1. Introduction
The depth (and degree) of décollement within the continental crust during (collisional) deformation has been widely debated for many years (e.g. Pfiffner, Reference Pfiffner, Mazzoli and Butler2006). Two extreme opposing views have arisen through time for the interpretation of foreland fold–thrust belts (FTBs). One view states that the sedimentary cover is detached from the underlying basement along a shallow, low-strength décollement and deformed by thrusts with ramp-flat geometries rooting into the décollement (thin-skinned tectonic style). This thin-skinned style supposes large-scale displacements and duplication of the sedimentary sequence, the underlying basement remaining undeformed. The alternative view states that the basement is also involved in the deformation along crustal-scale ramps (thick-skinned tectonic style). A major difference between the two end-member models is that the amount of shortening is more conservative in a thick-skinned context, especially if inversion tectonics is involved (e.g. Butler et al. Reference Butler, Mazzoli, Corrado, De Donatis, Di Bucci, Gambini, Naso, Nicolai, Scrocca, Shiner, Zucconi and McClay2004).
The term thick-skinned is used hereinafter in a slightly different and somewhat broader sense than sometimes found in the literature. Compared to Rodgers (Reference Rodgers1949), for whom thin-skinned implies cover deformation only (part of the cover being decoupled from the basement), whereas thick-skinned implies deformation of both cover and basement (the cover remaining welded to the basement), we consider that the two styles of deformation are not mutually exclusive, i.e. both superimposed structural styles may occur coevally. We further do not distinguish between FTBs with a thin-skinned structural style and basement-involved belts with a thick-skinned structural style as done by Poblet & Lisle (Reference Poblet, Lisle, Poblet and Lisle2011), and we chose hereinafter to simply speak about thick-skinned FTBs. Finally, we do not restrict the use of the term thick-skinned to the cases where the entire crust is involved as suggested by Pfiffner (Reference Pfiffner, Mazzoli and Butler2006), and we also use it to describe thrust sheets made of upper crustal flakes only.
There are different ways to define the basement (e.g. Allaby & Allaby, Reference Allaby and Allaby1991; Holbrook, Mooney & Christensen, Reference Holbrook, Mooney and Christensen1992; Sheriff, Reference Sheriff2002). Structural geologists often define the basement as any rock, generally of crystalline nature, that underlies a specific stratigraphic cover sequence; in this case, the term ‘basement’ is used to describe the upper part of the crystalline crust. Geophysicists define the basement on the basis of reflectivity pattern or physical properties (e.g. seismic wave velocities, magnetic or gravimetric signature) to be compared to those of the overlying sedimentary rocks. When addressing the rheology of the material deformed in basement-involved, thick-skinned shortening one should theoretically distinguish the true crystalline basement from the overlying sedimentary cover sequence that remains welded to it. In practice, however, the contribution of the welded cover sequence – even bedded, hence expectedly anisotropic – to the bulk mechanical behaviour of the deformed material is quantitatively negligible, the latter being mainly controlled by the crystalline upper crustal rocks. In the following, the term ‘basement’ will refer to the true crystalline upper crust, but for the sake of clarity and exactness, possible deviation from this definition will be discussed specifically if needed (e.g. Taiwan).
Many FTBs exhibit both thin- and thick-skinned structural styles in different portions of the belt. Some, like the Andes, change style along-strike (e.g. Watts et al. Reference Watts, Lamb, Fairhead and Dewey1995; Allmendinger et al. Reference Allmendinger, Jordan, Kay and Isacks1997; Baby et al. Reference Baby, Rivadeneira, Barragan, Christophoul, Nemcok, Mora and Cosgrove2013; Carrera & Munoz, Reference Carrera, Munoz, Nemcok, Mora and Cosgrove2013; Iaffa et al. Reference Iaffa, Sabat, Munoz, Carrera, Nemcok, Mora and Cosgrove2013). Others, like the Rocky Mountains, exhibit a thin-skinned structural style in their interior (Sevier belt) and thick-skinned style (Laramide belt) in their exterior (e.g. Hamilton, Reference Hamilton, Schmidt and Perry1988). Nemcok, Schamel & Gayer (Reference Nemcok, Schamel and Gayer2005) and Nemcok, Mora & Cosgrove (Reference Nemcok, Mora, Cosgrove, Nemcok, Mora and Cosgrove2013) emphasized that 50% of orogens for which deformation styles are known show a thick-skinned character and have evolved from either passive margins or intra-cratonic rifts.
Thick-skinned FTBs mainly form at the expense of lower and/or upper plates during collision in ‘Alpine-type’ orogens, in pro-wedge and retro-wedge settings (e.g. Alps, Apennines, Carpathians, Balkans) (Fig. 1a) or within the upper plate of subduction orogens (retro-wedge setting: Rocky Mountains, Sierras Pampeanas of the Argentine Andes) (Fig. 1b). Thick-skinned FTBs are also encountered – even more rarely – in intraplate settings (e.g. North Sea region, High Atlas of Morocco; Teixell et al. Reference Teixell, Arboleya, Julivert and Charroud2003).
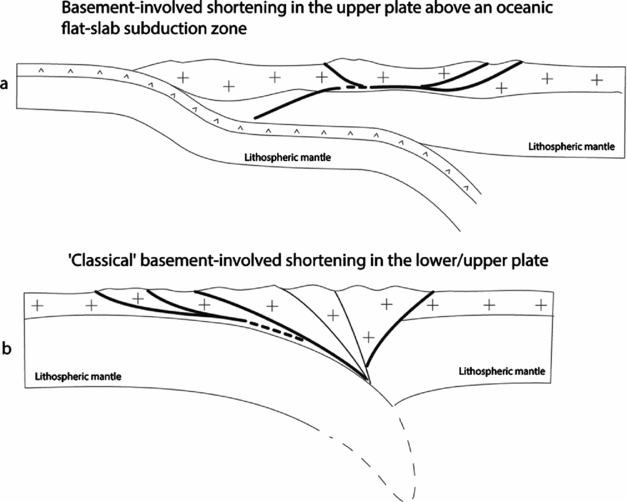
Figure 1. Main geodynamic and tectonic settings of basement-involved FTBs.
In areas where shortening was preceded by rifting, deep-rooting, inversion-dominated deformation likely prevailed during the building of FTBs. Reactivation/inversion of basement faults widely occurs during orogenic evolution of collided passive margins and inverted intracontinental rifts and this process is known to exert a strong control on the evolution of orogens (e.g. Jackson, Reference Jackson1980; Winslow, Reference Winslow, McClay and Price1981; Gillcrist, Coward & Mugnier, Reference Gillcrist, Coward and Mugnier1987; Roure et al. Reference Roure, Howell, Guellec, Casero and Letouzey1990; Letouzey, Reference Letouzey and Letouzey1990; Dechesne & Mountjoy, Reference Dechesne, Mountjoy, Mitra and Fisher1992; Coward, Reference Coward and Hancock1994; Berberian, Reference Berberian1995; Mitra & Mount, Reference Mitra and Mount1998; Coward et al. Reference Coward, De Donatis, Mazzoli, Paltrinieri and Wezel1999; Sandiford, Reference Sandiford1999; Brown et al. Reference Brown, Alvarez-Marron, Perez-Estaun, Puchkov and Ayala1999; Cristallini & Ramos, Reference Cristallini and Ramos2000; Kley & Monaldi, Reference Kley and Monaldi2002; Lacombe & Mouthereau, Reference Lacombe and Mouthereau2002; Mouthereau et al. Reference Mouthereau, Deffontaines, Lacombe, Angelier, Byrne and Liu2002; Mouthereau & Lacombe, Reference Mouthereau and Lacombe2006; Butler, Tavarnelli & Grasso, Reference Butler, Tavarnelli and Grasso2006; Carrera et al. Reference Carrera, Muñoz, Sábat, Mon and Roca2006; Scisciani, Reference Scisciani2009; Bonini, Sani & Antonielli, Reference Bonini, Sani and Antonielli2012; Alvarez-Marron et al. Reference Alvarez-Marron, Brown, Camanni, Wu and Kuo-Chen2014; Camanni et al. Reference Camanni, Brown, Alvarez-Marron, Wu and Chen2014).
Fault inversion is a complex and selective process that depends on the orientation and steepness of the fault plane, the friction along the fault plane and the contrast of this frictional strength with respect to that of the surrounding medium (Letouzey, Reference Letouzey and Letouzey1990; Sassi et al. Reference Sassi, Colletta, Bale and Paquereau1993; Sibson, Reference Sibson, Buchanan and Buchanan1995; Roure & Colletta, Reference Roure, Colletta, Ziegler and Horvath1996; Butler, Holdsworth & Lloyd, Reference Butler, Holdsworth and Lloyd1997; Holdsworth, Buick & Hand, Reference Holdsworth, Buick and Hand2001; Turner & Williams, Reference Turner and Williams2004; Butler, Tavarnelli & Grasso, Reference Butler, Tavarnelli and Grasso2006; Roure, Reference Roure2008; Scisciani, Reference Scisciani2009; Bonini, Sani & Antonielli, Reference Bonini, Sani and Antonielli2012; Bellahsen et al. Reference Bellahsen, Jolivet, Lacombe, Bellanger, Boutoux, Garcia, Mouthereau, Le Pourhiet and Gumiaux2012). Even when they are not reactivated, pre-existing faults are important mechanical discontinuities that can generate stress concentration and buttressing effects and often localize thrust ramps (Tricart & Lemoine, Reference Tricart and Lemoine1986; Gillcrist, Coward & Mugnier, Reference Gillcrist, Coward and Mugnier1987; Coward, Gillcrist & Trudgill, Reference Coward, Gillcrist, Trudgill, Roberts, Yielding and Freeman1991; Butler, Tavarnelli & Grasso, Reference Butler, Tavarnelli and Grasso2006; Mattioni, Sassi & Callot, Reference Mattioni, Sassi, Callot, Ries, Butler and Graham2007; Scisciani, Reference Scisciani2009; Cooper & Warren, Reference Cooper, Warren, Law, Butler, Holdsworth, Krabbendam and Strachan2010; Bonini, Sani & Antonielli, Reference Bonini, Sani and Antonielli2012; Bellahsen et al. Reference Bellahsen, Mouthereau, Boutoux, Bellanger, Lacombe, Jolivet and Rolland2014; Boutoux et al. Reference Boutoux, Bellahsen, Lacombe, Verlaguet and Mouthereau2014). Reactivated basement faults may induce localization of thrusts and folds in the developing shallow thrust wedge, development of basement uplifts or crystalline thrust sheets, out-of-sequence thrusting and refolding of shallow nappes or development of accommodation structures such as lateral ramps, among others.
In no longer active Cenozoic FTBs (e.g. Pyrenees–Provence, Rocky Mountains), thick-skinned tectonics can be documented by geophysical investigations (gravimetry, seismics) for buried structures and by structural investigations of basement uplifts and exposed basement-cored structures where the relationships between cover and basement can be deciphered, together with exhumed basement shear zones (e.g. Smithson et al. Reference Smithson, Brewer, Kaufman, Oliver and Hurich1979; Roure et al. Reference Roure, Choukroune, Berastegui, Munoz, Villien, Matheron, Bareyt, Seguret, Camara and Deramond1989; Stone, Reference Stone, Schmidt, Chase and Erslev1993; Schmidt, Genovese & Chase, Reference Schmidt, Genovese, Chase, Schmidt, Chase and Erslev1993; Roure & Colletta, Reference Roure, Colletta, Ziegler and Horvath1996; Fan & Carrapa, Reference Fan and Carrapa2014) (Fig. 2). In more recent and still active orogens (e.g. western Alps, Sierras Pampeanas, Zagros, Taiwan), the integrated approach combining geophysical (geodesy, gravimetry, seismics) and structural investigations can be complemented by seismological and thermochronological studies to document basement thrusting and uplift and to unravel structural style and occurrence of deep inversion tectonics (e.g. Mosar, Reference Mosar1999; Lacombe & Mouthereau, Reference Lacombe and Mouthereau2002; Camanni et al. Reference Camanni, Brown, Alvarez-Marron, Wu and Chen2014; Siame et al. Reference Siame, Sebrier, Bellier, Bourlès, Costa, Ahumada, Gardini and Cisneros2015) (Fig. 2).

Figure 2. Main characteristics of Tertiary and recent/active FTBs possibly documenting basement-involved, thick-skinned tectonics.
A brief review of some Cenozoic FTBs demonstrates that basement shortening may have various types of occurrence, later discussed in the sections of this paper. They include Oisans (western Alps) style, with distributed shearing within the basement reflecting basement underplating then frontal accretion/exhumation thanks to crustal thrust ramps (Fig. 3a; Bellahsen et al. Reference Bellahsen, Jolivet, Lacombe, Bellanger, Boutoux, Garcia, Mouthereau, Le Pourhiet and Gumiaux2012); Mont Blanc (western Alps) style, with stacking of crustal slices at the rear of the thin-skinned FTB as a result of basement underplating and localized exhumation then frontal accretion/exhumation thanks to crustal thrust ramps (Fig. 3b; Burkhard & Sommaruga, Reference Burkhard, Sommaruga, Mascle, Puigdefabregas, Luterbacher and Fernandez1998); Zagros style, with superimposed thin-skinned and thick-skinned tectonic styles (Fig. 3c; Mouthereau et al. Reference Mouthereau, Tensi, Bellahsen, Lacombe, Deboisgrollier and Kargar2007) or Sierras Pampeanas–Laramide style with the basement being involved within the foreland of the thin-skinned FTB (e.g. Laramide uplifts in the foreland of the Sevier belt and Sierras Pampeanas uplifts in the foreland of the Precordillera belt; Fig. 3d) (e.g. Hamilton, Reference Hamilton, Schmidt and Perry1988).

Figure 3. Examples of Cenozoic FTBs with different styles of basement-involved shortening. (a) Oisans (western Alps) style, with distributed shearing within the basement reflecting basement underplating then frontal accretion/exhumation thanks to crustal thrust ramps; (b) Mont Blanc (western Alps) style, with stacking of crustal slices at the rear of the frontal thin-skinned FTB as a result of basement underplating and localized exhumation then frontal accretion/exhumation thanks to crustal thrust ramps; (c) Zagros style, with superimposed thin-skinned and thick-skinned tectonic styles; (d) Sierras Pampeanas–Laramide style with the basement being involved in shortening in the foreland (Laramide/Sierras Pampeanas basement uplifts) of the thin-skinned FTBs (Sevier/Precordillera, respectively).
It thus comes that after two decades of fashionable interpretation of FTBs in terms of thin-skinned tectonics, there are increasing lines of evidence of basement-involved shortening in FTBs, even in the ‘archetypal’ thin-skinned belts. Recently, Mouthereau, Watts & Burov (Reference Mouthereau, Watts and Burov2013) proposed that FTBs can be gathered into two groups characterized by contrasting amounts of shortening and thermotectonic age of the lithosphere from which they developed. An important implication of this work could be that boundary conditions (plate kinematics, interplate coupling, synorogenic sedimentation, among others) may have little or no effect on the deformation style, being only controlled by the rheology of the lithosphere as inherited from the last thermotectonic (rifting) event before shortening. This view, however, does not take into account for instance those inner parts of the FTBs that possibly underwent syn-convergence, early collisional burial and heating before being shortened (e.g. Bellahsen et al. Reference Bellahsen, Mouthereau, Boutoux, Bellanger, Lacombe, Jolivet and Rolland2014).
This motivated this review of selected Cenozoic orogens worldwide, in an attempt at examining the evolution of ideas about FTBs and the extent to which the basement controls the geometry, kinematics and mechanics of foreland FTBs, and at addressing and evaluating in particular the role of crustal (lithospheric) strength, thickness and anisotropy, plate-tectonic setting, deposition leading to burial, depth to décollement and occurrence of pre-existing basement structures on basement-involved shortening, hence on the structural style in the outer parts of mountain belts.
2. Evolution of ideas and views about basement-involved deformation in FTBs: some examples
The overall geometry and mechanics of foreland FTBs are well accounted for by the Coulomb critical wedge model (Chapple, Reference Chapple1978; Davis, Suppe & Dahlen, Reference Davis, Suppe and Dahlen1983; Dahlen, Suppe & Davis, Reference Dahlen, Suppe and Davis1984; Stockmal et al. Reference Stockmal, Beaumont, Nguyen, Lee, Sears, Harms and Evenchick2007). This model describes FTBs as critically tapered wedges made of homogeneous deformable material moving above an undeformed substratum along a shallow, low-dipping décollement. Since Davis, Suppe & Dahlen's (Reference Davis, Suppe and Dahlen1983) seminal paper, critically tapered wedge mechanics has greatly influenced our view on the mountain-building processes, even though the model fundamentally meets the important restrictions that the deformable material of natural wedges is not homogeneous and that the substratum below the basal décollement horizon is not rigid and undeformable as assumed or implied by the theory.
The Coulomb theory has been stated in Davis, Suppe & Dahlen's (Reference Davis, Suppe and Dahlen1983) paper as to expectedly break down where the wedge thickens to the depth below which the basal resistance to sliding drops abruptly with increasing temperature (‘brittle–ductile’ transition). Although there is no theoretical limitation in applying the critically tapered wedge model to thick (-skinned) orogenic wedges – the bases of which extend down to and below the depth of the frictional to viscous transition – provided the theory is modified to take into account the temperature-dependent viscosity of crustal material at depth (e.g. Williams et al. Reference Williams, Connors, Dahlen, Price and Suppe1994; Mouthereau, Lacombe & Meyer, Reference Mouthereau, Lacombe and Meyer2006), this point has likely unconsciously hampered thinking equally in terms of thick-skinned tectonics and thin-skinned tectonics; the deformable material of the wedge has therefore often been considered to be restricted to the detached cover, which led to favouring thin-skinned tectonics.
As a result, in the 1980–90s structural sections across many outer parts of orogens were built, either explicitly or implicitly, assuming thin-skinned tectonic mechanisms. Because deformation of a sedimentary layer requires a lower amount of deviatoric yielding stress than deformation of the underlying crystalline basement rocks, thin-skinned tectonic style is usually expected at the thrust front of orogens, especially in the absence of well-oriented, pre-existing crustal weaknesses. As a consequence, in the absence of sound sub-surface evidence for basement involvement, thin-skinned deformation has generally been favoured with respect to the alternative thick-skinned mode, leading to often underestimating basement-involved shortening in the outer parts of orogens.
While in many cases surface geological structures are compatible with a model of shallow thrusting over a regional décollement in the sedimentary cover, a number of geological and geophysical data suggest that deeper levels of the crust are involved in the mountain-building process. In the following, we show on the basis of three famous FTBs the way our views and our understanding of the structural style have evolved.
2.a. Taiwan
The Taiwan orogen results from the active oblique collision of the young Chinese continental margin belonging to the Eurasian plate with the Luzon Arc that belongs to the oceanic Philippine Sea plate. The arc–continent collision started during late Miocene – early Pliocene times as dated by the beginning of the flexural subsidence in early Pliocene time (Chang & Chi, Reference Chang and Chi1983). This collisional evolution post-dated the Eocene–Oligocene continental rifting and subsequent spreading associated with the opening of the South China Sea, as well as later Miocene extensional events that affected the Chinese margin.
The Taiwan orogen is divided into several tectonostratigraphic belts. To the east, the Coastal Range, mainly composed of Neogene volcanic rocks interbedded with intra-arc Pliocene basin deposits, is the northern extension of the Luzon Arc. The rocks exposed in the Central Range belong to a metamorphic belt (Mesozoic Tananao Complex) or a slate belt (Eocene to middle Miocene rocks). The Central Range results from the exhumation and emplacement of a complex assemblage of ancient sedimentary basins, volcanic deposits and oceanic materials (Teng, Reference Teng1990). The Hsuehshan Range results from the inversion of the former extensional Hsuehshan Basin of the Chinese passive margin; it is made up of variably metamorphosed Eocene clastic sediments overlain by Oligocene shales and sandstones. The Western Foothills consist of a foreland FTB involving E-dipping imbricate thrust sheets. The thrust sheets are mainly composed of pre-tectonic Miocene shelf deposits of the Chinese platform. In addition, Pliocene–Pleistocene synorogenic sediments derived from the erosion of inner domains were deposited and incorporated into the propagating thrust units. To the west, the Coastal Plain consists of alluvial deposits poorly deformed at the surface (Figs 4, 8).

