA, with DHA and ARA being the most abundant and representing approximately 20 % of total fatty acids( Reference Sinclair 2 ). Accretion of DHA in brain phospholipids occurs actively during the developmental period, which is termed as the ‘brain growth spurt’( Reference Clandinin, Chappell and Leong 3 ). In rats, DHA accumulates mainly during the last 3 d of gestation and continues throughout weaning( Reference Green and Yavin 4 ). Availability of both DHA and ARA during this period is therefore crucial for the fetus and the neonate.
The fetus and neonate have limited capacity to synthesise DHA and ARA( Reference Bowen and Clandinin 5 ). Therefore, the mother is the primary source of these fatty acids for the fetus during the intra-uterine period and for the neonate in early postnatal life. Maternal stores of these conditionally essential fatty acids depend on the dietary supply of preformed DHA, ARA and their precursors, i.e. LA and ALA. Placenta and breast-milk are involved in the transfer of maternal DHA and ARA to the growing conceptus. Dams on control (C) diets supplied with varying ratios of LA:ALA can synthesise adequate DHA and ARA( Reference Cheon, Huh and Lee 6 ). Further, it is the maternal protein deficiency that adversely affects LC-PUFA metabolism( Reference Burdge, Dunn and Wootton 7 ) and not the maternal energetic restriction during pregnancy( Reference Agale, Kulkarni and Ranjekar 8 ). In fact, maternal low-protein diets (9 % protein) have been shown to decrease maternal hepatic and plasma DHA levels due to reduced activity of desaturase enzymes, thus limiting their transfer to the fetus and their consequent accretion in fetal brain lipids( Reference Burdge, Dunn and Wootton 7 ). These effects are shown to be persistent even after weaning, signifying the importance of in utero nutrition( Reference Burdge, Delange and Dubois 9 ).
Studies( Reference Levant, Radel and Carlson 10 – Reference Lim, Hoshiba and Salem 12 ) have examined the effects of LC-PUFA supplementation in n-3-deficient progeny on brain fatty acid composition and noted that reversal of deficiency is best achieved in neonatal and lactating pups than adult rats. However, often the reported supplementation studies are carried out at C protein levels of 18–20 %. Further, these findings are supported by studies in term infants( Reference Carlson, Ford and Werkman 13 , Reference Jensen, Voigt and Prager 14 ) where infant formulae with DHA and ARA improved their visual and neurodevelopment indices indicating the significance of optimal maternal LC-PUFA intake during lactation as well.
These findings assume greater importance in developing countries like India where a large part of the population is strictly vegetarian, most maternal diets in poor communities are inadequate in proteins and grossly deficient in energy, limiting the functional use of available dietary proteins. Consequently, the increased requirements of DHA and ARA during pregnancy and lactation may not be adequately met. It is therefore worthwhile to investigate whether in such situations supplementation with a direct source of DHA and ARA proves beneficial for brain fatty acid accretion of offspring. As mammary glands are shown to actively participate in LC-PUFA synthesis right from late gestation and throughout lactation by expressing desaturases and elongases( Reference Rodriguez-Cruz, Sánchez and Sánchez 15 ) it is worthwhile to examine simultaneously the fatty acid profile of dam milk. The present study thus aimed to examine the possible effects of maternal LC-PUFA supplementation in protein-restricted dams during prenatal and postnatal periods on changes in milk and brain fatty acid profile in progeny using an animal model.
Materials and methods
All experimental procedures were in accordance with the guidelines of the Institutional Animal Ethical Committee. Animal maintenance and handling were in accordance with the Indian National Institute of Nutrition guidelines( Reference Raghuramulu, Nair and Kalyansundaram 16 ).
Diets
All diets were formulated according to American Institute of Nutrition (AIN)-93G standards, which have been recommended for pregnant and lactating female rats( Reference Reeves, Nielsen and Fahey 17 ). The composition of the C diet was the same as AIN-93G and contained 18 g protein/100 g diet (Table 1). We formulated two iso-energetic treatment diets low in protein (9 g/100 g diet) by increasing the amount of maize starch to 48·8 g/100 g diet. Of these diets, one (low protein (LP)) had soyabean oil (7 %) while the other (low protein DHA + ARA supplemented (LPS)) had a combination of single cell oils containing 40–45 % of DHA and ARA (Martek Biosciences) with soyabean as the basal oil. The percentages of these single cell oils were calculated based on oral diet safety studies( 18 , Reference Blum, Kiy and Tanaka 19 ). All three diets contained tertiary-butyl-hydroquinone as an antioxidant. The single cell oils also had added antioxidant. Special attention was given while processing the LPS diet, which was dried at room temperature (30°C) to avoid the oxidation of added oils. The dry pellets were stored at refrigeration temperature (4°C) and used within 1 week.
Table 1. Composition (g/100g) of control and experimental diets (Mean values and standard deviations)
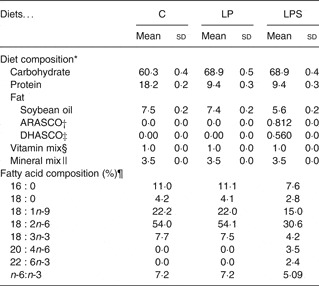
C, control diet; LP, low-protein diet; LPS, low-protein diet supplemented with DHA and arachidonic acid.
* Proximate analysis (ten batches of diets per group).
† ARASCO, Arachidonic acid single cell oil (Martek Biosciences).
‡ DHASCO, Docosahexaenoic acid single cell oil (Martek Biosciences).
§ Mineral mixture (g/kg mix): calcium carbonate 357, potassium phosphate 196, potassium citrate 70·78, sodium chloride 78, potassium sulfate 46·6, magnesium oxide 24, ferric citrate 6·06, zinc carbonate 1·65, manganous carbonate 0·63, cupric carbonate 0·3, potassium iodate 0·01, sodium selenate 0·01, ammonium paramolybdate 0·007, sodium metasilicate 1·45, chromium potassium sulfate 0·275, lithium chloride 0·01, boric acid 0·08, sodium fluoride 0·06, nickel carbonate 0·03, ammonium vandate 0·006, sucrose 221·02
|| Vitamin mixture (g/kg mix): nicotinic acid 3, calcium pantothenate 1·6, pyridoxine-HCL 0·7, thiamin-HCL 0·6, riboflavin 0·6, folic acid 0·2, d-biotin 0·02, vitamin B12 (0·1% in mannitol) 2·5, vitamin E15, vitamin A 0·8, vitamin D 0·25, vitamin K 0·075, sucrose 974·655.
¶ Fatty acid composition of extracted fat was analysed by gas chromatography, expressed as median values.
Experimental design
The animal house at Agharkar Research Institute (Pune, India) is registered under the Committee for the Purpose of Control and Supervision of Experiment on Animals, India, and follows vigorously all instructions for adequate animal care. All the rats were pathogen free. Nulliparous female Wistar rats weighing 200–250 g were obtained from the in-house colony of the institute. They were then bred in a ratio of three females:one male. The animals were maintained at 22°C on a controlled 12 h light and 12 h dark cycle with an appropriate ventilation system. Rice husk was used as bedding material and was non-nutritive and non-toxic. The pups born were separated according to sex on day 22. A total of thirty female pups were randomly selected for the experimental protocol. When they reached body weights of 200 g they were kept for breeding. Mating was confirmed by sperm-positive vaginal smear. On confirmation of pregnancy, i.e. 0 d of gestation, they were randomly allocated to one of the three diet groups (ten per group). In both the C and LP groups, one dam did not conceive and therefore the groups had nine dams. The dams received the dietary treatment during gestation (21 d) and lactation (21 d).
Observations recorded
Daily food intake during pregnancy and weekly body weights were recorded for dams in all three groups. Spontaneous delivery took place on day 22. Dam weights after parturition, litter size and litter weights at birth were recorded. Large litters were reduced to eight pups on day 1 to ensure a standard litter size per dam.
Brain lipid fatty acid composition
These analyses were conducted on total brain lipids. Brain fatty acids were analysed for a sub-sample of pups at postnatal day 1 and during lactation (day 14). Fresh whole brains were collected from anaesthetised pups and cryo-frozen at −80°C. Hydrolysis and transesterification of lipids were carried out according to the method of O'Fallon et al. ( Reference O'Fallon, Busboom and Nelson 20 ). Heptanoic acid (17 : 0; Sigma) was used as an internal standard. The methyl esters were extracted with hexane and the fatty acid composition of the extract was analysed by GC using Shimadzu model GC-17A Version 3 fitted with capillary column SPB 35. The analysis conditions were as follows: capillary column, 30 m × 0·32 mm inner diameter, film thickness, 0.25 μm. The temperature programme was as follows: the initial temperature 100°C (5 min hold), 100–220 °C (10°C per min). GC parameters were: injector temperature 200°C, detector temperature 220°C, flame ionisation detector, carrier gas He; flow rate 1·8 ml/min; split ratio 33:1.