Figure 4. Examples of evolution of interpretations through time of two anticlines in the western Taiwan Foothills. Hsiaomei anticline (Central Taiwan): (a) Thin-skinned interpretation by Suppe & Namson (Reference Suppe and Namson1979) as a fault-bend fold; the pre-Miocene ‘basement’ of the Chinese continental margin is not involved in shortening. (b) Interpretation by Hung et al. (Reference Hung, Wiltschko, Lin, Hickman, Fang and Bock1999) as related to the reverse reactivation of the upper part of an inherited normal fault. In this view, the crystalline upper crust remains undeformed and the style of deformation is still to be considered as thin-skinned. (c) Thick-skinned interpretation by Lacombe & Mouthereau (Reference Mouthereau and Lacombe2006) modified after Yang et al. (Reference Yang, Huang, Wu, Lee, Ting and Mei2001). The anticline is related to basement-involved thrusting, i.e. the upper crust is involved in shortening. Shortening is much lower with the thick-skinned than with the thin-skinned interpretations. Chingtsaohu anticline (NW Taiwan): (d) Interpretation by Namson (Reference Namson1981) as a fault-bend fold. (e) Interpretation by Yang et al. (Reference Yang, Wu, Wickham, Ting, Wang and Chi1996) as related to shallow thrusting rooting within shallow décollement levels interacting with a high-angle thrust resulting from the reverse reactivation of an inherited normal fault of the Chinese passive margin.
Since Suppe's pioneering works in Taiwan (Suppe, Reference Suppe1976, Reference Suppe1980; Suppe & Namson, Reference Suppe and Namson1979; see also Namson, Reference Namson1984), and the first test and illustration of the critically tapered wedge mechanics with data from Taiwan (Davis, Suppe & Dahlen, Reference Davis, Suppe and Dahlen1983; Dahlen, Suppe & Davis, Reference Dahlen, Suppe and Davis1984), ideas about the interpretation of surface structures at depth have considerably evolved, thanks to both newly acquired sub-surface data and challenging of the thin-skinned conceptual model by alternative views. Suppe & Namson (Reference Suppe and Namson1979) built the first balanced section across the Hsiaomei anticline interpreted as a fault-bend fold (Fig. 4a). According to these authors, the growth of this anticline was due to sliding above the Miocene Talu shales: this horizon is connected through a ramp to an upper décollement lying in the Pliocene Chinshui shales. In this interpretation, the decoupling/décollement level lies at 3–4 km depth in agreement with a thin-skinned deformation, while the pre-Miocene rocks of the Chinese continental margin are not involved in shortening (Fig. 4a). A more recent seismic profile allowed Hung et al. (Reference Hung, Wiltschko, Lin, Hickman, Fang and Bock1999) to propose that the formation of the Hsiaomei anticline may well be related to the reverse reactivation of the upper part of an inherited normal fault (Fig. 4b), which requires that the basal décollement, if present, is much deeper that that predicted for the fault-bend folding model (at ~12 km depth within the pre-Miocene formations). In this view, however, the crystalline upper crust still remains undeformed. Finally, Yang et al. (Reference Yang, Huang, Wu, Lee, Ting and Mei2001) suggested that thrusting involving the pre-Miocene rocks may be responsible for the Hsiaomei anticline structure. This interpretation implicitly requires that thrusting is accommodated at a deep crustal level (Mouthereau & Lacombe, Reference Mouthereau and Lacombe2006; Fig. 4c). Noticeably, shortening deduced from the thin-skinned interpretation is 10 km, whereas the thick-skinned interpretation gives shortening of only ~3 km.
Another example is provided by the Chingtsaohu anticline in NW Taiwan, first interpreted by Namson (Reference Namson1981) as a fault-bend fold due to sliding below the Oligocene Wuchihshan Formation (Fig. 4d) in a thin-skinned style of deformation. Yang et al. (Reference Yang, Wu, Wickham, Ting, Wang and Chi1996) later reinterpreted the formation of this anticline as related to shallow thrusting rooting within shallow décollement levels such as the lower–middle Miocene Peiliao and Hopai–Tungkeng formations or the lower Pliocene Kueichulin Formation, and interacting with high-angle thrusting resulting from the reverse reactivation of an inherited normal fault of the Chinese passive margin (Fig. 4e).
These examples emphasize the extent to which geological cross-sections illustrate structural interpretations that reflect combinations of available data and the model-dependent understanding of structural style at a given time. The first interpretations of surface geology, strongly driven by the thin-skinned tectonic model, were progressively challenged by inversion tectonic and thick-skinned tectonic views which still satisfy surface geological constraints and better integrate the control by the pre-orogenic structural setting. As a result, there are at present two alternative interpretations of the deformation style in the Western Foothills of Taiwan. For some authors, the imbricate thrust system is connected to a shallow, gently E-dipping décollement near the top of the Miocene or at the base of the Pliocene synorogenic sediments (e.g. Suppe Reference Suppe1980; Carena, Suppe & Kao, Reference Carena, Suppe and Kao2002; Yue, Suppe & Hung, Reference Yue, Suppe and Hung2005; Brown et al. Reference Brown, Alvarez-Marron, Schimmel, Wu and Camanni2012), while for others there is extensive inversion tectonics and basement-involved shortening (e.g. Lee, Chang & Coward, Reference Lee, Chang, Coward, Byrne and Liu2002; Mouthereau et al. Reference Mouthereau, Deffontaines, Lacombe, Angelier, Byrne and Liu2002; Mouthereau & Lacombe, Reference Mouthereau and Lacombe2006, Yang et al. Reference Yang, Huang, Wu, Ting and Mei2006; Rodriguez-Roa & Wiltschko, Reference Rodriguez-Roa, Wiltschko, Goffey, Craig, Needham and Scott2010).
It should be noticed, however, that there is an ambiguity in the literature on what is called basement in westernmost Taiwan (Fig. 4). While some authors have defined the basement as any pre-Tertiary rocks (e.g. Ho, Reference Ho1986), others have called basement any pre-Miocene (undifferentiated) rocks (e.g. Mouthereau et al. Reference Mouthereau, Deffontaines, Lacombe, Angelier, Byrne and Liu2002; Mouthereau & Lacombe, Reference Mouthereau and Lacombe2006; Fig. 4). The latter rocks, drilled in a few places in the vicinity of the Peikang Plain and beneath the Penghu Islands (Chiu, Reference Chiu1973), are Cretaceous to Eocene in age and are mainly composed of arkoses and arkosic sandstones, while locally some mineral assemblages point to contact metamorphism due to plutonic intrusions (Chiu, Reference Chiu1973). Even though these variably consolidated pre-Miocene sedimentary rocks may be lithologically and mechanically different from true crystalline rocks, they, however, remained welded to the crystalline basement, so that the bottom of the Miocene section can be regarded as a good proxy for the top of the true basement (for a more detailed discussion, see Mouthereau et al. Reference Mouthereau, Deffontaines, Lacombe, Angelier, Byrne and Liu2002). As a consequence, the involvement in shortening of the pre-Miocene rocks must be considered as strongly indicative for true thick-skinned tectonics.
In Central Taiwan (see Section 3.b), recent studies have suggested that the structure and level of crustal involvement in inner parts of the belt may also significantly differ from a thin-skinned tectonic model (e.g. Wu, Rau & Salzberg, Reference Wu, Rau and Salzberg1997; Wu, Chang & Wu, Reference Wu, Chang, Wu, Malpas, Fletcher, Ali and Aitchison2004; Gourley et al. Reference Gourley, Byrne, Chan, Wu and Rau2007; Brown et al. Reference Brown, Alvarez-Marron, Schimmel, Wu and Camanni2012). This change seemingly takes place across the Shuilikeng fault, which bounds to the west the Hsuehshan Range (Camanni et al. Reference Camanni, Brown, Alvarez-Marron, Wu and Chen2014). The regional-scale structure of the Hsuehshan Range can be interpreted as a basement-cored anticlinorium (Brown et al. Reference Brown, Alvarez-Marron, Schimmel, Wu and Camanni2012) resulting from the inversion and shortening of the former marginal Hsuehshan Basin. There, field geology (e.g. rock ages, style and amount of deformation, level of exhumation), deep seismic events and high P-wave velocities (up to 5.5 km s−1) at shallow depths support basement-involved shortening and uplift of crystalline basement rocks at shallower depths than in the Western Foothills (e.g. Camanni et al. Reference Camanni, Brown, Alvarez-Marron, Wu and Chen2014).
As a result, the increasing availability of seismic profiles constrained by deep borehole data (e.g. Yang et al. Reference Yang, Huang, Wu, Ting and Mei2006) led to generalized positive inversion models for the western Taiwan FTB associated with likely basement involvement in shortening. In the inner part of the Taiwan belt, structural geology combined with geophysical investigations also document basement-involved shortening and positive inversion of former marginal basins. Seismic activity near the thrust front or eastwards, deeper than the originally inferred décollement, is likely caused by active thrust faulting distributed within the entire Eurasian continental crust (see Section 3).
2.b. Apennines
The Apennines extend from the Po Valley to the Calabrian arc and form the backbone of the Italian Peninsula (Fig. 5). The foredeep includes parts of the Po Valley in the north, part of the Adriatic Sea and, onshore in southern Italy, the Bradanic Trough. The Northern and the Southern Apennine arcs display contrasting outcropping structural levels, structural styles and kinematic histories (e.g. Satolli et al. Reference Satolli, Pace, Viandante and Calamita2014). The Northern Apennines consist of a FTB that developed during Cenozoic times, following the closure of an eastern branch of the Mesozoic Tethys Ocean (Ligurian ocean) and the collision of the European (Corsica–Sardinia block) and African (Adria block) continental margins.

Figure 5. Evolution of ideas about the structural style of the Umbria–Marches domain of the Northern Apennines (modified after Scisciani et al. Reference Scisciani, Agostini, Calamita, Pace, Cilli, Giori and Paltrinieri2014). (a) Thin-skinned tectonic style, with imbrication of sedimentary units detached along the Triassic evaporites, over an undeformed and buried basement (Bally et al. Reference Bally, Burbi, Cooper and Ghelardoni1986). (b) Thick-skinned tectonic style (Calamita et al. Reference Calamita, Coltorti, Piccinini, Pierantoni, Pizzi, Ripepe, Scisciani and Turco2000). (c) Thick-skinned tectonic style with imbricated array of gently dipping thrusts cutting through basement and sedimentary cover (Mirabella, Barchi & Lupattelli, Reference Mirabella, Barchi and Lupattelli2008). (d) Thick-skinned tectonic style with inversion of Permo-Triassic basins with relatively steeply dipping faults (Tavarnelli et al. Reference Tavarnelli, Butler, Decandia, Calamita, Grasso, Alvarez, Renda, Crescenti, D'Offizi, Merlini and Sacchi2004). (e) Thick-skinned tectonic style with deep-rooted basement-involved positive inversion of pre-existing extensional basins (Scisciani et al. Reference Scisciani, Agostini, Calamita, Pace, Cilli, Giori and Paltrinieri2014). Note the different shortening estimates and depth of basement between cross-sections.
During the 1980s, a thin-skinned tectonic style was proposed for the Apennines. This view was supported by seismic profiles (Bally et al. Reference Bally, Burbi, Cooper and Ghelardoni1986; Hill & Hayward, Reference Hill and Hayward1988), magnetic anomaly mapping of Italy showing a SW-dipping regional monocline in the axial Northern Apennines at a depth of 10–15 km (Arisi Rota & Fichera, Reference Arisi Rota and Fichera1987), as well as the absence of outcropping crystalline basement in the outer part of the Apennines, together with the presence of evaporites towards the base of the sedimentary cover. These thin-skinned models imply that substantial displacements have carried allochthonous sheets over buried foreland successions.
However, Coward et al. (Reference Coward, De Donatis, Mazzoli, Paltrinieri and Wezel1999) questioned the validity of these models in parts of the belt. These authors suggested that thrusts extend to depth down into the basement without significant duplication of the sedimentary sequence. The interpretation of different seismic profiles (CROP 03, Barchi, Minelli & Pialli, Reference Barchi, Minelli and Pialli1998; Finetti et al. Reference Finetti, Boccaletti, Bonini, Delben, Geletti, Pipan and Sani2001) and the integration of surface and sub-surface datasets (Coward et al. Reference Coward, De Donatis, Mazzoli, Paltrinieri and Wezel1999; Butler et al. Reference Butler, Mazzoli, Corrado, De Donatis, Di Bucci, Gambini, Naso, Nicolai, Scrocca, Shiner, Zucconi and McClay2004) led to challenging thick-skinned models of the Northern Apennines (Finetti et al. Reference Finetti, Boccaletti, Bonini, Delben, Geletti, Pipan and Sani2001; Mirabella, Barchi & Lupattelli, Reference Mirabella, Barchi and Lupattelli2008) that appealed to structural inheritance (Tavarnelli et al. Reference Tavarnelli, Butler, Decandia, Calamita, Grasso, Alvarez, Renda, Crescenti, D'Offizi, Merlini and Sacchi2004; Scisciani et al. Reference Scisciani, Agostini, Calamita, Cilli, Giori, Pace and Paltrinieri2010; Calamita et al. Reference Calamita, Satolli, Scisciani, Esestime and Pace2011). The interpretation of a recent magnetic anomaly map of the Apennines–Adriatic foreland system showed that the basement of the foreland is involved in shortening through high-angle thrust ramps likely reactivating pre-existing extensional faults (Speranza & Chiappini, Reference Speranza and Chiappini2002). In the Umbria–Marches domain, a thick-skinned inversion tectonics model is consistent with both the limited amount of shortening and the remarkable structural elevation (Scisciani et al. Reference Scisciani, Agostini, Calamita, Pace, Cilli, Giori and Paltrinieri2014).
Inherited basement structures clearly exerted a strong control in the evolution of the Apennines (Tavarnelli, Reference Tavarnelli1997; Coward et al. Reference Coward, De Donatis, Mazzoli, Paltrinieri and Wezel1999; Butler, Tavarnelli & Grasso, Reference Butler, Tavarnelli and Grasso2006; Scisciani, Reference Scisciani2009; Calamita et al. Reference Calamita, Satolli, Scisciani, Esestime and Pace2011; Bonini, Sani & Antonielli, Reference Bonini, Sani and Antonielli2012; Pace & Calamita, Reference Pace and Calamita2014; Pace et al. Reference Pace, Scisciani, Calamita, Butler, Iacopini, Esestime and Hodgson2015). Field observations and sub-surface data gathered across the Apennines revealed that the steep Jurassic to Miocene normal faults were systematically truncated and transported by W-dipping Mio-Pliocene thrusts in the Northern Apennines (Bonini, Sani & Antonielli, Reference Bonini, Sani and Antonielli2012; Calamita, Pace & Satolli, Reference Calamita, Pace and Satolli2012), in the Romagna–Marche area in the Central Apennines (Coward et al. Reference Coward, De Donatis, Mazzoli, Paltrinieri and Wezel1999; Butler et al. Reference Butler, Mazzoli, Corrado, De Donatis, Di Bucci, Gambini, Naso, Nicolai, Scrocca, Shiner, Zucconi and McClay2004; Butler, Tavarnelli & Grasso, Reference Butler, Tavarnelli and Grasso2006) and under the Montagna dei Fiori, the Sibilini Mountains and the Gran Sasso in the Central Apennines (Tozer et al. Reference Tozer, Butler, Corrado, Geologiche, Tre, San and Murialdo2002; Scisciani & Montefalcone, Reference Scisciani, Montefalcone, Mazzoli and Butler2006; Scisciani, Reference Scisciani2009; Calamita et al. Reference Calamita, Satolli, Scisciani, Esestime and Pace2011).
The evolution of ideas about the structural style of the Umbria–Marches domain of the Northern Apennines has been recently re-highlighted by Scisciani et al. (Reference Scisciani, Agostini, Calamita, Pace, Cilli, Giori and Paltrinieri2014) and is illustrated in Figure 5. The region has been classically interpreted as a thin-skinned thrust belt, with imbrication of sedimentary units detached along the Triassic evaporites, over an undeformed and buried crystalline basement (Bally et al. Reference Bally, Burbi, Cooper and Ghelardoni1986; Fig. 5a). Later interpretations showed that at least the upper part of the basement (i.e. the Verrucano phyllites) is also involved in the main thrust sheets, leading to a thick-skinned tectonic style (Calamita et al. Reference Calamita, Coltorti, Piccinini, Pierantoni, Pizzi, Ripepe, Scisciani and Turco2000; Fig. 5b). Mirabella, Barchi & Lupattelli (Reference Mirabella, Barchi and Lupattelli2008) alternatively proposed an imbricated array of gently dipping thrusts cutting through basement and sedimentary cover (Fig. 5c). A thick-skinned model involving inversion of Permo-Triassic basins with relatively steeply dipping faults was proposed by Tavarnelli et al. (Reference Tavarnelli, Butler, Decandia, Calamita, Grasso, Alvarez, Renda, Crescenti, D'Offizi, Merlini and Sacchi2004) (Fig. 5d). The most recent structural model to date, supported by recently released seismics, favours a deep-rooted basement-involved positive inversion of pre-existing extensional basins (Scisciani et al. Reference Scisciani, Agostini, Calamita, Pace, Cilli, Giori and Paltrinieri2014; Fig. 5e).
Recent research from the Northern Apennines has thus confirmed that thick-skinned tectonic interpretations apply to the external parts of the thrust belt. In the Marche area, simple thick-skinned inversion models honour available seismic, well and outcrop data, and clearly require far less orogenic shortening than do equivalent thin-skinned ones, e.g. 8.5 km of shortening (Butler et al. Reference Butler, Mazzoli, Corrado, De Donatis, Di Bucci, Gambini, Naso, Nicolai, Scrocca, Shiner, Zucconi and McClay2004) compared to 50 km for the equivalent transect using décollement-dominated, thin-skinned thrusting (Bally et al. Reference Bally, Burbi, Cooper and Ghelardoni1986). Recent seismotectonic studies also support active basement-involved shortening in the Northern Apennines and the southern Po Valley (Carannante et al. Reference Carannante, Argnani, Massa, D'Alema, Lovati, Moretti, Cattaneo and Augliera2015; Turrini et al. Reference Turrini, Angeloni, Lacombe, Ponton and Roure2015). In contrast, thin-skinned thrusting models are overall more suitable for the Southern Apennines. There, well data clearly demonstrate the presence of allochthonous thrust sheets transported for several tens of kilometres over mostly autochthonous 6–8 km thick Apulian platform carbonates. These buried carbonates are deformed by low-displacement, high-angle reverse faults involving the basement. Therefore, in the Southern Apennines, a switch from thin-skinned to thick-skinned thrusting seemingly occurred as the Apulian carbonates – and the underlying thick continental lithosphere – were deformed. The Central Apennines sector lies between these two main segments of the orogen and its structural style contains elements of both thin-skinned thrusting and thick-skinned inversion.
2.c. Zagros (Fars province)
The Zagros is the largest and most active collisional orogen related to Arabia/Eurasia convergence. It belongs to the Alpine–Himalayan orogenic system that resulted from the closure of the Neotethys during Cenozoic times. Collision is now thought to have initiated at ~35 Ma (e.g. Ballato et al. Reference Ballato, Uba, Landgraf, Strecker, Sudo, Stockli, Friedrich and Tabatabaei2011; Mouthereau, Lacombe & Verges, Reference Mouthereau, Lacombe and Verges2012) followed by intensification of deformation at ~20 Ma, possibly caused by the end of underthrusting of the thin Arabian margin crust under Eurasia, and so the onset of deformation of the interior of the Arabian plate (e.g. Morley et al. Reference Morley, Kongwung, Julapour, Abdolghafourian, Hajian, Waples, Warren, Otterdoom, Srisuriyon and Kazemi2009; Allen et al. Reference Allen, Saville, Blanc, Talebian and Nissen2013).
The Zagros folds formed in a thick (up to 12 km) pile of sedimentary rocks (Stocklin, Reference Stocklin1968; Colman-Sadd, Reference Colman-Sadd1978) including Palaeozoic, Mesozoic and Cenozoic strata of the former Arabian passive margin and platform. These strata were deposited in an extensional and passive margin setting during the late Palaeozoic and most of the Mesozoic periods followed by compression and development of the flexural foreland basin starting in Late Cretaceous times (e.g. Koop & Stoneley, Reference Koop and Stoneley1982; Beydoun, Clarke & Stoneley, Reference Beydoun, Clarke, Stoneley, Macqueen and Leckie1992; Homke et al. Reference Homke, Verges, Serra-Kiel, Bernaola, Sharp, Garces, Montero-Verdu, Karpuz and Goodarzi2009). Tertiary foreland deposits are represented by a ~3 km thick regressive siliciclastic sequence overlying the Oligo-Miocene carbonate platform. In the Fars, the base of the sedimentary cover rocks overlies the Infracambrian–Cambrian salt (Hormuz Formation), which has a maximum thickness of 1–2 km and which acts as an extremely efficient décollement (Colman-Sadd, Reference Colman-Sadd1978; Edgell, Reference Edgell, Alsop, Blundell and Davison1996).
In the Zagros, there is a lack of published sub-surface data from seismic refraction or reflection for depths > 10 km, so that it is difficult to have a clear image of the top of the basement (and of possible infra-Hormuz salt syn-rift Precambrian series; Husseini, Reference Husseini1988) and of the basement faults’ pattern. Two dominant tectonic trends, respectively N–S and NW–SE (to WNW–ESE), however, exist in the Arabian Shield (e.g. Stern, Reference Stern1985; Husseini, Reference Husseini1988). There is evidence for the continuation of such structures northwards in the former Zagros basin, and these structures were reactivated during the Cenozoic Zagros orogeny (e.g. Berberian, Reference Berberian1995; Talbot & Alavi, Reference Talbot, Alavi, Alsop, Blundell and Davison1996; Bahroudi & Talbot, Reference Bahroudi and Talbot2003; Ahmadhadi, Lacombe & Daniel, Reference Ahmadhadi, Lacombe, Daniel, Lacombe, Lavé, Vergés and Roure2007). The first group of basement faults are high-angle reverse faults resulting from the reactivation of basement normal faults formed in response to the Permo-Triassic rifting of Iran from Arabia. The other group of basement faults are N–S-trending faults, which developed during latest Proterozoic and early Cambrian times in the Arabian basement (Beydoun, Reference Beydoun1991). During Mesozoic times, the N–S uplifts and basins related to this group of basement faults were intermittently reactivated (Edgell, Reference Edgell, Rickard, Harrington and Williams1992; Sherkati & Letouzey, Reference Sherkati and Letouzey2004; Sepehr & Cosgrove, Reference Sepehr and Cosgrove2005). These faults are steep to vertical and are currently undergoing right-lateral strike-slip motion (Baker, Jackson & Priestley, Reference Baker, Jackson and Priestley1993; Berberian, Reference Berberian1995; Hessami et al. Reference Hessami, Koyi, Talbot, Tabasi and Shabanian2001; Sepehr & Cosgrove, Reference Sepehr and Cosgrove2005).
In the Fars province, the style of deformation is characterized by a first-order remarkable train of symmetrical, regularly spaced folds of similar amplitude with a wavelength typically of 15–20 km and length of 100 km and more. Most are underlined by the limestones of the Asmari–Jahrom formations. There is a noticeable lack of macroscopic reverse faults in the cover except where basement faults have been recognized. Seismicity in the Zagros is concentrated between the deformation front in the foreland and the regional 1250m contour (Jackson & McKenzie, Reference Jackson and McKenzie1984; Talebian & Jackson, Reference Talebian and Jackson2004; Nissen et al. Reference Nissen, Tatar, Jackson and Allen2011). Earthquakes of M ~5–6 occur in the crystalline basement up to depths of 20 km, but no deeper.
The Zagros Fold Belt (ZFB) has been viewed, for years, as a salt-based wedge (Davis & Engelder, Reference Davis and Engelder1985) in which the low topographic slope reflects the low friction décollement. In parallel, the reactivation of the basement normal faults formed in response to the rifting of Iran from Arabia as high-angle reverse faults at a depth between 10 and 20 km was also thought for a long time to be responsible for the major earthquakes along the Zagros belt (Jackson, Reference Jackson1980; Berberian & King, Reference Berberian and King1981; Jackson & McKenzie, Reference Jackson and McKenzie1984; Berberian, Reference Berberian1995). Mouthereau, Lacombe & Meyer (Reference Mouthereau, Lacombe and Meyer2006) showed that the short wavelength topography λ < 40 km coincides with the ‘whaleback’ folds, and that this local topography is superimposed onto a much larger scale signal (overall increase of elevation from 0 to 2500m across the Zagros folded belt) that results from distributed shortening at the crustal level (Fig. 6a, b, c). These authors concluded that shortening of the sedimentary cover does not create regional topography, which is rather related to thrusting within the Precambrian crystalline basement. This hypothesis is further supported by localized and long-lived deformation along several basement fault segments (e.g. Surmeh Fault and Mountain Front Fault) and the distribution of seismogenic activity within the upper brittle crust (Fig. 6e). Indeed, compressional or transpressional basement structures played a role in the deformation of the Zagros by localizing some topographic steps and major (often active) thrusts/strike-slip faults in the cover (Fig. 6c). Nissen et al. (Reference Nissen, Tatar, Jackson and Allen2011) re-appraised the inferred basement earthquakes and relocated most of them in the cover, suggesting that the basement deforms by aseismic creep beneath the ZFB. They also noticed that a few large earthquakes (Mw ~6.7) can rupture the basement.

Figure 6. Shortening mechanisms in the cover and the basement in the Zagros (modified after Mouthereau, Lacombe & Verges, Reference Mouthereau, Lacombe and Verges2012). (a) Topography (GTOPO30) and main structural features of the SE Zagros belt (Fars). (b) Cross-section of the Fars region. (c) Observed wavelength components of the topography showing the superimposition of regional topography (crustal deformation) and local fold topography (folding), modified after Mouthereau, Lacombe & Meyer (Reference Mouthereau, Lacombe and Meyer2006). (d) Principles of the crustal-scale orogenic wedge modelling of the regional topography, modified after Mouthereau, Lacombe & Meyer (Reference Mouthereau, Lacombe and Meyer2006). (e) Interpretative sketch showing the relationships between seismogenic deformation, main decoupling levels and topography in the Zagros orogenic wedge (modified after Mouthereau, Lacombe & Verges, Reference Mouthereau, Lacombe and Verges2012).
The nearly complete lack of sub-surface data, especially for depths > 10 km where most controversy exists over the depth to the basement and the geometry of tectonic structures, allows the drawing of very different fold and fault deep geometries that still honour surface and near-surface data. As recently re-emphasized by Allen et al. (Reference Allen, Saville, Blanc, Talebian and Nissen2013), nothing argues against both thick-skinned and thin-skinned deformation occurring coevally, so it is entirely feasible that the cover deforms in relation to multiple décollements while the basement deforms by high-angle thrusting. Figure 7 illustrates the evolution of ideas (but also the ongoing debate) on the crustal-scale structure of the ZFB of the Fars province. McQuarrie (Reference McQuarrie2004) proposed that the Fars segment of the FTB is completely detached on Hormuz salt (Fig. 7a) and that basement-involved thrusting occurs only in the hinterland of the orogen. This interpretation was later challenged by alternative views (Sherkati, Letouzey & Frizon de Lamotte, Reference Sherkati, Letouzey and Frizon de Lamotte2006; Fig. 7b) considering basement thrusting responsible for major steps of the basement–cover interface and accounting for significant step-like changes in the base level of synclines across the belt below a detached faulted and folded cover. Numerical models (Fig. 6d) also showed that alone the Hormuz weak basal salt layer cannot support the topography (Mouthereau, Lacombe & Meyer, Reference Mouthereau, Lacombe and Meyer2006). It has been therefore proposed that the origin of the topography of the Zagros would be related to deeper, crustal-scale deformation and not solely to thrust imbrication in the sedimentary cover. In this scenario, building the crustal-scale FTB requires sliding of the whole upper crust over the mostly aseismic lower crust (Mouthereau, Lacombe & Meyer, Reference Mouthereau, Lacombe and Meyer2006; Nissen et al. Reference Nissen, Tatar, Jackson and Allen2011). Indeed, the analysis of the topography shows that the Iranian plateau is currently expanding into the ZFB, as inferred by the plateau-like region in the northern ZFB and High Zagros of the Fars arc (Mouthereau, Lacombe & Verges, Reference Mouthereau, Lacombe and Verges2012; Allen et al. Reference Allen, Saville, Blanc, Talebian and Nissen2013). This has led to a wealth of recent structural cross-sections of the Zagros that therefore consider that long-term shortening is achieved by cover folding above the décollement in the Hormuz salt that is cut occasionally by active basement thrusts (Blanc et al. Reference Blanc, Allen, Inger and Hassani2003; Sherkati & Letouzey, Reference Sherkati and Letouzey2004; Molinaro et al. Reference Molinaro, Leturmy, Guezou, Frizon De Lamotte and Eshraghi2005; Sherkati, Letouzey & Frizon de Lamotte, Reference Sherkati, Letouzey and Frizon de Lamotte2006; Mouthereau et al. Reference Mouthereau, Tensi, Bellahsen, Lacombe, Deboisgrollier and Kargar2007; Emami et al. Reference Emami, Vergés, Nalpas, Gillespie, Sharp, Karpuz, Blanc, Goodarzi, Leturmy and Robin2010). Figure 7c illustrates the section proposed by Mouthereau et al. (Reference Mouthereau, Tensi, Bellahsen, Lacombe, Deboisgrollier and Kargar2007) and Mouthereau, Lacombe & Verges (Reference Mouthereau, Lacombe and Verges2012) where the southern Zagros results from the propagation and stacking of deep-reverse faults rooting at depth into the middle–lower crust. Note that on this section, there is nearly no thrust within the folded Fars cover, as suggested by recent mechanical models of buckling of the detached cover including second-order décollement levels (Yamato et al. Reference Yamato, Kaus, Mouthereau and Castelltort2011). The most recent section across the Fars (Allen et al. Reference Allen, Saville, Blanc, Talebian and Nissen2013; Fig. 7d) fulfils both available structural constraints and the seismological constraint that most (~75%) earthquakes of M ~5–6 are likely located within the cover and do not cut across the Hormuz Series salt (Nissen et al. Reference Nissen, Tatar, Jackson and Allen2011). Most thrusts appear to be blind and do not cut through anticlines exposed at the surface, but they do exist within the cover of the Zagros Simply Folded Belt. Basement faults have been included where there is a distinct change in structural relief on either side of an exposed structure, consistent with seismicity data that suggest that larger earthquakes in the Zagros occur in regions of greater structural relief, on faults that cut through the basement–cover boundary (Nissen et al. Reference Nissen, Tatar, Jackson and Allen2011). Allen et al. (Reference Allen, Saville, Blanc, Talebian and Nissen2013) acknowledged that it is a likely minimum interpretation of basement involvement.

Figure 7. Evolution of ideas about the structural style of the Fars province roughly along the section of Figure 6. (a) Interpretation of the Fars as completely detached on Hormuz salt (McQuarrie, Reference McQuarrie2004). (b) Interpretation considering basement thrusting responsible for major steps of the basement–cover interface and accounting for significant step-like changes in the base level of synclines below a detached faulted and folded cover (Sherkati, Letouzey & Frizon de Lamotte, Reference Sherkati, Letouzey and Frizon de Lamotte2006). (c) Interpretation with long-term shortening being achieved by cover folding (buckling) above the Hormuz salt that is cut occasionally by active basement thrusts (Mouthereau et al. Reference Mouthereau, Tensi, Bellahsen, Lacombe, Deboisgrollier and Kargar2007; Mouthereau, Lacombe & Verges, Reference Mouthereau, Lacombe and Verges2012); the Fars thus results from the propagation and stacking of deep-reverse faults rooting at depth into the middle–lower crust. (d) Interpretation by Allen et al. (Reference Allen, Saville, Blanc, Talebian and Nissen2013) with most cover thrusts as blind and not cutting through exposed anticlines, and a few basement faults associated with changes in structural relief and along which large earthquakes occur. MFF – Mountain Front Fault; HZF – High Zagros Fault; MZT – Main Zagros Thrust.
Interestingly, in their recent 2D thermomechanical modelling approach, Jammes & Huismans (Reference Jammes and Huismans2012) defined the Zagros-type as a broad orogenic domain involving propagation of thrusts towards the external part of the belt (they neglect the thin-skinned FTB as a first approximation) and showed that thick-skinned deformation in this orogen is well captured by an orogenic system with weak upper and lower crusts, which leads to deformation being accommodated on a few thick-skinned crustal-scale thrusts with moderate displacement and by distributed thickening of the crust.
3. Basement control on segmentation and along-strike variations of deformation style: Taiwan
In the Western Foothills of Taiwan, a wealth of structural and geophysical studies show that the basement is variably involved in shortening and is responsible for along-strike variations of structural style, wedge geometry and kinematics.
3.a. NW Taiwan
In NW Taiwan, the Taihsi Basin corresponds to a syn-rift Palaeogene basin trending ENE–WSW, i.e. parallel to the regional trend of the continental margin. It is bounded to the north by the Kuanyin High and to the south by the Peikang High (Fig. 8), two major basement promontories that were only slightly affected by extension. Deposition of a thick Oligocene–Miocene sequence occurred during a second episode of extension that took place after rifting and prior to collision. Both the pre-Miocene basement of the basin and the overlying Neogene sedimentary cover have been uplifted, making the Taihsi Basin an inverted basin (Huang, Chen & Chi, Reference Huang, Chen and Chi1993; Shen et al. Reference Shen, Huang, Tang and Hsu1996).

Figure 8. The Taiwan orogen. Insert: Main structural units of Taiwan. Isobaths in metres. PH, KH – Peikang/Kuanyin Highs; HR – Hsuehshan Range; S-PTFZ – Sanyi–Puli Transfer Fault Zone; CTFZ – Chishan Transfer Fault zone; J – 2010 Mw 6.2 Jiashian earthquake; N1 and N2 – 27 March ML 6.2 and 2 June ML 6.5 2013 Nantou earthquakes. (a1) Seismic evidence of reactivation of inherited basement faults from the Chinese continental margin in NW Taiwan (data after Yang et al. Reference Yang, Wu, Wickham, Ting, Wang and Chi1996, Reference Yang, Ting, Wu and Chi1997). (a2) Kinematic model of the NW Taiwan arcuate belt (modified after Lacombe et al. Reference Lacombe, Mouthereau, Angelier, Chu and Lee2003). The curvature of the basin-controlled salient is accommodated to the south by the Pakua Transfer Fault Zone (PTFZ) and to the north by a diffuse oblique ramp (the Kuanyin Transfer Fault Zone, KTFZ) where high-angle wrench-thrust faults inherited from the inversion of normal faults guided the emplacement of thin-skinned low-angle thrusts. WF – Western Foothills; HR – Hsuehshan Range; Central R – Central Range; CR – Coastal Range; a – local extension accommodating curvature along the limbs of the arc; b – normal fault of the margin; c – high-angle wrench-thrust fault; d – vertical axis rotations. (a3) Structural sketch map of the Taiwan thrust belt, showing locations of the different types of thrust-belt fronts in western Taiwan and their relationship with the shape of the pre-Miocene basement. The along-strike variation of structural style is correlated with along-strike variations in the main stress regimes and demonstrates the evolution from prominent strike-slip regimes to purely compressional regimes especially in areas where basin inversion occurs. (b) Seismological evidence for crustal shortening in western Central Taiwan: (b1) Crustal cross-section modified after Brown et al. (Reference Brown, Alvarez-Marron, Schimmel, Wu and Camanni2012) and Camanni et al. (Reference Camanni, Brown, Alvarez-Marron, Wu and Chen2014). (b2) Crustal cross-section modified after Chuang et al. (Reference Chuang, Johnson, Wu, Ching and Kuo2013). The red beach balls denote the Nantou main shocks, and the black beach balls denote the Chi-Chi aftershocks and other ML > 5.5 events. (c) Seismological evidence of crustal shortening and a deep décollement in SW Taiwan. (c1) Distribution with depth of mean slip and aftershocks following the 2010 Jiashian earthquake (blue circles) together with background seismicity (grey circles). White star denotes the location of the 2010 Jiashian earthquake main shock (modified after Ching et al. Reference Ching, Johnson, Rau, Chuang, Kuo and Leu2011; Rau et al. Reference Rau, Lee, Ching, Lee, Byrne and Chen2012). (c2) Conceptual tectonic model for southern Taiwan inferred from the 2010 Jiashian earthquake. Red star denotes the location of the main shock. CCU – Chaochou fault; CKU – Chukou fault; CTFZ – Chishan Transfer Fault Zone (modified after Ching et al. Reference Ching, Johnson, Rau, Chuang, Kuo and Leu2011).
Offshore, most features strike N070°, at a high angle to the structural grain of the belt: they correspond either to Palaeogene to Miocene normal faults which probably extend down to the Mesozoic basement, or to high-angle thrusts which originated from the reactivation of the previous normal faults (Yang et al. Reference Yang, Wu, Ting, Wang and Chi1994, Reference Yang, Ting, Wu and Chi1997). The N070° faults extend onshore as S-dipping high-angle thrusts (Fig. 8a1). These high-angle thrusts result from the compressional reactivation of pre-existing normal faults of the margin (Huang, Chen & Chi, Reference Huang, Chen and Chi1993; Yang et al. Reference Yang, Wu, Wickham, Ting, Wang and Chi1996). Oblique en échelon folds along these high-angle thrusts as well as along-strike variations in magnitude and sense of offset additionally indicate a significant amount of right-lateral wrench movement (Huang, Chen & Chi, Reference Huang, Chen and Chi1993; Lee et al. Reference Lee, Chang, Mao and Tseng1993; Shen et al. Reference Shen, Huang, Tang and Hsu1996).
The pattern of folds and W-verging imbricated thin-skinned thrust sheets of the arcuate FTB of NW Taiwan formed in relation with various décollement levels recognized within both the pre-collisional Palaeogene to Miocene deposits and the synorogenic Plio-Pleistocene formations.
The obliquity of the N120° regional transport direction with respect to the orientation of the inherited extensional structures of the margin localized areas dominated by frontal contraction and those dominated by wrench deformation (Fig. 8a3). Frontal contraction occurred against the Peikang High, which acted as a buttress for the propagating thrusts (Lu et al. Reference Lu, Jeng, Chang and Jian1998; Mouthereau et al. Reference Mouthereau, Lacombe, Deffontaines, Angelier, Chu and Lee1999). In contrast, the northern edge of the Peikang High as well as the southern edge of the Kuanyin High, which are associated with significant basement offset and changes in thickness of sedimentary formations, have localized ‘conjugate’ lateral/oblique ramps that guided thrust emplacement and accommodated curvature of the NW Taiwan salient. The structural and kinematic model of Figure 8a2 involves the oblique reactivation of the normal faults inherited from the Palaeogene–Neogene extensional history of the margin and their interaction with the thrusts of the Plio-Pleistocene Taiwan orogenic wedge. N070°-trending normal faults were first obliquely reactivated as high-angle thrusts with a right-lateral wrench component in response to the far-field transmission of orogenic stresses during the early stage of the Plio-Pleistocene arc–continent collision. Thrust sheets related to the growing orogenic wedge then initiated in relation to shallow décollements in the cover and propagated in a nearly N110–120° transport direction, i.e. obliquely towards the reactivated normal faults. In a second stage, the inverted normal faults were used as lateral/oblique ramps for the low-angle thrusts, which continued to propagate and consequently turn their strike northwards into a direction parallel to the N070° strike of the high-angle thrusts (Fig. 8a1, a2; Lacombe et al. Reference Lacombe, Mouthereau, Angelier, Chu and Lee2003).
The NW Taiwan arc is therefore mainly a basin-controlled salient where deformation was accommodated by both thin-skinned shallow thrusts and basement faults, and therefore where both the cover and the basement are involved in shortening. The arc clearly developed in the portion of the foreland basin that was initially thicker (the Taihsi Basin) (Lacombe et al. Reference Lacombe, Mouthereau, Angelier, Chu and Lee2003; Fig. 8a2). This demonstrates also the ‘passive’ control on the development of the NW Taiwan salient exerted by the distribution of the basement highs and the related along-strike variations in the pre-orogenic basin thickness.
3.b. North Central Taiwan
In north Central Taiwan, thin-skinned deformation predominates in the outermost (frontal) unit, whereas basement-involved thrusting likely played a major role more to the east. To this respect, and although challenging interpretations exist (Yue, Suppe & Hung, Reference Yue, Suppe and Hung2005), the Chelungpu–Sani fault has been interpreted by Mouthereau & Lacombe (Reference Mouthereau and Lacombe2006) as an inverted normal fault.
A number of geophysical observations suggest that deeper levels of the crust are involved in the mountain-building process east of the frontal units. Seismicity in Taiwan extends well below the proposed décollement depth (Carena, Suppe & Kao, Reference Carena, Suppe and Kao2002) and thick crust underlying high topography supports active deformation of deeper crust, hence basement-involved, thick-skinned models (e.g. Lacombe & Mouthereau, Reference Lacombe and Mouthereau2002; Mouthereau & Lacombe, Reference Mouthereau and Lacombe2006).
The 27 March ML 6.2 and 2 June ML 6.5 2013 Nantou earthquakes nucleated below the shallow décollement within the deep 21 September 1999 Chi-Chi aftershock clusters. Chuang et al. (Reference Chuang, Johnson, Wu, Ching and Kuo2013) proposed that the two earthquakes occurred on essentially the same fault plane, the so-called Nantou fault, and that the majority of the combined slip was released between depths of 5 and 20 km (Fig. 8b2). These authors proposed that the Nantou earthquakes as well as the Chi-Chi aftershocks reflect a deep extension of the shallow ramp fault structure into the middle crust.
Contrasting interpretations of the Nantou fault have been proposed: (1) it could be a deep extension of the Shuilikeng fault (Brown et al. Reference Brown, Alvarez-Marron, Schimmel, Wu and Camanni2012; Camanni et al. Reference Camanni, Brown, Alvarez-Marron, Wu and Chen2014); in this interpretation, the Shuilikeng fault is an active deep-seated main structure of the Taiwan orogen (Fig. 8b1). Westwards, a subhorizontal cluster of seismicity can be interpreted as the décollement to the imbricate stack mapped there, linking it to the thick-skinned deformation east of the Shuilikeng fault margin of the Hsuehshan Basin in Eocene time (Camanni et al. Reference Camanni, Brown, Alvarez-Marron, Wu and Chen2014); (2) The Nantou fault may link to the shallow décollement that partially ruptured during the Chi-Chi earthquake based on the Chi-Chi aftershock distributions. The bottom of the Nantou fault might link to the deep Chi-Chi aftershock cluster; (3) if the Nantou fault has no direct link with any of the shallow ramp faults overlying the décollement, the Nantou fault can well be a newly developed sub-surface structure (Fig. 8b2) (Chuang et al. Reference Chuang, Johnson, Wu, Ching and Kuo2013).
Seismicity deeper than the proposed Taiwan décollement thus exists in north Central Taiwan, including the deep Chi-Chi aftershock cluster at depths between 20 and 30 km. It is therefore indisputable that a significant part of the shortening across Taiwan is accommodated by slip on deep fault systems. The existence of the deep Nantou fault as well as a lack of a clear seismic signature of the décollement beneath the Central Range (e.g. Wu, Chang & Wu, Reference Wu, Chang, Wu, Malpas, Fletcher, Ali and Aitchison2004; Gourley et al. Reference Gourley, Byrne, Chan, Wu and Rau2007; Wu et al. Reference Wu, Chang, Zhao, Teng and Nakamura2008; Brown et al. Reference Brown, Alvarez-Marron, Schimmel, Wu and Camanni2012) clearly argue against a structural architecture across Taiwan being fully accounted for by thin-skinned tectonics above a main décollement (e.g. Suppe, Reference Suppe1980; Carena, Suppe & Kao, Reference Carena, Suppe and Kao2002; Yue, Suppe & Hung, Reference Yue, Suppe and Hung2005), and support that deep structures play an important role in accommodating regional compressional deformation across the active collisional belt (Brown et al. Reference Brown, Alvarez-Marron, Schimmel, Wu and Camanni2012; Chuang et al. Reference Chuang, Johnson, Wu, Ching and Kuo2013; Camanni et al. Reference Camanni, Brown, Alvarez-Marron, Wu and Chen2014).
3.c. South Central Taiwan
The Chishan Transfer Fault Zone (CTFZ) has been interpreted as a major transverse domain that marks the transition from a region of basement-involved tectonics to the north (Deffontaines et al. Reference Deffontaines, Lacombe, Angelier, Chu, Mouthereau, Lee, Deramond, Lee, Yu and Liew1997; Mouthereau et al. Reference Mouthereau, Deffontaines, Lacombe, Angelier, Byrne and Liu2002; Mouthereau & Lacombe, Reference Mouthereau and Lacombe2006) to the area of tectonic escape with a thin-skinned style (Lacombe et al. Reference Lacombe, Mouthereau, Angelier and Deffontaines2001). In the field the CTFZ strikes N130°E and crops out as a more than 20 km long and 1 km wide elongated bulge, containing several closely spaced NW–SE shear zones associated with a left-lateral sense of transpressive shear (Deffontaines et al. Reference Deffontaines, Lacombe, Angelier, Chu, Mouthereau, Lee, Deramond, Lee, Yu and Liew1997; Lacombe et al. Reference Lacombe, Mouthereau, Deffontaines, Angelier, Chu and Lee1999). In map view, the CTFZ acts as a lateral ramp connecting stepping thrusts, the Chaochou fault and the Chukou fault.
In south Central Taiwan, basin inversion and basement-involved shortening predominate in the outermost units (Mouthereau et al. Reference Mouthereau, Deffontaines, Lacombe, Angelier, Byrne and Liu2002; Mouthereau & Lacombe, Reference Mouthereau and Lacombe2006). As in NW Taiwan, the structure of folds and thrusts beneath the Coastal Plain results mainly from inversion of inherited normal faults. The development of fold-and-thrust structures in this domain is strongly influenced by the presence of an inherited pre-orogenic trough, namely, the Tainan basin. In a somewhat analogue way to the Taihsi Basin northwards, the inversion of the intra-marginal Tainan basin leads to the development of a dominant thick-skinned deformation style.
The 2010 Mw 6.2 Jiashian earthquake (Ching et al. Reference Ching, Johnson, Rau, Chuang, Kuo and Leu2011; Rau et al. Reference Rau, Lee, Ching, Lee, Byrne and Chen2012; Wen et al. Reference Wen, Hsu, Chang and Chen2012; Fig. 8c2) provided direct seismological evidence of activity of the CTFZ. The position and strike of the inferred fault plane suggests that the earthquake occurred on the southeastern extension of the CTFZ. The mismatch between the inferred fault top edge and the trace of the CTFZ might be explained either by along-strike bending of the surface trace of the southeastern extension of the CTFZ towards the SE or by a change in dip of the fault plane at depth such that the upper section of this fault has a larger dip angle than the deeper part that ruptured in the earthquake (Ching et al. Reference Ching, Johnson, Rau, Chuang, Kuo and Leu2011).
For the 2010 Jiashian earthquake, the focal depth is about 23 km and the inferred slip is confined to the 10–25 km depth range (Ching et al. Reference Ching, Johnson, Rau, Chuang, Kuo and Leu2011; Rau et al. Reference Rau, Lee, Ching, Lee, Byrne and Chen2012; Fig. 8c1). Together with the Nantou earthquakes in north Central Taiwan, this implies that the depth of seismicity and the depth of the likely active basal décollement are much deeper than the previously proposed ~10 km depth inferred by the distribution of small earthquakes (Carena, Suppe & Kao, Reference Carena, Suppe and Kao2002). Regardless of whether this earthquake reflects motion on a reactivated passive margin structure (Huang et al. Reference Huang, Dreger, Bürgmann, Yoo and Hashimoto2013) or a lateral ramp of a basal décollement (Ching et al. Reference Ching, Johnson, Rau, Chuang, Kuo and Leu2011), or even whether the earthquake may involve two rupture planes (Lin et al. Reference Lin, Delouis, Hu, Nocquet and Mozziconacci2015), it strongly supports the idea that the whole Chinese crust is involved in shortening.
To conclude on Taiwan, there is a wealth of evidence provided by structural geology, magnetotelluric sounding, earthquake hypocentre distribution and seismic tomography that that rocks below the basal thrust proposed by Carena, Suppe & Kao (Reference Carena, Suppe and Kao2002), including the pre-Tertiary crystalline basement, are involved in collisional shortening. Many works have documented steeply dipping faults that penetrate down into the middle and perhaps even the lower crust, many (if not all) of them being inherited from the extensional history pre-dating the Taiwan orogeny. Pre-existing structures of a continental margin therefore play a very important role in many aspects of the evolution of an orogen during mountain building. The young Chinese continental margin was affected by a rapid succession of thermal and extensional events since Palaeogene times. These events caused a pre-orogenic structural segmentation of the margin, outlined for instance by marginal basins separated by basement highs. This segmentation pattern was presumably responsible for significant rheological heterogeneities in the crust/lithosphere along the Chinese continental margin prior to the collisional event. Subsequent shortening has been largely controlled by these pre-existing heterogeneities and the positive inversion of the former extensional basins (e.g. Wu, Rau & Salzberg, Reference Wu, Rau and Salzberg1997; Mouthereau et al. Reference Mouthereau, Deffontaines, Lacombe, Angelier, Byrne and Liu2002; Mouthereau & Petit, Reference Mouthereau and Petit2003; Mouthereau & Lacombe, Reference Mouthereau and Lacombe2006; Hwang et al. Reference Hwang, Hsiao, Shih, Yang, Chen, Forsberg and Olesen2007; Byrne et al. Reference Byrne, Chan, Rau, Lu, Lee, Wang, Brown and Ryan2011; Brown et al. Reference Brown, Alvarez-Marron, Schimmel, Wu and Camanni2012; Camanni et al. Reference Camanni, Brown, Alvarez-Marron, Wu and Chen2014, Reference Camanni, Alvarez-Marron, Brown, Wu and Hsieh2015). Along-strike structural changes in the Taiwan belt are associated with variations in the recent kinematics of the deformation in the frontal areas, which reflect local effects such as frontal contraction and lateral movement in response to indentation by the basement buttresses (Fig. 8a3).
4. Along-strike variations of deformation style and shortening: western Alps and Pyrenees
4.a. The External Crystalline Massifs of the western Alps
The Alps result from the closure of the Ligurian oceanic domain, the subduction of which started during Late Cretaceous times and lasted until late Paleocene to early Eocene times. Oceanic subduction was followed by continental subduction during middle to late Eocene times (e.g. Dora Maira; Duchene et al. Reference Duchene, Blichert-Toft, Luais, Telouk, Lardeaux and Albarede1997; Rubatto & Hermann, Reference Rubatto and Hermann2001; Oberhänsli et al. Reference Oberhänsli, Bousquet, Engi, Goffé, Gosso, Handy, Höck, Koller, Lardeaux, Polino, Rossi, Schuster, Schwartz and Spalla2004), then collisional crustal thickening that started during Oligocene times (e.g. for the western Alps; Corsini, Ruffet & Caby, Reference Corsini, Ruffet and Caby2004; Rolland et al. Reference Rolland, Rossi, Cox, Corsini, Mancktelow, Pennacchioni, Fornari, Boullier, Wibberley, Kurz, Imber, Holdsworth and Collettini2008; Simon-Labric et al. Reference Simon-Labric, Rolland, Dumont, Heymes, Authemayou, Corsini and Fornari2009; Sanchez et al. Reference Sanchez, Rolland, Schneider, Corsini, Oliot, Goncalves, Verati, Lardeaux and Marquer2011; Bellanger et al. Reference Bellanger, Bellahsen, Jolivet, Baudin, Augier and Boutoux2014), especially with shortening of the external Alps, in the External Crystalline Massifs (ECMs). The Alpine ECMs are composed of the Argentera–Mercantour in the southwestern Alps, the Oisans, Grandes Rousses, Belledonne, Mont Blanc and Aiguilles Rouges massifs in the western Alps, and the Aar and Gothard massifs in the central Alps (Fig. 9a).