Milk fatty acid composition
For milk collection, pups were separated from their dams after providing a sufficient time for nursing the young ones. On day 1 of lactation, two pups/litter/diet group were randomly selected for collection of gastric milk samples. On day 14 of lactation too, the gastric milk sample from one pup/litter/diet group was randomly selected. Before collection of milk, pups were exposed to anaesthetic ether and then milk was obtained from the stomach and the collected samples were cryo-frozen at −80°C. The milk lipids were extracted and transesterified according to the method of Christopherson & Glass( Reference Christopherson and Glass 21 ) and analysed by GC using Shimadzu model GC-17A Version 3 fitted with capillary column SPB 35. The analysis conditions were as follows: capillary column, 30 m × 0·32 mm inner diameter, film thickness, 0.25 μm. The temperature programme was as follows: initial temperature 40°C (5 min hold), 40–100°C (6°C/min) and 100–220°C (10°C/min). The injector temperature was 200°C, and the detector temperature was 220°C. The detector was a flame ionisation detector. The carrier gas was He with a flow rate of 1·8 ml/min and a split ratio of 33:1. Fatty acid concentrations were calculated from their relative peak areas as compared with the internal standard. Retention times and peak areas were automatically computed by GC. Individual fatty acids were identified by comparing the retention time of the peak with that of standard fatty acid methyl esters (37-component FAME Mix; Sigma). Fatty acids were quantified as percentage total fatty acids in the sample lipids.
Statistical methods
Data are presented as means and standard deviations. The treatment groups were compared with the C group by one-way ANOVA and the post hoc Bonferroni test was applied to make pair-wise comparisons. The Kruskal–Wallis independent sample non-parametric analysis was also applied to test significance in the case of small sample size (n < 30), for the comparison of brain and milk fatty acids profiles. The data were analysed using the SPSS/PC+ package (Version 11.0; SPSS Inc.).
Results
Dams' food intake and body weight gain during pregnancy
Despite differences in protein levels, there was no difference in food intake during pregnancy (Table 2) and lactation among the dams from the C and treatment groups. The total weight gain during pregnancy was the highest in the dams from the C group; however, it did not differ significantly from those in the LP and LPS groups (Table 2).
Table 2. Effect of dietary treatment on food consumption, body weight gain and reproductive performance of control diet-fed (C) and experimental dams (Mean values and standard deviations)
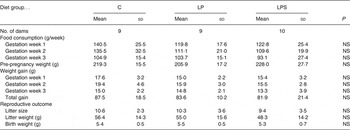
LP, low-protein diet; LPS, low-protein diet supplemented with DHA + arachidonic acid.
Reproductive performance
There was no difference in length of gestation between the C and treatment groups. The day of parturition (day 22) was similar between groups. Diet had no effect on litter size and litter weights at birth (Table 2).
Brain fatty acid composition of pups
The fatty acid composition of pups' brains on postnatal day 1 and during lactation (day 14) is shown in Table 3. At birth, there was no difference in the level of SFA between groups; however, the level of MUFA was significantly higher in brains of pups from the LP group compared to pups from the C and LPS groups. Among the essential fatty acids, the level of LA was similar between groups but that of ALA was significantly higher in pups of the LPS group. Among the supplemented fatty acids, the level of ARA in brain lipids did not differ between groups, but DHA was significantly higher in the pups from the LPS group. In addition, pups from this group had a significantly higher amount of EPA (20 : 5n-3) and total n-3 and lower level of n-6 docosapentaenoic acid (22 : 5) as compared with the LP group. Consequently, the n-6:n-3 ratio for brain fatty acids from LPS pups was significantly lower than for pups from the C and LP groups.