Figure 9. (a) Structural sketch of the western Alps. AR – Aiguilles Rouges; MB – Mont Blanc; GR – Grandes Rousses; B – Belledonne. (b) Structural sketch of the Pyrenees. NPZ/SPZ – North/South Pyrenean Zone. (c) Structural sections across the external zones at the latitude of the northern Mont-Blanc ECM (c1), of the southern Mont-Blanc ECM (c2) and of the Oisans ECM (c3) (modified after Bellahsen et al. Reference Bellahsen, Mouthereau, Boutoux, Bellanger, Lacombe, Jolivet and Rolland2014). Note the along-strike change in accommodation of basement shortening from c1 to c3. (d) Structural sections across the Axial Zone of the Pyrenees and the southern Pyrenean FTB. (d1) Modified after Jolivet et al. (Reference Jolivet, Labaume, Monie, Brunel, Arnaud and Campani2007). (d2) Modified after Mouthereau et al. (Reference Mouthereau, Filleaudeau, Vacherat, Pik, Lacombe, Fellin, Castelltort, Christophoul and Masini2014).
At the latitude of Oisans, the total amount of shortening across the external zone is about 28 km (Fig. 9c3), including 11.5 km accommodated within the ECMs (Bellahsen et al. Reference Bellahsen, Jolivet, Lacombe, Bellanger, Boutoux, Garcia, Mouthereau, Le Pourhiet and Gumiaux2012) where the Mesozoic metasedimentary cover is not significantly detached from the Variscan basement. Disharmonic folding occurs in the cover, especially above W-verging basement shear zones (see Section 7.b). Shortening of these basement/cover units occurred under greenschist-facies conditions (3–4 kb and 300–350°C: Jullien & Goffé, Reference Jullien and Goffé1993; Crouzet, Menard & Rochette, Reference Crouzet, Menard and Rochette2001; Simon-Labric et al. Reference Simon-Labric, Rolland, Dumont, Heymes, Authemayou, Corsini and Fornari2009); their exhumation started at least at 27 Ma from zircon fission track (ZFT) ages (van der Beek et al. Reference van der Beek, Valla, Herman, Braun, Persano, Dobson and Labrin2010) for the Meije massif (NE Oisans) and at least at 24 Ma from thermopalaeomagnetism (Crouzet, Menard & Rochette, Reference Crouzet, Menard and Rochette2001) for the Grandes Rousses massif; apatite fission track dating (AFT) showed that these massifs cooled through the 110°C isotherm at about 3–8 Ma (Vernon et al. Reference Vernon, van der Beek, Sinclair and Rahn2008). In the Mont Blanc massif (Fig. 9b), basement deformation by anastomosed steep transpressional shear zones occurred from 22 to 15 Ma (Rolland et al. Reference Rolland, Rossi, Cox, Corsini, Mancktelow, Pennacchioni, Fornari, Boullier, Wibberley, Kurz, Imber, Holdsworth and Collettini2008) at around 400°C and 5 kb (Rolland et al. Reference Rolland, Cox, Boullier, Pennacchioni and Mancktelow2003). Recently, ages of 29 Ma were obtained in central Mont Blanc shear zones (Cenki-Tok et al. Reference Cenki-Tok, Darling, Rolland, Dhuime and Storey2013), suggesting that the onset of the shortening could be older. For the Aiguilles Rouges massif, the structural interpretation of Burkhard & Sommaruga (Reference Burkhard, Sommaruga, Mascle, Puigdefabregas, Luterbacher and Fernandez1998) suggests that the upper Aiguilles Rouges basement thrusts were active during deposition of the so-called Upper Freshwater Molasse, i.e. since 16 Ma. In both the Mont Blanc and the Aiguilles Rouges massifs, most ZFT ages are about 8–15 Ma; AFT ages are about 3–8 Ma (Vernon et al. Reference Vernon, van der Beek, Sinclair and Rahn2008). In the Aar massif, the main shearing event occurred between 17 and 21 Ma at 400–450°C and 6 kb (Challandes, Marquer & Villa, Reference Challandes, Marquer and Villa2008) or 20–22 Ma (Rolland, Cox & Corsini, Reference Rolland, Cox and Corsini2009) with W-verging kinematics and was followed by reverse to dextral shear zones at 12–14 Ma (Rolland, Cox & Corsini, Reference Rolland, Cox and Corsini2009). Late brittle deformations occurred from 9–5 Ma (Kralik et al. Reference Kralik, Clauer, Holnsteiner, Huemer and Kappel1992) and probably until 3 Ma (Hofmann et al. Reference Hofmann, Helfer, Diamond, Villa, Frei and Eikenberg2004).
In the frontal thin-skinned FTBs (Vercors, Chartreuse, Bauges, Bornes, from south to north), the age of shortening is about middle Miocene (e.g. Burkhard & Sommaruga, Reference Burkhard, Sommaruga, Mascle, Puigdefabregas, Luterbacher and Fernandez1998). The Jura is slightly younger and developed from Serravallian until early Pliocene times (see Section 5). In these massifs, the thrusts root into a décollement located within the upper Triassic or Liassic series (Deville et al. Reference Deville, Mascle, Lamiraux and Le Bras1994; Philippe, Deville & Mascle, Reference Philippe, Deville, Mascle, Mascle, Puigdefabregas, Luterbacher and Fernandez1998).
Localization and style of basement-involved deformation vary along the strike of the western Alpine arc (Fig. 9a). In the Oisans (Fig. 9c, section c3), basement was shortened in a distributed way during Oligocene times (Simon-Labric et al. Reference Simon-Labric, Rolland, Dumont, Heymes, Authemayou, Corsini and Fornari2009; Bellahsen et al. Reference Bellahsen, Mouthereau, Boutoux, Bellanger, Lacombe, Jolivet and Rolland2014; Bellanger et al. Reference Bellanger, Augier, Bellahsen, Jolivet, Monie, Baudin and Beyssac2015) before deformation localized on the frontal ramp that activated the Vercors shallow décollement (Deville et al. Reference Deville, Mascle, Lamiraux and Le Bras1994). Deformation there was thus first characterized by accretion and thrust stacking below the wedge (distributed underplating) without wedge widening, and later by frontal accretion, hence orogenic wedge widening during Miocene times. In contrast, along the Mont Blanc–Aiguilles Rouges section, basement shortened by underplating below the internal units during Oligo-Miocene times (Leloup et al. Reference Leloup, Arnaud, Sobel and Lacassin2005; Rolland et al. Reference Rolland, Rossi, Cox, Corsini, Mancktelow, Pennacchioni, Fornari, Boullier, Wibberley, Kurz, Imber, Holdsworth and Collettini2008). During late Miocene – early Pliocene times, basement units were still underplated (lower Aiguilles Rouges) while a very wide cover domain was accreted in the frontal parts (e.g. Jura and Molasse Basin) with the activation of large basement thrusts (Burkhard & Sommaruga, Reference Burkhard, Sommaruga, Mascle, Puigdefabregas, Luterbacher and Fernandez1998).
Moreover, both amounts of shortening and shortening across the entire external zone increase from the Oisans section to the Mont Blanc section (Fig. 9c). The increase in the amount of shortening is most likely due to a wider inherited Mesozoic basin in the north (Ultra-Helvetic/Valaisan; Bellahsen et al. Reference Bellahsen, Mouthereau, Boutoux, Bellanger, Lacombe, Jolivet and Rolland2014). However, the increase in the shortening values probably has a rheological explanation. Along the Mont Blanc section, basement shortening remains localized, leading to stacking of basement slices (Fig. 9c, section c1), while it is distributed far towards the foreland along the Oisans section (Fig. 9c, section c3); this can be related to the rheology of the crust during collision, the more buried and thermally weakened crust at the latitude of Mont Blanc (400°C, 5 kb) being more prone to localized shortening at the orogen scale (see Section 8).
4.b. Pyrenees
The Pyrenean mountain belt results from the inversion of a rifted and hyper-extended Mesozoic crustal domain located in the present-day North Pyrenean Zone (NPZ) (Roure et al. Reference Roure, Choukroune, Berastegui, Munoz, Villien, Matheron, Bareyt, Seguret, Camara and Deramond1989; Beaumont et al. Reference Beaumont, Munoz, Hamilton and Fullsack2000; Verges, Fernandez & Martinez, Reference Verges, Fernandez and Martinez2002; Jammes et al. Reference Jammes, Manatschal, Lavier and Masini2009; Lagabrielle, Labaume & de Saint Blanquat, Reference Lagabrielle, Labaume and de Saint Blanquat2010). The convergence started during Late Cretaceous times and lasted until Oligocene times (e.g. Verges, Fernandez & Martinez, Reference Verges, Fernandez and Martinez2002). During the collision, N-verging crustal thrusts deformed the NPZ, while S-verging thrusts affected the Axial Zone and the South Pyrenean Zone (SPZ; Fig. 9b, d). The Axial Zone is composed of a few basement units, namely the Lakora, Gavarnie, Bielsa–Millares and Guarga in the western part and Gavarnie/Nogueres, Orri and Rialp in the east (Fig. 9b). These units form the so-called Pyrenean axial antiformal stack (Fig. 9d).
In the western part of the Axial Zone, the Lakora thrust was active above the future Gavarnie unit during Lutetian times (Teixell, Reference Teixell1996) during the deposition of the Hecho Group turbidite series (Labaume, Seguret & Seyve, Reference Labaume, Seguret and Seyve1985) in the Jaca basin (Fig. 9b, d). During late Eocene – early Oligocene times, the Gavarnie thrust affected the basement, triggering the southward migration of the foreland basin. Finally, during Oligocene times, the Guarga unit was emplaced in the basin. Further east, in the Lakora/Gavarnie unit, reverse shear zones were dated at about 47–48 Ma (Wayne & McCaig, Reference Wibberley, Bruhn and Burlini1998). In the footwall of the Gavarnie thrust, the Bielsa–Millares unit was part of a larger basement unit, namely the Guarga unit (Teixell, Reference Teixell1996), the emplacement of which tilted the Lutetian Hecho turbidites in the Jaca basin (Labaume, Seguret & Seyve, Reference Labaume, Seguret and Seyve1985; Teixell, Reference Teixell1996) probably during late Oligocene times (Jolivet et al. Reference Jolivet, Labaume, Monie, Brunel, Arnaud and Campani2007). In the central Axial Zone, cooling history is well documented: in the Gavarnie unit, ZFT ages are about 50 Ma (Sinclair et al. Reference Sinclair, Gibson, Naylor and Morris2005), AFT ages about 26 to 45 Ma (Fitzgerald et al. Reference Fitzgerald, Muñoz, Coney and Baldwin1999; Sinclair et al. Reference Sinclair, Gibson, Naylor and Morris2005) and UTh/He on apatites between 17 and 37 Ma (Gibson et al. Reference Gibson, Sinclair, Lynn and Stuart2007). In the Orri unit, below the Gavarnie unit, AFT ages range between 17 and 35 Ma (Morris, Sinclair & Yelland, Reference Morris, Sinclair and Yelland1998; Fitzgerald et al. Reference Fitzgerald, Muñoz, Coney and Baldwin1999; Sinclair et al. Reference Sinclair, Gibson, Naylor and Morris2005; Gibson et al. Reference Gibson, Sinclair, Lynn and Stuart2007), and U–Th/He ages on apatites range between 10 and 35 Ma (Gibson et al. Reference Gibson, Sinclair, Lynn and Stuart2007). These ages show an extremely rapid exhumation during early Oligocene times, following and followed by a slower exhumation during Eocene and late Oligocene times (Fitzgerald et al. Reference Fitzgerald, Muñoz, Coney and Baldwin1999).
Because the Pyrenees result from the inversion of a former rifted domain, fault inversion is a common feature of their structural style in the Axial Zone (Saura & Teixell, Reference Saura and Teixell2006; Lagabrielle, Labaume & de Saint Blanquat, Reference Lagabrielle, Labaume and de Saint Blanquat2010), the NPZ (Biteau et al. Reference Biteau, Le Marrec, Le Vot and Masset2006; Lagabrielle, Labaume & de Saint Blanquat, Reference Lagabrielle, Labaume and de Saint Blanquat2010) or, in the eastern extension of the Pyrenees, the Provence domain (Roure & Colletta, Reference Roure, Colletta, Ziegler and Horvath1996; Lacombe & Mouthereau, Reference Lacombe and Mouthereau2002; Espurt et al. Reference Espurt, Hippolyte, Saillard and Bellier2012; Bestani et al. Reference Bestani, Espurt, Lamarche, Floquet, Philip, Bellier, Hollender, Seranne, Lamarche and Agosta2015). In contrast, in the southern Pyrenees, inherited faults were not widely reactivated but were truncated by thrusts (Fernandez et al. Reference Fernandez, Munoz, Arbues and Falivene2012) or simply localized frontal thrusts, as for the Boixols unit, by causing important sedimentary thickness variations on both sides of the thrust zones (Garcia-Senz, Muñoz & McClay, Reference Garcia-Senz, Muñoz and McClay2000).
P–T conditions of deformation in the Pyrenees were very different from the western Alps, especially in term of burial, which remained much shallower. However, the striking similarity in term of basement shortening style between sections c1 and d2, and sections c2 and d1 in Figure 9 suggests that along-strike variations in the structural style may also be controlled by differences in crustal thermicity, with temperatures lower to the west than to the east. This is consistent with the maximum temperature recorded by the Raman Spectroscopy on Carbonaceous Material technique in the NPZ and related to the Cretaceous extension and mantle denudation, higher to the east than to the west (Clerc & Lagabrielle, Reference Clerc and Lagabrielle2014). In this perspective, the crust was hotter and weaker in the east, where, as a consequence, shortening was more localized than in the west, although the total shortening is similar.
Low-temperature thermochronology supports that the high geothermal gradient lasted 30–50 Ma after extension, hence during convergence in the NPZ (Vacherat et al. Reference Vacherat, Mouthereau, Pik, Bernet, Gautheron, Masini, Le Pourhiet, Tibaric and Lahfid2014) and probably also in the Axial Zone, which likely favoured basement-involved shortening. Note that the formation of basement antiformal stacks (similar to the formation of the Nogueres, Orri and Rialp thrust sheets in the eastern Pyrenees and to the Gavarnie and Guarga thrust sheets in the western Pyrenees; Fig. 9d1, d2) is well reproduced by 2D thermomechanical modelling of an orogenic system in which the development of thrusts rooted in the buttress creates a stacking of basement rocks and the lower crust is thick (> 15 km) (Jammes & Huismans, Reference Jammes and Huismans2012).
5. Evolving tectonic style from thin-skinned to thick-skinned through time: the Jura
The Jura is an arcuate foreland FTB formed by folding and faulting of the Mesozoic cover detached from the Palaeozoic basement along Triassic evaporites as a result of crustal stacking in the Alpine ECMs (e.g. Philippe et al. Reference Philippe, Colletta, Deville, Mascle, Ziegler and Horvath1996; Burkhard & Sommaruga, Reference Burkhard, Sommaruga, Mascle, Puigdefabregas, Luterbacher and Fernandez1998; Bellahsen et al. Reference Bellahsen, Mouthereau, Boutoux, Bellanger, Lacombe, Jolivet and Rolland2014; Fig. 10a, b).

Figure 10. (a) Simplified structural map of the NW Alpine foreland. V – Vosges; BF – Black Forest; AR – Aiguilles Rouges; MB – Mont Blanc; B – Belledonne; O – Oisans. (b) Crustal-scale cross sections from the Bresse graben to the Belledonne massif emphasizing inversion of inherited Palaeozoic basin beneath the thin-skinned Jura FTB, basement thrusting and likely occurrence of a deep crustal décollement rooting at the brittle–ductile transition. Pz, Mz and Cz – Palaeozoic, Mesozoic and Cenozoic. (c) Two-stage tectonic evolution the northwestern Jura front. Late Miocene to early Pliocene thin-skinned deformation dominated in the Besançon Zone (BZ), followed by thick-skinned deformation involving both Mesozoic cover and Palaeozoic basement in the Avant-Monts Zone. This thick-skinned deformation is associated with compressional to transpressional reactivation of pre-existing normal faults of Palaeogene to Palaeozoic age of the intracontinental Rhine–Bresse Transfer Zone (RBTZ) and the underlying late Palaeozoic Burgundy Trough (modified after Madritsch, Schmid & Fabbri, Reference Madritsch, Schmid and Fabbri2008). BG – Bresse graben; LSH – La Serre Horst; URG – Upper Rhinegraben. (d) Distribution of inherited Permo-Carboniferous basins beneath the Jura and the Alpine foreland (modified after Truffert et al. Reference Truffert, Burg, Cazes, Byer, Damotte, Rey, Roure, Heitzmann and Polino1990). 1 – Palaeozoic basement. 2 – Permo-Carboniferous basins. 3 – Meso-Cenozoic cover of the Alpine foreland. 4 – Jura FTB. 5 – Faults bounding Permo-Carboniferous basins (documented = solid lines/inferred = dashed lines). (e) Seismic evidence for the compressional reactivation of an E–W-trending, N-dipping high-angle late Palaeozoic basement fault in northern Jura. This fault clearly cuts through the décollement and probably formed during the thick-skinned post-4–3 Ma tectonic stage. (f) Seismic evidence of inversion of a Permo-Carboniferous graben underneath the Jura and post-dating thin-skinned folding and thrusting (modified after Philippe et al. Reference Philippe, Colletta, Deville, Mascle, Ziegler and Horvath1996).
The Jura has for a long time been considered a typical thin-skinned FTB. At its northern and western rims, respectively, the Jura belt overthrusts the southern Rhinegraben and the eastern Bresse graben, two branches of the Oligocene West European Rift System. The deposition and pinching out of the Triassic evaporites, together with the pre-orogenic Oligocene extensional structures that caused offsets of the décollement prior to shortening, strongly controlled not only the emplacement and geometry of the thin-skinned outermost thrusts and folds, but also the kinematics of the entire FTB. The formation of the thin-skinned Jura FTB is usually considered a rather short-lived event, starting around 14–11 Ma and terminating at nearly 4–3 Ma (e.g. Becker, Reference Becker2000).
On the basis of reflection seismic profiles and cross-section balancing, inversion of Permo-Carboniferous grabens (Fig. 10d) and reactivation of their bounding normal faults underneath the Jura and post-dating thin-skinned folding and thrusting have been documented (Philippe et al. Reference Philippe, Colletta, Deville, Mascle, Ziegler and Horvath1996). As shown in Figure 10b, f, this inversion caused basement uplift and late deformation/refolding of the earlier thin-skinned cover nappes. In the northern Jura, seismic data demonstrate that the faults underlying some of the northernmost folds are high-angle reverse faults that extend down into the basement, supporting that thick-skinned tectonics prevailed here (Rotstein & Schaming, Reference Rotstein and Schaming2004). Ustaszewski & Schmid (Reference Ustaszewski and Schmid2006, Reference Ustaszewski and Schmid2007) later provided additional seismic evidence for basement-involved shortening. Figure 10e shows an E–W-trending, N-dipping high-angle basement fault of late Palaeozoic origin reactivated in compression that truncates the syn-rift fill and almost reaches the surface. Because this fault clearly cuts through the décollement, it most probably formed during a post 4–3 Ma thick-skinned tectonic stage. Geomorphological evidence from the southernmost Rhinegraben revealed the spatial coincidence between gentle anticlines in the base of the Pliocene fluvial Sundgau gravels and transpressively/compressively reactivated basement faults (Giamboni et al. Reference Giamboni, Ustaszewski, Schmid, Schumacher and Wetzel2004). These observations altogether confirm that in the Jura thin-skinned tectonics was followed by thick-skinned tectonics. A crustal décollement presumably rooting at the upper–lower crust transition and extending beneath the Molasse Basin and the Internal Jura is required to accommodate displacements and thick-skinned shortening related to inversion of the Permo-Carboniferous basins (Pfiffner, Erard & Stauble, Reference Pfiffner, Erard, Stauble, Pfiffner, Lehner, Heitzmann, Mueller and Steck1997; Lacombe & Mouthereau, Reference Lacombe and Mouthereau2002). The ongoing character of thick-skinned tectonics in the Jura is evidenced by seismological data as well as levelling data (Meyer et al. Reference Meyer, Lacassin, Brulhet and Mouroux1994; Mosar, Reference Mosar1999; Lacombe & Mouthereau, Reference Lacombe and Mouthereau2002). Seismic activity testifies that the whole crust is actively deforming in the entire internal Jura-Molasse basin domain and is not limited to the Mesozoic cover. The close spatial relationships between the occurrence of seismicity, basement thrusting and the presence of inverted Permo-Carboniferous basins beneath the Mesozoic cover suggest triggering of thick-skinned tectonics by pre-existing inherited extensional structures (Lacombe & Mouthereau, Reference Lacombe and Mouthereau2002) under the Plio-Pleistocene and modern Alpine stress fields.
Madritsch, Schmid & Fabbri (Reference Madritsch, Schmid and Fabbri2008) provided evidence for two contrasting styles of Neogene to recent contraction along the northwestern Jura front (Fig. 10c). Late Miocene to early Pliocene thin-skinned deformation dominated in the Besançon Zone. Palaeostress directions reveal a consistently fanning pattern. Thick-skinned deformation, involving both Mesozoic cover and Palaeozoic basement, is documented in the Avant–Monts Zone and has taken place under a NW–SE compression. This deformation is associated with compressional to transpressional reactivation of pre-existing normal faults of Palaeogene to Palaeozoic age of the intracontinental Rhine–Bresse Transfer Zone and the underlying late Palaeozoic Burgundy Trough. The complex structural setting at the northwestern Jura front (Fig. 10c) thus results from spatial interferences between the two different styles of deformation. Deep-seated seismogenic thick-skinned tectonics and, local, presumably largely aseismic, still active shallow décollement tectonics may interact in space and time from early Pliocene times (4.2 Ma) to the present (Madritsch, Schmid & Fabbri, Reference Madritsch, Schmid and Fabbri2008).
To summarize, the tectonic evolution of the Molasse Basin – Jura Mountains system comprises both thin-skinned tectonics along a basal décollement within the Triassic evaporites (e.g. Philippe et al. Reference Philippe, Colletta, Deville, Mascle, Ziegler and Horvath1996) and basement shortening above a deeper décollement (Pfiffner, Erard & Stauble, Reference Pfiffner, Erard, Stauble, Pfiffner, Lehner, Heitzmann, Mueller and Steck1997), which accounts for the inversion of underlying Permo-Carboniferous grabens (Fig. 10d) and accommodates stacking of basement thrust units beneath the ECMs (Lacombe & Mouthereau, Reference Lacombe and Mouthereau2002; Bellahsen et al. Reference Bellahsen, Mouthereau, Boutoux, Bellanger, Lacombe, Jolivet and Rolland2014). Deep-seated thrusting occurred (during and) after the cover detached along the basal Triassic décollement, causing basement uplift and late deformation of the thin-skinned nappes. The most plausible explanation so far for the transition from thin-skinned tectonics to basement-rooted deformation is ongoing tectonic underplating in the northwestern Alpine foreland with the Alpine wedge evolving to reach a new state of equilibrium (Mosar, Reference Mosar1999).
6. Basement uplifts in retro-forelands: Laramide province and Sierras Pampeanas
Although observed at many places in the world, foreland basement-cored arches are still poorly understood owing to the fact that they often occur distant from plate boundaries (e.g. Yeck et al. Reference Yeck, Sheehan, Anderson, Erslev, Miller and Siddoway2014). These arches comprise many prominent mountain ranges including the Tian Shan, the Sierras Pampeanas of Argentina and much of the US Rocky Mountains.
The Andean system displays distinctive along- and across-strike changes in structural style that correlate with changes in both crustal architecture and plate dynamics (Ramos, Cristallini & Perez, Reference Ramos, Cristallini and Perez2002; Ramos et al. Reference Ramos, Zapata, Cristallini, Introcaso and McClay2004). To this respect, the Precordillera and Sierras Pampeanas in western Argentina show an outstanding analogy with the Sevier and Laramide belts, respectively (Jordan & Allmendinger, Reference Jordan and Allmendinger1986; Fielding & Jordan, Reference Fielding, Jordan, Schmidt and Perry1988; Fig. 11a), although some differences exist (Yonkee & Weil, Reference Yonkee and Weil2015).