Table 3. Brain lipid fatty acids expressed as percentage total fatty acids of neonatal offspring of dams fed the control diet (C) and experimental diets (Mean values and standard deviations)
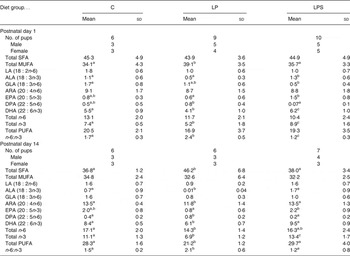
LP, low-protein diet; LPS, low-protein diet supplemented with DHA + arachidonic acid; LA, linoleic acid; ALA, α-linolenic acid; GLA, γ-linolenic acid; ARA, arachidonic acid; DPA, docosapentaenoic acid.
a,b,c Mean values within a row with unlike superscript letters were significantly different (P<0·05; preplanned comparisons using post hoc tests).
By mid-lactation (day 14), the percentage of ARA and DHA in brain lipids of pups increased significantly in all diet groups (Fig. 1). Likewise, a significant accretion of total PUFA was noted across all treatment groups. However, compared with pups from the C and LPS groups, brain lipids of pups from the LP group not only had significantly higher SFA (mainly palmitic; 16 : 0 29·73 % and stearic; 18 : 0 5·82 % compared with 26·18 and 5·07 % in pups from the C group) but also had markedly higher levels of docosapentaenoic acid, which is a marker of n-3 fatty acid deficiency. On the other hand, with regard to essential fatty acids, LPS pups continued to accrue higher levels of ALA, ARA, EPA, DHA and total n-3 fatty acids as compared with LP pups.
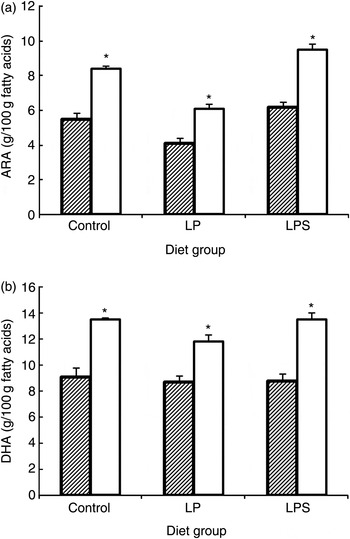
Fig. 1. Effect of dietary treatment on accretion of arachidonic acid (ARA) (a) and DHA (b) in whole brain phospholipids of offspring during the early postnatal period (///, day 1; □, day 14). Values are means, with standard errors represented by vertical bars (six to ten rats per group). * Mean value was significantly different from that at day 1 (P < 0·05; preplanned comparisons). LP, low-protein diet; LPS, low-protein diet supplemented with DHA + ARA.
Milk fatty acid composition of dams during lactation
The fatty acid composition of dams' milk at the beginning of lactation (day 1) and mid-lactation (day 14) is shown in Table 4. On day 1, there was no difference in the levels of SFA, MUFA or essential fatty acids, namely LA and ALA, between groups. However, the level of SCFA and medium-chain fatty acids (C4-C12) was significantly lower while the levels of both supplemented fatty acids (ARA and DHA) were significantly higher, resulting in a higher amount of total n-3 and PUFA in the milk samples of dams from the LPS group than those from the C and LP groups. The n-6:n-3 ratio of milk was the highest in the dams from the LP group.
Table 4. Milk fatty acids expressed as percentage total fatty acids during early and late lactation in dams fed the control diet (C) and experimental diets* (Mean values and standard deviations)
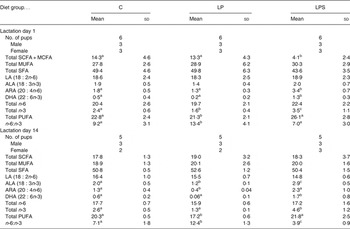
LP, low-protein diet; LPS, low-protein diet supplemented with DHA + arachidonic acid; MCFA, medium-chain fatty acids; LA, linoleic acid; ALA, α-linolenic acid; ARA, arachidonic acid.
a,b,c Mean values within a row with unlike superscript letters were significantly different (P<0·05; preplanned comparisons using post hoc tests).
* Post-nursing gastric milk samples collected from pup stomachs.
By mid-lactation (day 14) the percentage of SCFA and medium-chain fatty acids increased significantly in the milk of dams from the LPS group (4·1–18·3; P = 0·00) and nullified the between-group differences observed on day 1 of lactation. Among the essential fatty acids, ALA, ARA, DHA, total n-3 and PUFA content was the highest in the milk of dams from the LPS group, but was the lowest in the case of dams from the LP group. Within each dietary group, a reduction in DHA content of milk from day 1 to day 14 was observed but was maximum in the case of the LP group (0·2–0·06). A similar reduction was observed in the n-6:n-3 ratio, for both the C and LPS groups, but more sharply in the case of the latter while it remained constant in the LP group.
Correlation between maternal milk fatty acid and pup brain fatty acid composition
Correlations between dams' milk and pup brain fatty acids were computed for day 14 of lactation, by pooling the data for all diet groups (Table 5). We observed significant positive associations for the n-3 essential fatty acids, i.e. ALA but not for the n-6 essential fatty acids, namely LA. Further, a strong direct association was also noted for the respective n-3 LC-PUFA, i.e. DHA, but not for the n-6 LC-PUFA, i.e. ARA. However, the total n-3 and n-6 levels in milk and brain lipids showed significant positive associations as did the total PUFA content. One interesting observation was the significant inverse association between total SFA in maternal milk and total n-3 content of pup brains (r 2 −0·612; P < 0·05).
Table 5. Pearson correlation coefficients for dam milk and offspring brain fatty acids during mid-lactation (day 14)
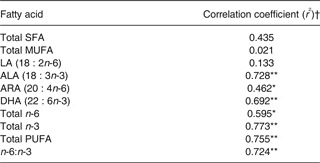
LA, linoleic acid; ALA, α-linolenic acid; ARA, arachidonic acid.
Significant one-tailed correlation: * P < 0·05, ** P < 0·01.
† Pooled sample for all diet groups: n 15.
Discussion
Maternal low protein intake during pregnancy and lactation has been shown to considerably perturb the normal metabolism of essential fatty acids, namely LA and ALA, resulting in poor ARA and DHA accumulation in the brain of growing offspring( Reference Burdge, Dunn and Wootton 7 , Reference Burdge, Delange and Dubois 9 ). Yet, there is paucity of studies investigating the effects of supplementation of LC-PUFA at low protein levels on changes in brain fatty acid content of the offspring and none have examined the consequent alterations in maternal milk profile. We observed that in conditions of protein restriction, supplemental DHA and ARA fed to dams led to their higher incorporation into pup brain lipids by altering the fatty acid profile of dams' milk.
Before discussing the important findings of the study it may be worthwhile to mention some of the points. Firstly, although the number of dams allocated to different diet groups was small, it was similar to those reported in earlier studies( Reference Cheon, Huh and Lee 6 , Reference Burdge, Dunn and Wootton 7 , Reference Saste, Carver and Stockard 22 , Reference Joshi, Rao and Girigosavi 23 ). Secondly, the number of pups dissected at day 0 and day 14 was modest as it was necessary to maintain a sizeable number of pups/group till adulthood, being a long-term study. Nevertheless, non-parametric tests were used for comparing differences in fatty acid profiles.
We observed that even at the low protein level of 9 %, with or without supplementation, litter size or litter weight did not differ significantly from that of the C group. Our observations are in agreement with reports from earlier studies that used diets containing menhaden fish oil( Reference Saste, Carver and Stockard 22 ) or used a large range of LA/ALA ratios (1·07–10·35) and did not find differences in length of gestation, litter size or pup weights between C and treatment groups( Reference Cheon, Huh and Lee 6 ). Even a protein-restricted diet with supplemental fish oil fed to pregnant dams did not show adverse effects on gestation, litter size or pup weight( Reference Joshi, Rao and Girigosavi 23 ). This has been attributed to the fact that in rats, a considerable part of growth and development occurs after birth than in utero, making them less susceptible to unfavourable nutrient situations while in the womb( Reference Widdowson and McCance 24 ).
The rate of accumulation of DHA and ARA in the rat brain is the highest during early postnatal life and supply of these fatty acids during brain growth can boost the rate of brain mass expansion( Reference Green, Glozman and Kamensky 25 ). We observed a significant increment in total brain ARA and DHA content from birth to 14 d of postnatal life in pups from all dietary groups; however, the highest accretion was noted in pups of LPS dams. Further, we also noted a strong positive correlation between maternal milk ALA, DHA, total n-3 content and brain n-3 fatty acid profiles, corroborating the hypothesis that maternal milk fatty acid content indeed determines pup brain fatty acid composition.