Figure 11. (a) Modern geodynamic setting of the Sierras Pampeanas of Argentina compared to the early Eocene geodynamic setting of the Laramide uplifts in the foreland of the Rockies (modified after Jordan & Allmendinger, Reference Jordan and Allmendinger1986). (b) Structural section across the Precordillera and the Sierras Pampeanas (location shown by heavy line in a) (Bellahsen et al. Reference Bellahsen, Sebrier, Siame, Lacombe, Ruh, Brown and Nilfouroushan2016, this issue). (c) Structural section across the easternmost Sevier thin-skinned FTB and the Laramide basement uplifts (location shown by heavy line in a) (modified after Stone, Reference Stone, Schmidt, Chase and Erslev1993; Marshak, Karlstrom & Timmons, Reference Marshak, Karlstrom and Timmons2000). (d) Possible models of accommodation of Laramide uplifts at the crustal/lithospheric scale, which could also apply to the Sierras Pampeanas.
6.a. Laramide uplifts
The Laramide belt consists of the deformed and disrupted foreland of the former Sevier orogeny and developed at the expense of North American cratonic lithosphere (Yonkee & Weil, Reference Yonkee and Weil2015). The belt exhibits a network of anastomosing thick-skinned, basement-cored anticlines and uplifts (the so-called ‘arches’) separated by broad basins, which developed more than 1000 km inboard from the plate margin, spanning a region from southern Montana to New Mexico (Erslev, Reference Erslev, Schmidt, Chase and Erslev1993; Fig. 11a). The Laramide arches are bounded by moderate-dipping reverse faults with offsets of ~10–30 km and continue upwards into folds in the sedimentary cover (Brown, Reference Brown, Schmidt and Parry1988; Stone, Reference Stone, Schmidt, Chase and Erslev1993; Fig. 11c). Major reverse faults are imaged geophysically to mid-crustal (~20 to 30 km) depths (Smithson et al. Reference Smithson, Brewer, Kaufman, Oliver and Hurich1979; Lynn, Quam & Thompson, Reference Lynn, Quam and Thompson1983) and likely flatten in the lower crust or within a mid-crustal weak zone (Fig. 11c). The various local trends of the arches, compared to the overall NW–SE grain of the Laramide belt, has been tentatively explained by the reactivation of pre-existing crustal weaknesses with different orientations, including metamorphic foliations, shear zones, dykes and Proterozoic normal faults, which partly influenced localization of deformation (Mitra & Frost, Reference Mitra and Frost1981; Marshak, Karlstrom & Timmons, Reference Marshak, Karlstrom and Timmons2000; Stone, Reference Stone2002; Neely & Erslev, Reference Neely and Erslev2009).
Shortening accommodated by reverse faults and folds is ~50 km across the Wyoming foreland (Stone, Reference Stone, Schmidt, Chase and Erslev1993; Fig. 11c). Low-temperature thermochronometry (e.g. Fan & Carrapa, Reference Fan and Carrapa2014) as well as thickness variations, progressive unconformities and clastic content of the Maastrichtian to lower Eocene synorogenic strata indicate that Laramide deformation lasted from 70 to 50 Ma, temporally overlapping with late Sevier deformation (e.g. Dickinson et al. Reference Dickinson, Klute, Hayes, Janecke, Lundin, McKittrick and Olivares1988; see synthesis in Yonkee & Weil, Reference Yonkee and Weil2015). However, within the Laramide belt, deformation did not propagate regularly in a classical ‘in sequence’, forelandward (eastward) fashion; for instance, Crowley et al. (Reference Crowley, Reiners, Reuter and Kaye2002) documented an earlier uplift of the Bighorn Mountain arch located east of the Bighorn basin. Thus, depending on the more or less favourable orientation of pre-existing crustal weaknesses and anisotropies, individual arches may have developed in a rather complex sequence in space and time.
Laramide deformation roughly coincided spatially and temporally with Late Cretaceous to Palaeogene development of a flat-slab subduction segment, interpreted to have developed during subduction of the conjugate to the Shatsky Rise, a broad oceanic plateau that formed along the ancestral Pacific ridge (e.g. Livaccari, Burke & Şengör, Reference Livaccari, Burke and Şengör1981). Flat-slab subduction is known to be favoured by rapid convergence, trenchward absolute motion of the upper plate and increased buoyancy from thicker subducted crust (Gutscher et al. Reference Gutscher, Spakman, Bijwaard and Engdahl2000; van Hunen, van der Berg & Vlarr, Reference van Hunen, van den Berg and Vlarr2002; Espurt et al. Reference Espurt, Funiciello, Martinod, Guillaume, Regard, Faccenna and Brusset2008). These factors likely contributed to the ancient Laramide flat slab of the North American Cordillera (Saleeby, Reference Saleeby2003; Liu et al. Reference Liu, Gurnis, Seton, Slaeeby, Muller and Jackson2010), and currently contribute to the Andean 27–33°S flat-slab segment where the thick-skinned Sierras Pampeanas are actively uplifting (Fig. 11a).
Dynamic processes that could have enhanced stress transfer into the upper plate and produced thick-skinned crustal deformation are still largely debated (Yonkee & Weil, Reference Yonkee and Weil2015). Rather than being transmitted tangentially through continental basement rocks from the active plate boundary along the continental margin to the west, Laramide compressive stresses are considered in more recent views to arise from shear/basal traction between the continental lithosphere and the underlying flat slab of subducted oceanic lithosphere, from increased coupling along a cratonic lithosphere root, from hydrodynamic forces related to forced mantle convection, and/or from return flow where the slab steepened (Dickinson & Snyder, Reference Dickinson, Snyder and Matthews1978; Bird, Reference Bird1984, Reference Bird1988; Livaccari, Reference Livaccari1991; Tikoff & Maxson, Reference Tikoff and Maxson2001; English, Johnston & Wang, Reference English, Johnston and Wang2003; O'Driscoll, Humphries & Saucier, Reference O'Driscoll, Humphreys and Saucier2009; Jones et al. Reference Jones, Farmer, Sageman and Zhong2011). Such specific boundary conditions enhancing interplate coupling (see Section 9), possibly associated with a particular rheological behaviour of the upper plate tectonized lithosphere either inherited or linked to the geodynamic flat subduction setting itself (cooling, hydration) (see Sections 8 and 9), caused diffuse intraplate shortening and related basement uplifts.
Different tectonic models have been proposed that predict kinematics of shortening in the upper plate, leading to the rise of foreland arches (Erslev, Reference Erslev, Kalstrom and Keller2005); these include buckling of the upper-plate lithosphere (Tikoff & Maxson, Reference Tikoff and Maxson2001), buckling and folding of the upper crust driven by mid-crustal décollement (Erslev & Rogers, Reference Erslev, Rogers, Schmidt, Chase and Erslev1993; Yeck et al. Reference Yeck, Sheehan, Anderson, Erslev, Miller and Siddoway2014) or sub-crustal shear (Fig. 11d). Buckling and fault-propagation folding in the upper crust accommodated by pure shear thickening in the lower crust has also been proposed (Egan & Urquhart, Reference Egan and Urquhart1993). These mechanisms are not mutually exclusive: the most recent geophysical investigations of the crustal structure of the Laramide arches (Bighorn Arch Seismic Experiment) (Worthington et al. Reference Worthington, Miller, Erslev, Anderson, Chamberlain, Sheehan, Yeck, Harder and Siddoway2016) suggest that Laramide shortening of the Bighorn Arch may have been controlled by one, or a combination, of the following: (1) nucleation of deformation on a pre-existing weakness associated with a Precambrian tectonic boundary at the eastern edge of the Bighorn Arch; (2) localization of deformation in an area with relatively weak lower crust compared to surrounding regions; (3) accommodation of deformation by a crustal décollement focused into the upper crust by a regional Moho high, possibly enhanced by a small amount of lithospheric buckling, which allowed the emergent thrusting of the Bighorn master thrust.
6.b. Sierras Pampeanas
The Sierras Pampeanas ranges are located east of the Andes and extend between latitudes 27°S and 33°S and longitudes 64°W and 68°W (Fig. 11a) in Argentina. There, the Andean foreland displays a wide retro-arc belt. Two compressional, ~N–S-striking, opposite-verging structural domains are facing: (1) the ~40 km wide, E-verging, thin-skinned Precordillera FTB made of Palaeozoic rocks and extending just to the east of the main high Andes, and (2) the ~350 km wide, thick-skinned Sierras Pampeanas domain with mostly W-verging basement uplifts made of Precambrian and lower Palaeozoic metamorphic rocks. The E-verging thin-skinned thrusts initiated by early to middle Miocene times, earlier than the Pampean basement uplifts, which started between late Miocene times in the north and Pliocene–Quaternary times in the south. Accordingly, these basements uplifts are located within the distal part of the Miocene foreland basin where they occurred as out-of-sequence faulted blocks, giving birth to a broken foreland (e.g. Allmendinger et al. Reference Allmendinger, Ramos, Jordan, Palma and Isacks1983; Strecker et al. Reference Strecker, Cerveny, Bloom and Malizia1989, Reference Strecker, Hilley, Bookhagen, Sobel, Busby and Azor2012; Hain et al. Reference Hain, Strecker, Bookhagen, Alonso, Pingel and Schmitt2011) (see Section 10). These block uplifts are controlled by the reactivation of inherited crustal weaknesses, like Proterozoic or Palaeozoic(?) sutures and late Palaeozoic or Cretaceous normal faults (e.g. Kley & Monaldi, Reference Kley and Monaldi2002; Ramos, Cristallini & Perez, Reference Ramos, Cristallini and Perez2002), which explain their variable lengths from several tens to several hundreds of kilometres.
The Sierras Pampeanas basement block uplifts developed where the Nazca slab, which is subducting eastwards below the western edge of the South American plate, flattens below the Andes at a depth of ~100 km (e.g. Jordan et al. Reference Jordan, Isacks, Allmendinger, Brewer, Ramos and Ando1983; Smalley & Isacks, Reference Smalley and Isacks1987; Cahill & Isacks, Reference Cahill and Isacks1992; Anderson et al. Reference Anderson, Alvarado, Zandt and Beck2007). Initial shallowing of the subduction zone was possibly underway by ~20–18 Ma and lasted until 10 Ma, causing initiation of thrusting and basin formation in the Precordillera; the main shallowing phase occurred at ~10–5 Ma, with continued thrusting in the Precordillera, and major uplift of the Sierras Pampeanas beginning at ~6 Ma (Kay & Abbruzzi, Reference Kay and Abbruzzi1996). This flat-slab geometry appears to result from the subduction of the Juan Fernandez aseismic ridge that initiated between 14 Ma and 11 Ma and increased the buoyancy of the Nazca plate (Pilger, Reference Pilger1981; Gutscher et al. Reference Gutscher, Spakman, Bijwaard and Engdahl2000).
The section of Figure 11b cuts across part of a ~400 km wide domain of the South-Central Sierras Pampeanas. There, the depth of the continental Moho varies from 66 km below the Central Precordillera (Ammirati et al. Reference Ammirati, Alvarado, Perarnau, Saez and Monsalvo2013) to 52 km below the Sierra Pie de Palo (Calkins et al. Reference Calkins, Zandt, Gilbert and Beck2006), while it is only 38 km and 35 km below the western and eastern parts of the Sierra de Cordoba, respectively (Perarnau et al. Reference Perarnau, Gilbert, Alvarado, Martino and Anderson2012). Crustal seismicity (Regnier et al. Reference Régnier, Chatelain, Smalley, Chiu, Isacks and Puebla1992; Smalley et al. Reference Smalley, Pujol, Regnier, Chiu, Chatelain, Isacks, Araujo and Puebla1993) is seemingly limited at depth by a ~34 km deep seismic discontinuity (noticeably, a depth similar to the 32 km flattening depth of the Wind River thrust (Lynn, Quam & Thompson, Reference Lynn, Quam and Thompson1983) and more generally to the depth of the weak crustal zone where Laramide crustal faults presumably root in the Laramide province (Marshak, Karlstrom & Timmons, Reference Marshak, Karlstrom and Timmons2000; Fig. 11c)), while a shallower one is observed at a depth of ~18 km (Calkins et al. Reference Calkins, Zandt, Gilbert and Beck2006; Alvarado, Beck & Zandt, Reference Alvarado, Beck and Zandt2007, Ammirati, Alvarado & Beck, Reference Ammirati, Alvarado and Beck2015). This seismic discontinuity near the base of the crustal seismicity could correspond to the main Andean décollement that likely allowed Andean shortening to propagate eastwards to the Sierra Pampeanas. This deep crustal seismicity (e.g. Smalley & Isacks, Reference Smalley and Isacks1990) is consistent with a low thermal gradient, in agreement with the flat-slab subduction setting, for which thermal modelling indicates a value of ~10° km−1 caused by the lack of asthenospheric wedge (Gutscher et al. Reference Gutscher, Spakman, Bijwaard and Engdahl2000) to be compared to values of around ~30° km−1 above steeper Andean subduction segments, and in agreement with the low thermal gradients reported in the Neogene basins (Collo et al. Reference Collo, Davila, Nobile, Astini and Gehrels2011; Davila & Carter, Reference Davila and Carter2013).
An example of basement uplift is provided by the Pie de Palo range (Fig. 11b). It is an actively growing basement anticline bounded by major faults and associated with a high level of crustal seismicity (Régnier et al. Reference Régnier, Chatelain, Smalley, Chiu, Isacks and Puebla1992; Smalley et al. Reference Smalley, Pujol, Regnier, Chiu, Chatelain, Isacks, Araujo and Puebla1993; Ramos, Cristallini & Perez, Reference Ramos, Cristallini and Perez2002; Siame et al. Reference Siame, Bellier, Sébrier, Bourlès, Leturmy, Perez and Araujo2002, Reference Siame, Sebrier, Bellier, Bourlès, Costa, Ahumada, Gardini and Cisneros2015; Siame, Bellier & Sébrier, Reference Siame, Bellier and Sébrier2006). The Tulum fault system (Zambrano & Suvires, Reference Zambrano and Suvires2008) limits the western edge of the Pampean Precambrian outcrops from the Palaeozoic outcrops of the Eastern Precordillera (Ramos, Cristallini & Perez, Reference Ramos, Cristallini and Perez2002) and corresponds further south to the western front of the active Pampean thrust system (Verges et al. Reference Verges, Ramos, Meigs, Cristallini, Bettini and Cortes2007; Cisneros, Costa & Gardini, Reference Cisneros, Costa and Gardini2010). The structure controlling the eastern border of the Pie de Palo range is considered either as dipping east (Langer & Bollinger, Reference Langer and Bollinger1988; Costa et al. Reference Costa, Machette, Dart, Bastias, Paredes, Perucca, Tello and Haller2000; Ramos, Cristallini & Perez, Reference Ramos, Cristallini and Perez2002) or west (Kadinsky-Cade, Reilinger & Isacks, Reference Kadinsky-Cade, Reilinger and Isacks1985; Reilinger & Kadinsky-Cade, Reference Reilinger and Kadinsky-Cade1985; Fig. 11b). The significance of the Pie de Palo anticline is still debated, being interpreted either as a W-verging fault-bend fold or an E-verging fault-propagation fold.
Summarizing, the striking similarity between the Sierras Pampeanas of Argentina and the Laramide uplifts strongly suggests that flat-slab subduction at an advancing subduction zone, possibly enhanced by increased coupling along a cratonic lithosphere root, appears to be a favourable setting to develop a wide retro-arc compressional province, leading to diffuse intraplate basement shortening well inboard the plate boundary. This setting is also associated with a low temperature gradient within the upper plate. This similarity suggests that tectonic models proposed to explain stress transfer and shortening in the upper plate in the Laramide belt, such as lithospheric buckling or fault-propagation folding of the upper crust driven by mid-crustal décollement, may also apply to the Sierras Pampeanas. The overall oceanward (westward) vergence of both the Laramide and Sierras Pampeanas uplifts, opposite to the E-verging, thin-skinned Sevier and Precordillera FTBs, respectively, may additionally suggest that shearing at the base of the upper plate crust/lithosphere under the far foreland in response to flat-slab subduction may have been significant.
7. Styles, kinematics and mechanics of basement-cored folding
In basement-cored anticlines, the overlying sedimentary cover is folded. If either the underlying basement–cover unconformity or the basement cannot be directly or entirely observed, the first point to address is whether basement rocks are really folded or not. If yes, one must examine how to fold the crystalline basement rocks usually considered as rigid and isotropic to a first order. If not, the question is to understand how the cover may fold above an unfolded basement. Examination of basement uplifts and related cover deformation in the Laramide belt, Sierras Pampeanas and western Alps provides some insights into these questions.
7.a. Basement-cored anticlines and basement uplifts: Laramide province and Sierras Pampeanas
In the Laramide province of the central Rocky Mountains, there is an overall agreement that the formation of basement thrusts within the Precambrian basement is a prerequisite for the development of thick-skinned folds within the overlying sedimentary cover (Stone, Reference Stone, Schmidt, Chase and Erslev1993; Fig. 12a, b). The variation in map view and in 3D geometries of individual structures, as well as their mutual spatial and angular relationships, is strongly suggestive of the influence of pre-existing basement discontinuities at depth (Paul & Mitra, Reference Paul and Mitra2012). The pattern of basement uplifts are therefore controlled by reactivated faults at depth (that in most places remain blind, either covered by Cenozoic sediments, or expressed at the surface as a monoclinal fault-propagation fold of Palaeozoic and Mesozoic strata), with only neoformed offshoots of those faults being most of the time exposed at the surface (Bump, Reference Bump2003).
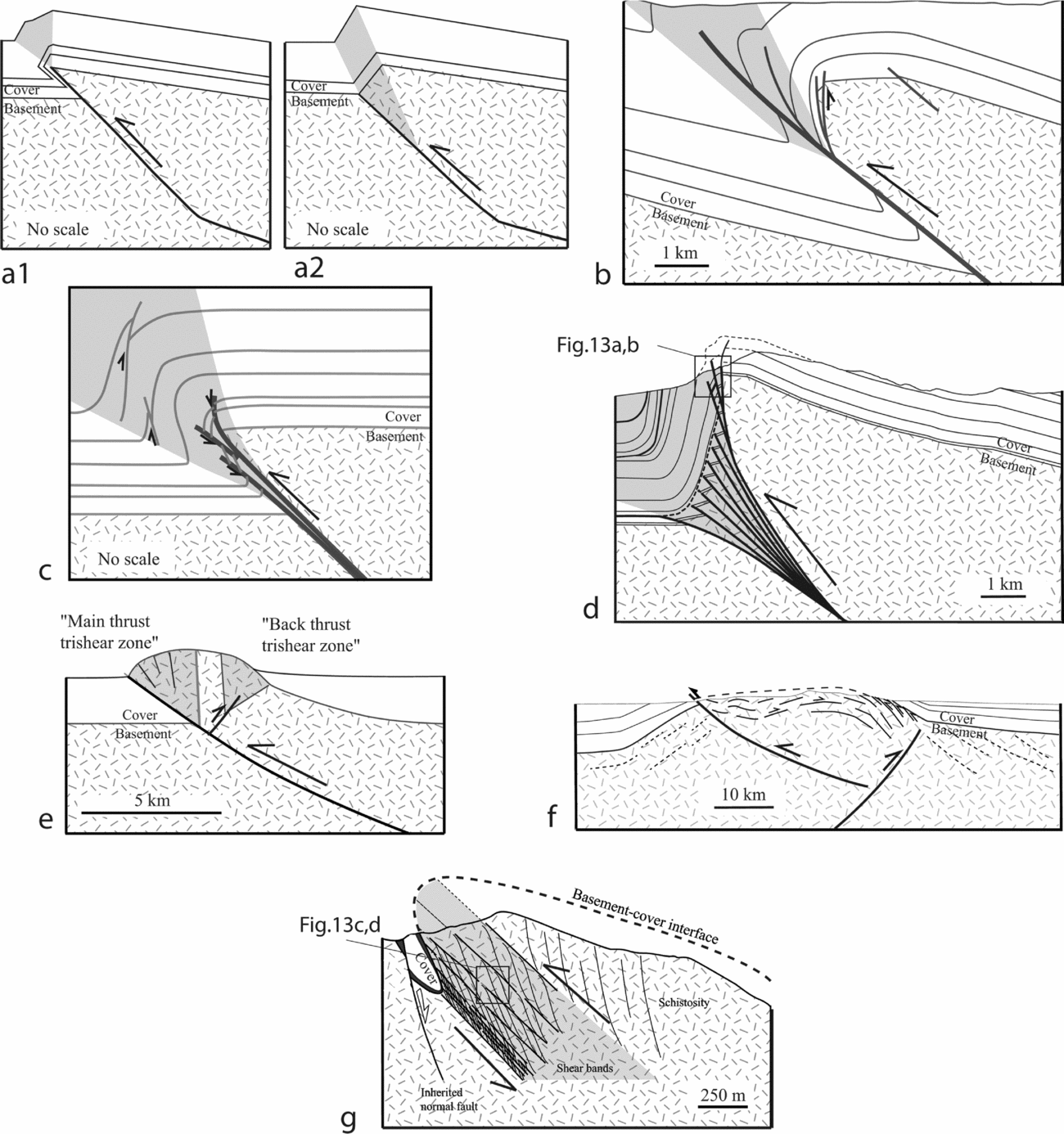
Figure 12. Basement-cored folding. The shaded area denotes the domain where basement and/or cover are physically damaged and strained as a result of the accommodation of slip along the master thrust. (a) Folds where the forelimb basement–cover interface is a fault (a1) compared to folds where the forelimb basement–cover interface is a rotated unconformity (a2) (modified after Schmidt, Genovese & Chase, Reference Schmidt, Genovese, Chase, Schmidt, Chase and Erslev1993). (b) Section across the Maverick Spring anticline (Laramide belt) (modified after Stone, Reference Stone, Schmidt, Chase and Erslev1993). (c) Trishear model (Erslev, Reference Erslev1991). (d) Interpretation of the Rattlesnake anticline (Laramide belt), involving distributed deformation along splay faults at the tip of the basement fault and at the top of the basement (Beaudoin et al. Reference Beaudoin, Leprêtre, Bellahsen, Lacombe, Amrouch, Callot, Emmanuel and Daniel2012). (e) Sierra de Hualfın anticline (Sierras Pampeanas): basement folding is accommodated by both a main thrust trishear zone and a subordinate back-thrust trishear zone (modified after Garcia & Davis, Reference Garcia and Davis2004). (f) Sierra Pie de Palo anticline (Sierras Pampeanas): basement shortening is accommodated by reverse faults localized along inherited foliation planes in the basement (Bellahsen et al. Reference Bellahsen, Sebrier, Siame, Lacombe, Ruh, Brown and Nilfouroushan2016, this issue). (g) Oisans ECM (western Alps): ‘folding’ of the basement–cover interface is spatially associated with low-angle basement shear zones distributed over a large thickness (modified after Bellahsen et al. Reference Bellahsen, Jolivet, Lacombe, Bellanger, Boutoux, Garcia, Mouthereau, Le Pourhiet and Gumiaux2012).
Thrusts are nearly planar within the basement, then propagate and steepen upwards, usually accompanied by fold backlimb rotation (Stone, Reference Stone, Schmidt, Chase and Erslev1993). The overlying Phanerozoic sedimentary cover is uplifted and stretched over rising hangingwall basement blocks, and ultimately offset by the propagating thrusts along the forelimbs of anticlines.
One of the important factors about fault-related folds in basement rocks is whether the basement–cover contact on the steep forelimb of the fold is a fault contact or a rotated unconformity. This implies a different behaviour below the cover (Fig. 12a1, a2) (Schmidt, Genovese & Chase, Reference Schmidt, Genovese, Chase, Schmidt, Chase and Erslev1993). In some folds, the forelimb basement–cover interface is a fault (Fig. 12a1). These folds developed when there is a lack of significant foliation or weaknesses in the basement, which is therefore basically isotropic at the time of shortening (Schmidt, Genovese & Chase, Reference Schmidt, Genovese, Chase, Schmidt, Chase and Erslev1993). In this case, all happens as if a relatively competent basement block was indenting a relatively incompetent cover, resulting in no significant basement deformation. In contrast, in other folds, the forelimb basement–cover interface is a rotated unconformity (Fig. 12a2). These folds are seemingly associated with a relatively deformable basement block forced against a competent cover. Basement can be deformed through slip on sets of closely spaced fractures, through flexural slip on pre-existing foliation oriented sub-parallel to bedding, through axial surface-parallel slip on foliation favourably oriented for simple shearing parallel to the master fault or through pervasive cataclasis (Schmidt, Genovese & Chase, Reference Schmidt, Genovese, Chase, Schmidt, Chase and Erslev1993; Chase, Schmidt & Genovese, Reference Chase, Schmidt, Genovese, Schmidt, Chase and Erslev1993).
In the case where metamorphic foliation in the basement rocks is parallel (or nearly parallel) to bedding of the cover rocks, folding of the entire package may occur by flexural slip, giving rise to a true basement fold (Schmidt, Genovese & Chase, Reference Schmidt, Genovese, Chase, Schmidt, Chase and Erslev1993). Passive folding of the basement may also occur by combined thrust and back-thrust folding assisted by fracturing (Garcia & Davis, Reference Garcia and Davis2004; Fig. 12e). Alternatively, the curved attitude of the basement–cover interface may only mimic true basement folding. Several mechanisms may account for such folding of cover rocks without folding of basement rocks, such as basement faulted by a single fault or by several faults (e.g. Fig. 12b), or by fault zones containing wedges of cataclastic material (e.g. Erslev, Reference Erslev1986; Spang & Evans, Reference Spang, Evans, Schmidt and Perry1988; Stone, Reference Stone, Schmidt, Chase and Erslev1993; Narr, Reference Narr, Schmidt, Chase and Erslev1993; Schmidt, Genovese & Chase, Reference Schmidt, Genovese, Chase, Schmidt, Chase and Erslev1993; Narr & Suppe, Reference Narr and Suppe1994).
The mechanical response of the basement rocks as well as the overall fold geometry is highly dependent on the temperature and confining pressure during deformation, the nature and orientation of the pre-deformation fabric of the basement rocks and the competence of the cover rocks and the degree to which folded strata are decoupled or welded to the basement blocks. In the Laramide arches, Schmidt, Genovese & Chase (Reference Schmidt, Genovese, Chase, Schmidt, Chase and Erslev1993) estimated the depth at the upper basement rock surface during folding at about 2.5 to 5 km, hence a lithostatic pressure in the range 70–135 MPa, and a temperature during deformation in the range 70–120°C, which suggests a brittle (or semi-brittle) mechanical behaviour of the basement rocks.
The propagation of the tip of an advancing basement fault creates, beyond the tip, a physical ground preparation of the medium that not only may accommodate further tip advance but also folding of the material through which the tip may propagate (Bump, Reference Bump2003; Garcia & Davis, Reference Garcia and Davis2004). In the trishear example (Erslev, Reference Erslev1991; Fig. 12c), the trishear angle, combined with slip magnitude, influences the size of the basement–cover folded interface. The damage in the trishear zone, in combination with pre-deformational anisotropies, may even give to granite a kind of macroductility (Garcia & Davis, Reference Garcia and Davis2004). Another key factor controlling folding of the basement is the initial position of the tip of the thrust fault that caused basement uplift. Basement-cored uplifts that form as a result of a fault that propagates over a short distance from the basement–cover interface or from the interface (i.e. reactivation of a previous fault or fracture) show minor deformation of the basement and considerable deformation of the overlying sedimentary rocks (Fig. 12c; Garcia & Davis, Reference Garcia and Davis2004). However, if the fault tip is initially located deep in the basement and does not propagate to the basement–cover interface but remains in the basement while slip is fed into the fault, then basement rocks above the fault tip must deform in order to accommodate slip (Bump, Reference Bump2003). To this respect, Figure 12d shows the interpretation of the Rattlesnake Mountain anticline by Beaudoin et al. (Reference Beaudoin, Leprêtre, Bellahsen, Lacombe, Amrouch, Callot, Emmanuel and Daniel2012). This interpretation involves distributed deformation along splay faults at the tip of the basement fault and at the top of the basement (Beaudoin et al. Reference Beaudoin, Leprêtre, Bellahsen, Lacombe, Amrouch, Callot, Emmanuel and Daniel2012). In this model, the tip of the basement fault is not located just at the basement–cover interface but deeper in the basement, which implies that the triangle zone where distributed deformation/damage occurs (splays faults, with cataclasis or intensively fractured rocks) is located within the basement. This particular behaviour could be tentatively related to high fluid pressure and related hydrofracturing leading to distributed brittle deformation. The behaviour of the basement that crops out along the Shoshone river (Fig. 13a) is clearly brittle, with high-angle reverse faults being parallel to (likely pre-existing) major fractures in the basement (Fig. 13b).

Figure 13. Field examples of basement-involved shortening. (a) Photograph and schematic interpretative cross-section of the Rattlesnake Mountain anticline. (b) Field evidence of the brittle behaviour of the basement rocks that crop out along the Shoshone river in the Rattlesnake Mountain anticline, showing high-angle reverse faults parallel to major fractures within the basement (modified after Beaudoin et al. Reference Beaudoin, Leprêtre, Bellahsen, Lacombe, Amrouch, Callot, Emmanuel and Daniel2012). (c) Field evidence of W-verging reverse shear zones within the basement of the Oisans ECM. Note the sigmoidal shape of the Alpine schistosity (S) within the shear zones. (d) Evidence for mylonitic deformation associated with basement shear zones in the Oisans Massif (western Alps) (modified after Bellahsen et al. Reference Bellahsen, Jolivet, Lacombe, Bellanger, Boutoux, Garcia, Mouthereau, Le Pourhiet and Gumiaux2012). Qz – quartz.
On the basis of their study of the Sierra de Hualfın in Argentina, Garcia & Davis (Reference Garcia and Davis2004) emphasized that the most important condition for the crystalline basement to fold is its rheology during deformation (e.g. Chase, Schmidt & Genovese, Reference Chase, Schmidt, Genovese, Schmidt, Chase and Erslev1993). The deformability of the basement may be achieved by pervasive faults and fractures that pre-dated, and/or formed during, folding. Because the basement is always pervaded with fractures and possible additional metamorphic or dyke fabrics, it no longer behaves as a rigid and isotropic medium and may fold.
In the Sierra de Hualfın, folding is accommodated by both a main thrust trishear zone and a subordinate back-thrust trishear zone (Fig. 12e; Garcia & Davis, Reference Garcia and Davis2004). At shallow basement levels, the smooth folded shape of the basement unconformity is achieved through movements on joints, minor faults and ‘brittle’ foliation. Faulting along spaced reactivated joints imparts an overall fold shape to the basement. Deeper in the basement, the combination of joints and faults allows the deformation and distortion of the basement rocks into a fold shape. Garcia & Davis (Reference Garcia and Davis2004) challenged models of basement-cored uplifts that assume that the fault tip is located at the basement–cover interface, and stressed that some basement-cored anticlines can be attributed to basement distortion taking place in advance of a propagating fault tip below the basement–cover unconformity.
In the Sierra Pie de Palo, basement shortening occurred at very low depth, i.e. the basement top was less than 1 km deep, and was accommodated on several reverse faults that deform the Tertiary continental beds (Bellahsen et al. Reference Bellahsen, Sebrier, Siame, Lacombe, Ruh, Brown and Nilfouroushan2016, this issue). These faults are not as large and as deep as previously proposed but are rather small and short faults that accommodate a large-scale ‘folding’/buckling of the crust. The proposed ‘folding’ mechanism thus involves reverse faults localized along inherited foliation planes in the basement. There, fine-grained foliation planes localized foliation-parallel shearing, which evolved as small-scale asymmetric folds and ultimately into cataclastic zones that constitute the present-day faults (Fig. 12f). Thus, it appears that the shortening has been achieved by reverse faults distributed in the crust that reactivated pre-existing basement anisotropies and weaknesses (inherited foliation planes) and accommodated a large-scale antiform above an E-verging blind thrust (Fig. 11b) (Bellahsen et al. Reference Bellahsen, Sebrier, Siame, Lacombe, Ruh, Brown and Nilfouroushan2016, this issue).
7.b. ‘Basement folds’ in the External Crystalline Massifs of the western Alps
In the ECMs, crustal thickening gave rise to so-called ‘basement folds’ (Ford, Reference Ford1996; Gidon, Reference Gidon1999; Dumont et al. Reference Dumont, Champagnac, Crouzet and Rochat2008). The northern Grandes Rousses cross-section (Fig. 9c3) displays two main ‘basement antiforms’, the eastern one being shown in Figure 12g. The two basement antiforms are separated by a tight syncline of Liassic rocks that is bounded to the west by a steep inherited Jurassic normal fault that offsets the Triassic layers and brings the Jurassic rocks directly over the basement. Because deformation in the central ECMs (Belledonne, Oisans) occurred at a maximum temperature of 330°C (Bellanger et al. Reference Bellanger, Bellahsen, Jolivet, Baudin, Augier and Boutoux2014) and under a tectonic burial of presumably about 10 km, these massifs provide the opportunity to address the question of basement folding at crustal depths greater than in the Laramide province and the Sierras Pampeanas.
Basement folding has been inferred from the curved attitude of the cover–basement interface as reflected by the Triassic layers that remained welded to the basement. However, the related deformation in the basement has only been recently deciphered. Bellahsen et al. (Reference Bellahsen, Jolivet, Lacombe, Bellanger, Boutoux, Garcia, Mouthereau, Le Pourhiet and Gumiaux2012) and Bellanger et al. (Reference Bellanger, Bellahsen, Jolivet, Baudin, Augier and Boutoux2014) described shear zones associated with a sigmoid-shaped Alpine schistosity that support reverse, top-to-the-W shear kinematics (Figs 12g, 13c). Close to the cover (Liassic) syncline, shear zones and schistosity are nearly parallel. Such maximum shearing of the basement is observed where the Triassic strata are steeply dipping and locally overturned.
The shear zones contain a large amount of chlorite and white micas suggesting that they developed under greenschist-facies conditions. Quartz clasts are mainly fractured but the overall mylonitic deformation is due to the large amount of white micas that grew coevally with quartz deformation (Fig. 13d), reflecting deformation in the brittle–ductile regime.
Dumont et al. (Reference Dumont, Champagnac, Crouzet and Rochat2008) suggested that the basement thrusts in the Oisans–Grandes Rousses massifs result from the reactivation of the steep Variscan foliation, which is ruled out by the shallow dip of the shear zones. Alternatively, Butler, Tavarnelli & Grasso (Reference Butler, Tavarnelli and Grasso2006) suggested that basement deformation might be due to the buckling of the upper crust characterized by lithology contrasts (especially the basement–cover interface). If the initiation of the deformation by buckling cannot be ruled out, shortening is most probably not only due to this mechanism as stressed by Bellahsen et al. (Reference Bellahsen, Jolivet, Lacombe, Bellanger, Boutoux, Garcia, Mouthereau, Le Pourhiet and Gumiaux2012): the spacing of the deformed zones (a few hundreds of metres to a few kilometres) is too small to be controlled by buckling and the preferential vergence of the shear zones does not support a buckling mechanism that would have implied a rather pure shear deformation. Thus, ‘folding’ of the basement–cover interface is clearly spatially associated with low-angle basement shear zones distributed over large thickness, and therefore linked to non-coaxial shearing in the basement, instead of true folding.
To conclude, basement-cored folding may be achieved by very different mechanisms depending on the rheological properties of the basement/cover rocks and the physical conditions of deformation (P, T, fluids). True basement folding is very rare and requires very specific conditions (e.g. well-oriented foliations) to occur, as shown above for the case of the Sierra Pie de Palo. In general, one faces cases of strain distribution, different from real folding and that are related to possible fluid circulation (e.g. Beaudoin et al. Reference Beaudoin, Bellahsen, Lacombe and Emmanuel2011), thermal synorogenic weakening (Bellahsen et al. Reference Bellahsen, Jolivet, Lacombe, Bellanger, Boutoux, Garcia, Mouthereau, Le Pourhiet and Gumiaux2012) or shear zones ahead of a thrust tip (Erslev, Reference Erslev1986, Reference Erslev1991; Bump, Reference Bump2003; Garcia & Davis, Reference Garcia and Davis2004).
8. Rheological aspects of basement-involved shortening and importance of structural/thermal inheritance
This section aims at discussing the possible control exerted by the rheology of the crust/lithosphere at the time of shortening on the structural style in FTBs.
8.a. Role of the intrinsic rheological structure of passive margins
Passive margins are key players in the collisional processes as the arrival of their proximal, poorly thinned parts into the subduction zone mark the onset of collision. The transition between continental and oceanic crusts is often marked by a wide domain of progressively thinner continental crust, with occasionally extensional upper crustal allochthons sheared off their lower crust and locally exhumed sub-continental mantle. Differential stretching of the lithosphere modifies its rheological properties (Fig. 14b), which will subsequently control deformation style during collision (Cloetingh et al. Reference Cloetingh, Ziegler, Beekman, Andriessen, Matenco, Bada, Garcia-Castellanos, Hardebol, Dezes and Sokoutis2005). As the crust thins and cools during progressive rifting, the reduction in overburden pressure and temperature makes the rocks which originally deformed by plastic creep gradually become more prone to brittle failure. The result is that the initial weak zones in the middle crust and deep crust disappear and that the entire crust becomes brittle (Fig. 14a). The important consequences of the progressive embrittlement of originally ductile rocks during lithospheric extension are (1) that lateral flow or displacement of particular layers within the crust should become progressively more difficult as rifting proceeds, and (2) that the upper crust becomes coupled to the mantle (Reston & Manatschal, Reference Reston, Manatschal, Brown and Ryan2011).