Two important and independent changes observed in milk fatty acid composition of dams from the LP and LPS groups are in need of attention. Firstly, in the case of dams from the LP group, despite comparable levels of n-6 LA in their milk, the milk was deficient in its long-chain metabolite, namely ARA as compared with its proportion in milk from dams in the C group. As mentioned earlier, it has been shown that mammary glands actively participate in LC-PUFA synthesis during lactation( Reference Rodriguez-Cruz, Sánchez and Sánchez 15 ), and so the protein-restricted diet may have down-regulated enzymes involved in desaturation and the elongation pathway of n-6 LA causing a reduction in the synthesis of ARA. Marin et al. ( Reference Marin, De-Tomas and Serres 26 ) and Burdge et al. ( Reference Burdge, Dunn and Wootton 7 ) have reported similar effects of feeding 10 % protein diets to pregnant and lactating rats on the metabolism of essential fatty acids in mammary glands.
The second notable observation was in the case of the LPS group which showed a significantly low proportion of SCFA and medium-chain fatty acids in dams' milk during initiation (day 1) of lactation. This may be due to their competitive replacement by supplemented LC-PUFA. However, by mid-lactation (day 14) a significant increase in these fatty acids made the levels comparable with those of the C group, indicating metabolic adaptations. For example, even in human subjects it has been shown that milk fatty acid composition undergoes changes during the course of lactation( Reference Sala-Vila, Castellote and Rodriguez-Palmero 27 ), which may be a feedback mechanism in order to meet the requirements for postnatal growth of the offspring. Our observation therefore suggests that examining alterations in milk fatty acid composition in the light of pup growth during lactation would be of immense importance.
Provision of preformed DHA and ARA to the pups through milk is crucial for optimal accretion of LC-PUFA in the rapidly growing brain of pups, as they are incapable of synthesising them from precursors( Reference Bowen and Clandinin 5 ). We observed that supplementation of preformed LC-PUFA to protein-restricted dams resulted in higher DHA and total n-3 fatty acids in their milk, unlike their non-supplemented LP counterparts. This observation thus calls attention to the significance of LC-PUFA supplementation at low protein levels in order to overcome the resulting deficiency due to poor inter-conversion of ALA to EPA and DHA and also to optimise the n-6:n-3 ratio of milk. Providing LC-PUFA during protein deficiency also served to conserve maternal ALA pools, possibly due to a lower amount of ALA entering the biosynthetic pathway, and enhanced EPA levels in milk by retro-conversion of DHA. This brought about a significant change in the total n-3 pools and n-6:n-3 ratio of LPS milk and led to superior accretion of DHA in the brains of lactating pups.
A large body of work suggests that an adequate supply of DHA during early life is essential for optimal growth and brain development. The present findings clearly demonstrate that low maternal dietary protein levels can profoundly affect DHA accretion in developing offspring due to n-3-deficient milk, and supplementing DHA and ARA in such a scenario can positively alter both maternal milk and pup brain fatty acid composition. The effect of the observed biochemical change on the functional capacity of the brain in the offspring needs to be evaluated. However, evidence from several supplementation studies in situations of adequate protein suggests that learning and cognitive behaviour are augmented with improvement in brain DHA status( Reference Garcia-Calatayud, Redondo and Martin 11 , Reference Ikemoto, Ohishi and Sato 28 , Reference Morigichi and Salem 29 ). Therefore, our findings in a protein-deficient condition assume greater importance owing to the fact that the effects of supplementation are more pronounced in conditions of deficiency. They emphasise the need for supplying adequate food sources of DHA to mothers during pregnancy and lactation to offset the negative effects of maternal protein deficiency on accretion of fetal brain DHA and subsequently brain growth.
Acknowledgements
The authors acknowledge the Director of Agharkar Research Institute Pune, India, for providing the facilities to carry out this research. Thanks are also due to the Head of the Chemistry Department, Animal Sciences Division, Agharkar Research Institute and Dr C. N. Dandge for their help with GC analysis. The authors are grateful to Martek Biosciences®, Canada for generously providing the experimental single cell oils. P. S. R. was supported by a Junior Research Fellowship of the University Grants Commission, India no. F15.6 - Dec 2005/2006 NET and UGC-REF. no. 204/NET - Dec 2005. The study was funded by Agharkar Research Institute. S. S. R. conceived and designed the study; P. S. R. conducted the study and analysed the data; S. S. R. and P. S. R. wrote the paper. P. S. R. had primary responsibility for the actual experiment and manuscript content. All authors contributed to, read and approved the final manuscript. The authors declare that there is no conflict of interest. The aforementioned work was presented in part at the 19th International Congress of Nutrition, October 2009, Bangkok, Thailand(30).