Figure 14. Change of lithospheric rheology during stretching and thinning during passive margin formation, which will subsequently control deformation style during collision. (a) Rheological model for a passive margin, emphasizing the effects of thinning and stretching of the lithosphere that lead to the disappearance of ductile middle and lower crust and embrittlement of the crust that is coupled to the mantle (modified after Reston & Manatschal, Reference Reston, Manatschal, Brown and Ryan2011). (b) Change in rheological properties of the lithosphere as a result of differential stretching (modified after Cloetingh et al. Reference Cloetingh, Ziegler, Beekman, Andriessen, Matenco, Bada, Garcia-Castellanos, Hardebol, Dezes and Sokoutis2005).
Summarizing, the occurrence of weak mechanical layers within the proximal passive margin lithosphere (the middle and most of the lower crust are expectedly ductile) (Fig. 14b) may therefore explain that subsequent contractional deformation will be distributed within most of the crust, giving rise to FTBs exhibiting a basement-involved tectonic style. In contrast, because these weak crustal levels are usually lacking in distal parts of the margins as a result of thinning, these stronger lithospheric domains are more prone to localized deformation in a continental subduction style. Noticeably, extensional upper crustal allochthons sheared off their lower crust during the rifting phase and subsequently shortened during collision may give rise to very thin basement nappes, as encountered in the Alps (Pfiffner, Reference Pfiffner, Lacombe, Ruh, Brown and Nilfouroushan2016, this issue) as a result of Mesozoic rifting and hyper-extension (Mohn et al. Reference Mohn, Manatschal, Masini and Muntener2011).
8.b. Role of pre-orogenic lithospheric thermotectonic inheritance
Correlation between spatial variations of the flexural rigidity of the lithosphere and the nature and amount of foreland deformation has been suggested for the Andes FTB (Watts et al. Reference Watts, Lamb, Fairhead and Dewey1995) and Taiwan (Mouthereau & Petit, Reference Mouthereau and Petit2003). These authors documented that regions with low Equivalent Elastic Thickness (Te) correlate with thick-skinned deformation whereas regions with high Te correlate with thin-skinned deformation. The underlying idea is that a strong lithosphere is less easily deformed so that shortening is localized in a narrow zone at shallow depth, while a weaker lithosphere enables crust–mantle decoupling and shortening of the whole crust. Mouthereau & Petit (Reference Mouthereau and Petit2003) emphasized that the local increase in plate coupling and inhomogeneities in a prefractured margin as in Taiwan (see Section 3) can affect the rigidity of the layered continental lithosphere, supporting a mechanical relationship between its strength and the structural style.
In foreland domains that did not undergo significant syn-collisional burial and heating, the age-dependent properties of the passive margin lithosphere at the time of its involvement in collisional deformation are key to explaining variations in the accommodation of continental deformation in external parts of orogens. Two distinct groups of orogens have been distinguished (Mouthereau, Watts & Burov, Reference Mouthereau, Watts and Burov2013; Fig. 15). The first group is characterized by high crustal strain (up to 70%) with deformation localized above a shallow décollement in the weak sedimentary cover, i.e. overall localized, simple-shear-type deformation (subduction style). This type is commonly associated with old (> 1 Ga), cold and strong cratonic lithosphere, where the subducted lower crust is coupled with the mantle. The second group is characterized by limited crustal strain (< 40%), revealing that deformation is distributed within the entire crust, with brittle crustal thrust ramps in the upper crust and flow in the ductile middle–lower crust, i.e. distributed, pure-shear-type deformation (collision style). This second type appears to be more commonly observed in FTBs built on young, hot and weak Phanerozoic lithosphere. These differences reflect the dependence of the lower crust and mantle strength on lithosphere thermal age (i.e. age of the last thermal event that led to modifying the thermal, hence rheological, state of the lithosphere; Mouthereau, Watts & Burov, Reference Mouthereau, Watts and Burov2013; Fig. 15).

Figure 15. Distinct groups of orogens recognized by Mouthereau, Watts & Burov (Reference Mouthereau, Watts and Burov2013) on the basis of the style of deformation, percentage of shortening in foreland FTBs that did not undergo significant syn-collisional burial and heating, and the thermotectonic age of the lithosphere at the time of its involvement in collisional deformation. The first group is characterized by high crustal strain (up to 70%) and is observed within old (>1 Ga), cold and strong cratonic lithosphere. The second group is characterized by limited crustal strain (< 40%) and is observed within young, hot and weak Phanerozoic lithosphere. Modified after Mouthereau, Watts & Burov (Reference Mouthereau, Watts and Burov2013).
One main implication is that in external orogenic domains, where syn-collisional burial is limited and transient deformation and dynamic weakening processes do not significantly alter the pre-collisional rheological layering, shortening of young lithosphere leads to basement-involved (thick-skinned) deformation, which reflects decoupling at the brittle–ductile transition or in the viscous lower crust.
The thermal state inherited from the last pre-orogenic rifting event has been further investigated by means of low-temperature thermochronology in the Pyrenees (Vacherat et al. Reference Vacherat, Mouthereau, Pik, Bernet, Gautheron, Masini, Le Pourhiet, Tibaric and Lahfid2014). These authors have shown that the high geothermal gradient related to Cretaceous hyper-extension and mantle exhumation lasted 30 Ma after the onset of convergence at ~83 Ma and was relaxed during the ‘collision’ phase from ~50 Ma. They further proposed that heat needed for ductile shortening during convergence in the NPZ has been primarily inherited from extension rather than being only related to tectonic and/or sedimentary burial. This study highlights how high temperatures inherited from the rifting event can affect the thermal structure of the crust during the early stages of mountain building. This hot crust was likely more prone to distributed deformation, hence thick-skinned tectonics, with or without reactivation of inherited normal faults.
Numerical modelling studies have recently addressed the structural style of mountain belts and have shown that the rheology of the basement–cover sequence and the rheological layering of the lithosphere have a strong control on this style (Jammes & Huismans, Reference Jammes and Huismans2012; Nilfouroushan et al. Reference Nilfouroushan, Pysklywec, Cruden and Koyi2013; Bauville & Schmalholz, Reference Bauville and Schmalholz2015). Jammes & Huismans (Reference Jammes and Huismans2012) showed by means of a 2D thermomechanical modelling approach that the relative strength of the frictional-plastic and viscous rheologies in the lithosphere affects the way it deforms in orogenic settings. Among the results pertaining to FTBs are: (1) systems with a weak upper and lower crust show deformation accommodated on a few thick-skinned crustal-scale thrusts with moderate displacement and by distributed thickening of the crust, as in the Zagros; (2) crustal systems characterized by a shallow decoupling zone in the mid-crust resulting from a thick and strong lower crust allows the formation of long thrust sheets and of an antiformal stack in the core of the orogen, as in the Pyrenees; and (3) weaknesses inherited from extensional deformation in the internal part of the chain affect the structure of the orogenic wedge and facilitate the propagation of the deformation in the external part of the chain.
8.c. Role of syn-convergence heating, hydration and ductilization
An alternative situation arises when the inner part of the foreland domain is buried and heated during convergence or when a particular setting like flat-slab subduction modifies the rheology of the overlying lithosphere. In this case, the inherited thermal-tectonic state of the lithosphere may be modified by subsequent heating or hydration, providing the crust/lithosphere with new rheological properties at the time of shortening.
In the western Alps, ductilization of the European crust underthrust below the Penninic units occurred under greenschist conditions at about 300–330°C with a rather long-lasting peak temperature (~10 Ma: Bellanger et al. Reference Bellanger, Augier, Bellahsen, Jolivet, Monie, Baudin and Beyssac2015). This ductilization likely provided favourable conditions for distributed shortening (Bellahsen et al. Reference Bellahsen, Jolivet, Lacombe, Bellanger, Boutoux, Garcia, Mouthereau, Le Pourhiet and Gumiaux2012, Reference Bellahsen, Mouthereau, Boutoux, Bellanger, Lacombe, Jolivet and Rolland2014) as illustrated in Figure 9c1, c2 in the ECMs. Later on, the ductilely deformed crust was exhumed by motion along localized frontal thrust ramps that connected and likely activated basal décollement of the cover nappes in the sub-alpine/Helvetic thin-skinned FTBs. The numerical simulations by Bauville & Schmalholz (Reference Bauville and Schmalholz2015) of the compression of a basement–cover system with half-grabens showed that (1) the transition between thin-skinned- and thick-skinned-dominated deformation is controlled by two effective viscosity ratios: the ratio inside the basement, that is the ratio of viscosity at the top of the basement to the viscosity at the bottom of basement, and the ratio of the viscosity at the top of the basement to the viscosity of the sedimentary cover directly above the basement, and (2) a higher basement-internal ratio favours thick-skinned deformation whereas a higher basement–cover ratio favours thin-skinned deformation. Applying their results to the Alpine Helvetic nappes of Switzerland, these authors demonstrated that formation of fold nappes (e.g. Morcles nappe) is associated with thick-skinned-dominated deformation whereas the formation of thrust nappes is instead related to thin-skinned-dominated deformation. This emphasizes the combined weakening effects of inherited extensional basins and burial on the crust that favour thick-skinned deformation (Bellahsen et al. Reference Bellahsen, Jolivet, Lacombe, Bellanger, Boutoux, Garcia, Mouthereau, Le Pourhiet and Gumiaux2012).
Taking into account the thermal–structural–rheological state of the crust at the time of shortening provides predictive insights into the way basement-involved, thick-skinned deformation may occur, namely with or without inversion tectonics. As suggested by Figure 16, partly inspired by the papers by Butler, Tavarnelli & Grasso (Reference Butler, Tavarnelli and Grasso2006) and Bellahsen et al. (Reference Bellahsen, Jolivet, Lacombe, Bellanger, Boutoux, Garcia, Mouthereau, Le Pourhiet and Gumiaux2012), the thermo-rheological state of the crust at the time of shortening, either inherited from pre-orogenic rifting events or later modified by early syn-convergence burial, has important consequences on the mode of deformation. Assuming a given reference strength of the crust (Fig. 16a), reactivation of inherited normal faults (as observed in the Taiwan and Laramide foreland) may be related to an abnormal weakness (weak Byerlee) of fault zones caused by local high fluid pressures or weak fault rocks (e.g. Imber et al. Reference Imber, Holdsworth, Butler and Lloyd1997, Reference Imber, Holdsworth, Butler and Strachan2001; Steward, Holdsworth & Strachan, Reference Stewart, Holdsworth and Strachan2000; Wibberley, Reference Wibberley, Bruhn and Burlini2005) leading to high-angle thrusting (Fig. 16b). Thermal weakening of the crust before convergence likely promotes distributed thick-skinned compressional tectonics (Fig. 16c). If the crust was thermally weakened before convergence and displays weak inherited faults (as for instance in the NPZ), basement-involved thick-skinned tectonics will expectedly occur with or without reactivation of inherited faults (Fig. 16d). Finally, if the crust has been buried and thermally weakened during convergence (as in the western Alpine ECMs), pre-existing faults are not significantly weaker than the crust, so basement-involved shortening may occur without reactivation of inherited faults (Fig. 16e).

Figure 16. Schematic rheological profiles of the continental crust illustrating possible thermal and structural weakening that may lead to thick-skinned deformation, with or without inversion tectonics.
At the time of the Laramide orogeny, the North American lithosphere was presumably cool and thick, with brittle failure prevailing down to more than 20 km depth (Fig. 11c) and limited ductile flow in the cool crust (see Section 6; Yonkee & Weil, Reference Yonkee and Weil2015). Depending on its composition – felsic or mafic granulites – the lower crust may have been either moderately weak with potential concentration of ductile flow along deep décollements or strong with potential for lithospheric buckling (Yonkee & Weil, Reference Yonkee and Weil2015). Flat-slab subduction likely changed the strength of the mantle lithosphere as a result of the competing effects of cooling that favours increased strength and stress transfer – and hydration – that decreases strength and enhances removal of mantle lithosphere. Humphreys et al. (Reference Humphreys, Hessler, Dueker, Farmer, Erslev and Atwater2003) proposed that slab de-watering under the increasingly cool conditions of slab contact with North America hydrated the base of the continental lithosphere, causing a steady regional uplift of the western US during the Laramide orogeny. In addition, magmatic ascent heated and weakened the lithosphere, which allowed horizontal shortening to occur in the mantle beneath the region of Laramide thrusting in the southern Rocky Mountains, possibly promoting crustal shortening there. In the Rocky Mountains of Wyoming, however, most of the mantle lithosphere remained strong during the Laramide orogeny over distances of several hundred kilometres (Humphreys et al. Reference Humphreys, Hessler, Dueker, Farmer, Erslev and Atwater2003), so that lithospheric shortening may have occurred at locations quite distant from the areas of crustal shortening, which implies decoupling between upper crust and mantle within the upper plate.
To conclude, basement-involved shortening in orogenic forelands occurs under specific rheological states, such as occurrence of a ductile middle or lower crust, more likely found in proximal parts of passive margins of young and hot lithosphere, hence enabling crust–mantle decoupling. Thermal inheritance from the last pre-orogenic rifting event and/or early orogenic thermal weakening of the crust/lithospheric mantle are key factors controlling deformation style. Intervening particular boundary conditions like flat-slab subduction may increase interplate coupling (see Section 9.b), hence efficient stress transmission in the far foreland, which, together with structural crustal inheritance and possible mantle weakening, may provide an explanation for diffuse intraplate basement-involved shortening in a retro-wedge setting (Laramide, Sierras Pampeanas).
9. Influence of boundary conditions and of the dynamics of orogenic wedges on the occurrence of foreland thick-skinned deformation
In addition to rheological parameters, including structural, thermal and/or compositional weakening, tectonic inversion / thick-skinned deformation occurring in the far foreland likely requires specific boundary conditions ensuring efficient transmission of stresses (crustal/lithospheric stress guide) and propagation of deformation in the pro- or retro-foreland. The nature of stress transfer leading to shortening in the upper plate far away from the plate boundary is not only highly dependent on mantle and crustal rheology, and thus on thermal state, fluids and basement rock types, but also on the degree of interplate coupling.
9.a. Influence of the geodynamic setting
The control exerted on the occurrence of thick-skinned deformation by the geodynamic setting, especially the behaviour of subduction zones (advancing, where overriding plate motion is greater than the rate of trench migration, resulting in retro-arc contraction; or retreating, where the rate of trench roll back is greater than the margin-normal absolute motion of the overriding plate, resulting in back-arc extension) has been recently discussed by Nemcok, Mora & Cosgrove (Reference Nemcok, Mora, Cosgrove, Nemcok, Mora and Cosgrove2013). When the orogens formed in the two subduction settings are considered and divided each into pro- and retro-wedge cases, one can estimate qualitatively the energy budgets involved in their development and the intensity of deformation. Accordingly, the thick-skinned regions of pro-wedges group into ‘forceful orogens’, located in orogens at advancing subduction zones (e.g. Ouachitas, Southern and western Alps), and ‘weak orogens’, located in orogens at retreating subduction zones (e.g. Apennines, Balkans, Carpathians). This distinction is not relevant for the thick-skinned regions of retro-wedges that are all located on the retro-side of the orogens developed at advancing subduction zones. Nemcok, Mora & Cosgrove (Reference Nemcok, Mora, Cosgrove, Nemcok, Mora and Cosgrove2013) concluded that an energy balance somewhat controls the presence or absence of thick-skinned domains along pro-wedges, retro-wedges and contracted intra-cratonic rift systems.
As also highlighted by Nemcok, Mora & Cosgrove (Reference Nemcok, Mora, Cosgrove, Nemcok, Mora and Cosgrove2013), unlike basement involvement documented in the western Alps that formed at an advancing subduction zone, thick-skinned tectonics occurred during the latest stages of thin-skinned tectonics in regions like the Balkans or the Carpathians (Nemcok et al. Reference Nemcok, Krzywiec, Wojtaszek, Ludhova, Klecker, Sercombe and Coward2006; Stuart et al. Reference Stuart, Nemcok, Vangelov, Higgins, Welker and Meaux2011). There, the depth range of thick-skinned tectonics is much lower than the depth of basement nappe formation in the western Alps. This may again indicate different – although still poorly understood – controls for orogens formed at retreating subduction zones compared with those formed at advancing subduction zones.
9.b. Influence of the degree of orogen-foreland / interplate coupling and transmission of compressional stresses
Tectonic inversion occurring far into the pro- or retro-foreland, or development of thick-skinned belts within cratons require both efficient transmission of orogenic stresses and accommodation of deformation. The transmission of stresses requires that the orogenic stresses are transferred to the upper plate and then transmitted far into its interior, using a crustal and/or a lithospheric stress guide. Propagation and accommodation of deformation likely requires crustal/lithospheric buckling or deep crustal décollement (e.g. Lacombe & Mouthereau, Reference Lacombe and Mouthereau1999, Reference Lacombe and Mouthereau2002; see also Section 6.a).
Regarding stress transfer, flat-slab subduction at an advancing subduction zone, possibly enhanced by increased coupling along a cratonic lithosphere root, is likely the most efficient setting as suggested by the similar settings of the Sierras Pampeanas and the Laramide uplifts.
A second controlling factor of occurrence of thick-skinned tectonics far into the foreland is the degree of coupling between the orogen and its foreland. Mechanical coupling at plate boundaries has obvious implications for transmission of stresses within the lithosphere and the resulting strain. Mechanical coupling between orogenic wedges and adjacent plates likely changes through time, for instance as lubrication of the subduction interface (due to sediment and/or fluid availability) decreases, as thermally driven softening by shear heating decreases, or even as the buoyancy of the incoming continental lithosphere that resists subduction increases (Faccenda et al. Reference Faccenda, Gerya and Chakraborty2008). Even orogens characterized by high convergence rates, such as the Rocky Mountains in Alberta, can lack basement uplifts or any kind of compressional intraplate deformation in their foreland if the foreland does not contain any major intraplate discontinuities, or if it lacks mechanical coupling with the orogen (Ziegler, Reference Ziegler, Cooper and Williams1989).
Buckling of the lithosphere is possibly an efficient way to propagate deformation into the upper plate. Weakening by either hydration or heating by magmatic ascent likely favours horizontal shortening occurring in the mantle through buckling, associated with (or causing?) thrusting in the crust above mantle lithosphere folds. Taking into account the Rocky Mountain arch wavelengths (e.g. Erslev, Reference Erslev, Kalstrom and Keller2005; Yeck et al. Reference Yeck, Sheehan, Anderson, Erslev, Miller and Siddoway2014), biharmonic-decoupled lithospheric buckling – folding of the upper crust and folding of the mantle lithosphere with different wavelengths – is more likely than monoharmonic lithospheric buckling with crust–mantle coupling (Fig. 11d). In the case that the lithosphere remains strong and rigid, crustal shortening requires a crustal décollement which at least partially decouples the uppermost crustal levels from the deeper lithosphere (Lacombe & Mouthereau, Reference Lacombe and Mouthereau1999, Reference Lacombe and Mouthereau2002; Fig. 11d). Geodynamic modelling of collision tectonics (Pfiffner, Ellis & Beaumont, Reference Pfiffner, Ellis and Beaumont2000) has shown that mid-crustal weak inclusions within the underthrusted lithosphere cause limited decoupling at the mid-crust and localize proward shear. Models of structural inversion in forelands often involve a (mid-)crustal weak zone above which basement high-angle thrusting occurs, this weak zone being possibly inherited from a previous mid-crustal extensional flat décollement (e.g. Cristallini & Ramos, Reference Cristallini and Ramos2000; Marshak, Karlstrom & Timmons, Reference Marshak, Karlstrom and Timmons2000). In the outermost Alpine foreland, a décollement in the basement probably accommodated inversion of Permo-Carboniferous grabens underlying the Mesozoic cover (Fig. 10b). A similar situation is met in the Taiwan foreland where a décollement at ~25 km depth (Fig. 8) probably accommodated offshore basin inversion and inversion of pre-existing normal faults. The recent geophysical investigations in the Bighorn Mountain region by Yeck et al. (Reference Yeck, Sheehan, Anderson, Erslev, Miller and Siddoway2014) and Worthington et al. (Reference Worthington, Miller, Erslev, Anderson, Chamberlain, Sheehan, Yeck, Harder and Siddoway2016) also suggested that mid-crustal décollement, together with fault-propagation folding, was the primary mechanism for building Laramide basement-involved foreland arches. Finally, the lower crustal imbricates imaged on the ECORS Pyrenees profile on the Spanish side (Roure et al. Reference Roure, Choukroune, Berastegui, Munoz, Villien, Matheron, Bareyt, Seguret, Camara and Deramond1989) account for a recent thickening of the Iberian crust compared to the Aquitaine crust (French side) where the lower crust is thinner and well layered, without such imbricates. This likely indicates the possible occurrence of a lateral ductile flow of the lower crust in the lower plate, allowing for long distance decoupling within the foreland and intraplate inversions away from the plate boundary (F. Roure, pers. comm, 2015). It is noticeable that thick-skinned deformation and shallow décollement tectonics may occur simultaneously (Zagros), which leads to definitely ruling out the idea that thick-skinned tectonics (only) occurs in the absence of potential shallow décollement in or at the base of the sedimentary cover.
The consequences of mechanical (de)coupling between weak orogenic wedges and strong adjacent foreland plates were investigated by means of lithospheric-scale analogue modelling (Willingshofer & Sokoutis, Reference Willingshofer and Sokoutis2009). Experimental results show that when the foreland and the orogenic wedge are decoupled, shortening is mainly taken up along the main overthrust, the decoupled boundary and within the orogenic wedge, leaving the indenter devoid of deformation. In contrast, strong mechanical coupling between the foreland and the orogenic wedge favours deformation of the lithosphere by buckling, involving both the weak zone and the strong plates. Increasing the degree of plate coupling, or the presence of decoupling horizons within the upper plate, reduces the efficiency of subduction, and convergence is accommodated partly by deformation of the upper plate (and also by thickening of the slab) (Willingshofer et al. Reference Willingshofer, Sokoutis, Luth, Beekman and Cloetingh2013). In contrast to weak plate-interface coupling, strong coupling at the plate interface leads to more complex and narrow crustal geometries in the lower plate and facilitates deformation of the upper plate. Additionally, the experiments confirm that the presence of decoupling horizons within the upper plate promotes the transfer of strain to the overriding plate and is thus key to understanding upper-plate deformation.
Summarizing, under conditions of mechanical coupling between an orogenic wedge and its foreland at crustal and/or lithospheric levels, or interplate coupling along a cratonic lithosphere root, compressional stresses can be transmitted into the foreland. Rheologically weak layers are consequently activated as décollement horizons, inducing the reactivation of pre-existing crustal discontinuities and/or broad crustal and lithosphere folding at great distances from the collision front (Ziegler, Bertotti & Cloetingh, Reference Ziegler, Bertotti and Cloetingh2002). For a given degree of coupling, the response of the foreland crust/lithosphere to the far-field stress transmission strongly depends on its thickness, thermal regime and fluid content.
9.c. Influence of the dynamics of the orogenic wedge
Occurrence of basement-involved shortening is also seemingly promoted by the intrinsic dynamics of the evolving orogenic wedge.
In the weak pro-wedges of the Carpathians and the Balkans, thick-skinned tectonics occurred during a late stage of the orogenic build-up, when the taper angle was high, the thermal regime was elevated and an extra mechanism for out-of-sequence shortening was required to further increase the orogenic taper (see Nemcok et al. Reference Nemcok, Krzywiec, Wojtaszek, Ludhova, Klecker, Sercombe and Coward2006; Stuart et al. Reference Stuart, Nemcok, Vangelov, Higgins, Welker and Meaux2011).
The Andean Eastern Cordillera, a forceful retro-wedge detached at a depth of about 30 km (Mora et al. Reference Mora, Parra, Strecker, Sobel, Hooghiemstra, Torres and Jaramillo2008, Reference Mora, Horton, Mesa, Rubiano, Ketcham, Parra, Blanco, Garcia and Stockli2010; Parra et al. Reference Parra, Mora, Jaramillo, Strecker, Sobel, Quiroz, Rueda and Torres2009 a,b), indicates that thick-skinned deformation occurs at stages when the orogenic foreland is unable to flex under the advancing orogen so that the orogen needs to build up extra strength in order to advance any further (Hermeston & Nemcok, Reference Hermeston, Nemcok, Nemcok, Mora and Cosgrove2013). Similarly, the narrow foreland plates of the Kura Valley basin, trapped between two orogens moving towards each other (Greater and Lesser Caucasus), is affected by thick-skinned deformation (Nemcok et al. Reference Nemcok, Glonti, Yukler, Marton, Nemcok, Mora and Cosgrove2013).
In the Alps–Jura system, the deformation of the Jura FTB and the Molasse basin over a basal décollement was followed by the development of new crustal imbricates and the associated out-of-sequence thrusting to regain a stable orogenic wedge geometry (Mosar, Reference Mosar1999).
In Taiwan, the thrust front seemingly propagated preferentially as a thick-skinned structure where pre-orogenic basins were present and as a thin-skinned structure where those were absent (Section 3). In the inner zones of the western FTB where topographic elevation is significant, deformation involves both thin-skinned and thick-skinned styles. According to Mouthereau & Lacombe (Reference Mouthereau and Lacombe2006), this internal deformation of the thrust wedge occurs by out-of sequence thrusting (e.g. Chelungpu–Sani thrust), possibly in order to maintain a relative balance between compressional stresses in the crust, arising from the convergence, and the topography.
It thus seems that the evolution towards a thick-skinned tectonic style of deformation may reflect in some cases a necessary step to achieve a stable (steady-state?) orogenic system after cessation or even during the latest stages of thin-skinned deformation, in response to ongoing convergence.
10. Interactions between basement-involved shortening and syntectonic sedimentation in (broken) forelands
Broken forelands are an integral part of many orogens (Hain et al. Reference Hain, Strecker, Bookhagen, Alonso, Pingel and Schmitt2011). Modern examples of broken foreland basins are parts of the Colombian Andes (e.g. Mora et al. Reference Mora, Parra, Strecker, Kammer, Dimate and Rodriguez2006, Reference Mora, Gaona, Kley, Montoya, Parra, Quiroz, Reyes and Strecker2009; Parra et al. Reference Parra, Mora, Jaramillo, Strecker, Sobel, Quiroz, Rueda and Torres2009 a,b), the Tian Shan (e.g. Sobel & Dumitru, Reference Sobel and Dumitru1997; Sobel, Hilley & Strecker, Reference Sobel, Hilley and Strecker2003; Kober et al. Reference Kober, Seib, Kley, Voigt, Nemcok, Mora and Cosgrove2013), the Qilian Shan (e.g. Tapponnier et al. Reference Tapponnier, Meyer, Avouac, Peltzer, Gaudemer, Guo, Xiang, Yin, Chen, Cai and Dai1990), the Santa Barbara system of northwestern Argentina and the Sierras Pampeanas provinces further south (e.g. Allmendinger et al. Reference Allmendinger, Ramos, Jordan, Palma and Isacks1983; Jordan & Allmendinger, Reference Jordan and Allmendinger1986). Ancient analogues of broken forelands are the Cretaceous–Eocene Laramide foreland of the western US (Dickinson & Snyder, Reference Dickinson, Snyder and Matthews1978; Jordan & Allmendinger, Reference Jordan and Allmendinger1986; Dickinson et al. Reference Dickinson, Klute, Hayes, Janecke, Lundin, McKittrick and Olivares1988; Davis et al. Reference Davis, Mulch, Carroll, Horton and Chamberlain2009; Ernst, Reference Ernst2010) and the Palaeozoic Alice Springs broken foreland in Australia (Haines, Hand & Sandiford, Reference Haines, Hand and Sandiford2001). In contrast to ‘typical’ (continuous) foreland basins associated with thin-skinned FTBs, shortening in broken forelands is accommodated by spatially disparate, diachronous basement uplifts bounded by high-angle thrusts and separated by broad basins.
10.a. Control of foreland basement faulting on syntectonic sedimentation: the Laramide broken foreland
In broken forelands, patterns of deformation, sediment routing and accumulation are different from thin-skinned FTBs (e.g. DeCelles & Giles, Reference DeCelles and Giles1996) and may be characterized by highly disparate spatio-temporal patterns of deformation and sedimentation (e.g. Hain et al. Reference Hain, Strecker, Bookhagen, Alonso, Pingel and Schmitt2011; Strecker et al. Reference Strecker, Hilley, Bookhagen, Sobel, Busby and Azor2012).
This mode of foreland basin evolution usually compartmentalizes a formerly contiguous sedimentary basin and often causes specific trends in rock uplift and sedimentation patterns. In the Rocky Mountain region, sedimentologically isolated nonmarine basins were produced by basement deformation during the Laramide orogeny within the area formerly occupied by a broad Late Cretaceous foreland basin in which laterally continuous marine facies had accumulated (Dickinson et al. Reference Dickinson, Klute, Hayes, Janecke, Lundin, McKittrick and Olivares1988). These highly localized intermontane basins are separated by strongly emergent basement-cored uplifts that tectonically fragmented the drainage system and served as local sources of coarse alluvial orogenic sediments (Dickinson, Reference Dickinson1976). Studies of synorogenic conglomerates on the flanks of Laramide basins by DeCelles et al. (Reference DeCelles, Tolson, Graham, Smith, Ingersoll, White, Schmidt, Rice, Moxon, Lemke, Handschy, Follo, Edwards, Cavazza, Caldwell and Bargar1987, Reference DeCelles, Gray, Ridgway, Cole, Srivastava, Pequera and Pivnik1991) and Hoy & Ridgeway (Reference Hoy and Ridgway1997) provided important insight into the kinematic and erosional evolution of these uplifts (Seager, Mack & Lawton, Reference Seager, Mack and Lawton1997). The presence of lacustrine facies in basin fill indicates times during which structural and topographic relief between basins and adjacent Laramide uplifts was sufficient to pond water within the depocentres.
Accommodation of deformation within basement ranges and the formation of a broken foreland may occur well inboard of the primary topographic margin of the orogen (e.g. Allmendinger et al. Reference Allmendinger, Ramos, Jordan, Palma and Isacks1983). Importantly, the uplift of such basement ranges leads to a different flexural response, hence a different evolution of associated depositional systems compared to the one expected in typical foreland basin systems related to a growing orogenic wedge (Cross, Reference Cross, Allen and Homewood1986). Because the crust is subdivided into short beams that can rotate about horizontal axes relative to one another, this rotation is a principal component of tectonic subsidence in addition to flexural loading. Laramide basin subsidence is thus related to the flexural effects of tectonic loads imposed on the lithosphere by thrust masses emplaced along the flanks of adjacent uplifts (Hagen, Shuster & Furlong, Reference Hagen, Shuster and Furlong1985). For instance, the depocentre of the Upper Cretaceous in eastern Wyoming is presumably too far from the thrust belt to have been simply caused by loading and flexure and was likely controlled by Laramide basement uplifting.
The structural, geomorphic and depositional characteristics and dynamics of broken foreland basins on the eastern flanks of the central Andes in Bolivia and NW Argentina have been recently discussed by Strecker et al. (Reference Strecker, Cerveny, Bloom and Malizia1989, Reference Strecker, Hilley, Bookhagen, Sobel, Busby and Azor2012), Sobel & Strecker (Reference Sobel and Strecker2003), Hain et al. (Reference Hain, Strecker, Bookhagen, Alonso, Pingel and Schmitt2011), Iaffa et al. (Reference Iaffa, Sabat, Munoz, Carrera, Nemcok, Mora and Cosgrove2013) and Davila & Carter (Reference Davila and Carter2013). An important point is that the along-strike variations in the geometry of foreland basins are likely generated by the variability in the structure of the Andes, structural inheritance (e.g. Mescua et al. Reference Mescua, Giambiagi, Tassara, Gimenez and Ramos2014) and/or the variable strength of the upper-plate lithosphere.
10.b. Control of syntectonic sedimentation on foreland basement-involved deformation
Based on numerical modelling, Erdos, Huismans & van der Beek (Reference Erdos, Huismans and van der Beek2015) recently suggested an additional factor that may control the occurrence of basement-involved shortening in forelands. The basic idea is that the minimum-work principle applies to basement thrusting, i.e. a new crustal thrust should occur at the locus where the total work required for slip on the viscous mid-crustal décollement and breakthrough to the surface is minimized. The main effect of syntectonic sedimentation is to increase the frictional work required to create a new thick-skinned crustal-scale thrust. Consequently, the formation of a new thrust is favoured where sediments taper out.
Numerical modelling supports that sediment loading on the external parts of an orogen may provide a first-order control on its crustal-scale structural style and significantly changes its dynamics. In sediment-starved orogens, basement deformation is mostly limited to the axial orogenic zone with thin-skinned deformation in the adjacent foreland, whereas in sediment-loaded orogens, thick-skinned crustal-scale deformation with long basement thrust sheets occurs in both the Axial Zone and beneath the foreland (Erdos, Huismans & van der Beek, Reference Erdos, Huismans and van der Beek2015). Erosion, while strongly affecting the width of the orogenic core, appears to have limited direct effects on the evolution of the foreland.
The effect of synorogenic/syntectonic sedimentation on basement involvement in shortening in orogenic forelands as predicted by numerical models can be illustrated by natural field examples. The Ural Mountains (central Russia) is a collisional belt formed during Late Devonian to Late Permian – Early Triassic times (Brown et al. Reference Brown, Alvarez-Marron, Perez-Estaun, Gorozhanina, Baryshev and Puchkov1997). The kinematic evolution consists of two forward-propagating thrust sequences, with imbricates of Precambrian basement and Palaeozoic cover; the amount of shortening is small and the basement thrust sheets show a spacing of about 10 km (Brown et al. Reference Brown, Alvarez-Marron, Perez-Estaun, Gorozhanina, Baryshev and Puchkov1997, Reference Brown, Alvarez-Marron, Perez-Estaun, Puchkov and Ayala1999). Overall, the Urals show little or no basement deformation below the foreland, and the whole orogen is relatively narrow as a result of basement deformation being restricted to the central orogenic core. These characteristics are those predicted by numerical models (Erdos, Huismans & van der Beek, Reference Erdos, Huismans and van der Beek2015) for a very limited thickness of syntectonic deposits, which is the case for the sediment-starved Ural orogen. In contrast, the Swiss Alps along the Jura section (Fig. 10a, b) display long basement thrust sheets (up to ~50 km) (Fig. 10b) below thick foreland basin deposits, up to 4 km (Burkhard & Sommaruga, Reference Burkhard, Sommaruga, Mascle, Puigdefabregas, Luterbacher and Fernandez1998) and possibly up to 8 km even now partly eroded (Bonnet, Malavieille & Mosar, Reference Bonnet, Malavieille and Mosar2007). In agreement with numerical models, the Swiss Alps orogenic wedge is larger than the Urals as a result of thick-skinned basement deformation below the foreland.
To conclude, although the long basement thrust sheets as documented in numerical models are likely different from localized basement uplifts of broken forelands, one can nevertheless expect a mutual control between thick-skinned deformation in FTBs and sediment deposition leading to burial in forelands: basement thrusts beneath the foreland are favoured in the case of sediment-loaded orogens, and in turn basement thrusts strongly influence the local pattern of uplift, erosion and sedimentation.
11. Sequence of deformation and relationships between thick-skinned and thin-skinned tectonic styles in space and time
In this section, we only attempt to discuss the sequence of thick-skinned deformation and its relationships in space and time with thin-skinned deformation in foreland FTBs, irrespective of the absolute timing of thick-skinned deformation in the reviewed orogens.
On the basis of the examples reviewed here, we state that different spatial and temporal relationships between the two tectonic styles are encountered, preventing formulation of any simple rule on their occurrence.
Case 1: Early inversion of inherited normal faults in the foreland leading to high-angle basement thrusting prior to thin-skinned deformation (Fig. 17a): In the Zagros and in Taiwan for instance, early compressional/transpressional inversion of pre-existing normal faults of the Arabian and southeastern Chinese passive margins, respectively, occurred far in the foreland before frontal thin-skinned thrusts were activated (Lacombe & Mouthereau, Reference Lacombe and Mouthereau2002; Lacombe et al. Reference Lacombe, Mouthereau, Angelier, Chu and Lee2003; Ahmadhadi, Lacombe & Daniel, Reference Ahmadhadi, Lacombe, Daniel, Lacombe, Lavé, Vergés and Roure2007). These early movements along steeply dipping faults, however, accommodated a small amount of shortening.

Figure 17. Different spatial and temporal relationships/sequences between thin-skinned and thick-skinned tectonics in FTBs.
Case 2: Early basement shortening, then activation of outer thin-skinned deformation coeval with exhumation and forelandward transport of shortened basement (Fig. 17b): In the western Alps, at the latitude of Oisans for instance, basement-involved shortening occurred in the buried basement of the innermost external zone before thin-skinned deformation initiated in the frontal thin-skinned Vercors FTB (Fig. 17b). As discussed in Section 4.a, the later activation of the shallow décollement is related to thrusting along a localized frontal ramp that coevally transported westwards and exhumed the basement of the ECMs (Bellahsen et al. Reference Bellahsen, Mouthereau, Boutoux, Bellanger, Lacombe, Jolivet and Rolland2014).
Case 3: Mainly coeval thin-skinned and thick-skinned deformation (Fig. 17c): There is to date a consensus that thin-skinned and thick-skinned styles of deformation are superimposed (as attested by seismicity) and occurred roughly coevally in the Zagros (Fars Province) (e.g. Oveisi et al. Reference Oveisi, Lave, van der Beek, Carcaillet, Benedetti and Aubourg2009; Mouthereau, Lacombe & Verges, Reference Mouthereau, Lacombe and Verges2012), even though early basement faults may have been activated before cover folding (Fig. 17a) (e.g. Ahmadhadi, Lacombe & Daniel, Reference Ahmadhadi, Lacombe, Daniel, Lacombe, Lavé, Vergés and Roure2007) or late basement thrusts may offset thin-skinned structures in places (Molinaro et al. Reference Molinaro, Leturmy, Guezou, Frizon De Lamotte and Eshraghi2005; Sherkati, Letouzey & Frizon de Lamotte, Reference Sherkati, Letouzey and Frizon de Lamotte2006). The cover is detached mainly above the low-viscosity Hormuz salt layer while the basement deforms by both seismogenic faulting and ductile aseismic shearing. As mentioned earlier, that thick-skinned deformation and shallow décollement tectonics may occur simultaneously leads to definitely ruling out the idea that thick-skinned tectonics (only) occurs in the absence of potential shallow décollement in or at the base of the sedimentary cover.
Case 4: Late (out-of-sequence) basement thrusting (Fig. 17d): In the Jura, thick-skinned thrusting related to reactivation of inherited Palaeozoic basins caused refolding of shallow thin-skinned nappes and present-day surface uplift. The same possibly occurs in the eastern Precordillera of western Argentina (e.g. Meigs et al. Reference Meigs, Krugh, Schiffman, Vergés and Ramos2006). In Taiwan, out-of-sequence seismogenic basement faulting may occur below or across thin-skinned nappes.
Case 5: Late thick-skinned deformation forelandward (Fig. 17e): In the US Rockies, thick-skinned shortening gave rise to the Laramide arches in the foreland of the thin-skinned Sevier FTB. Thick-skinned Laramide deformation occurred partly coevally with, and after, Sevier deformation. A similar setting is currently encountered in the active Sierras Pampeanas in the foreland of the Precordillera FTB of Argentina.
Inversion tectonics and basement-involved shortening may therefore occur at different stages of the tectonic evolution of FTBs, prior to thin-skinned deformation through inversion of inherited basement faults in the foreland, coevally with thin-skinned deformation or after thin-skinned tectonics. While thin-skinned systems usually form an orogenic wedge that propagates/accretes progressively outwards through time in a more or less continuous and regular fashion, thick-skinned systems often display a more irregular and erratic sequence (e.g. Laramide, Sierras Pampeanas), possibly linked to the heterogeneous and complex transmission of orogenic stress and accommodation of shortening through the crystalline basement with inherited weaknesses and anisotropies.
12. Conclusions
FTBs mainly form either in lower and upper plates at the expense of proximal parts of former passive margins or intracontinental rifts during collision or within the upper plate of flat-slab subduction orogens. In contrast, inner parts of mountain belts are likely made of stacked units from the distal passive margin domains that have undergone continental subduction and high-pressure – low-temperature metamorphism.
There are increasing lines of evidence of basement-involved shortening in FTBs, even in the ‘archetypal’ thin-skinned belts. This basement involvement is often associated with basement inversion tectonics. Our review illustrates how the pre-orogenic deformation of the basement may control the geometry, kinematics and mechanics of FTBs, either at the scale of the whole belt (e.g. belt curvature, segmentation and along-strike variations of structural styles, sequence of deformation, localization of contractional deformation and percentage of shortening) or at the scale of tectonic units (reactivation of inherited basement faults, basement-cored folding). In some cases, however, inherited basement (normal) faults are not reactivated whereas newly formed compressional shear zones develop, which brings into question the bulk rheology of the crust versus the rheology of pre-existing fault zones available for reactivation.
In basement-involved, thick-skinned FTBs, shortening is distributed throughout the whole crust and is usually lower than in their thin-skinned counterparts, which likely requires/reflects a specific thermo-mechanical behaviour of the underlying lithosphere (e.g. hot and young, hence weak). In FTBs resulting from inversion of former proximal passive margins, basement thrusting that occurs in a rather localized way in their inner parts requires structural inheritance and/or a hot crustal temperature either inherited from a recent (pre-orogenic) rifting event or resulting from synorogenic underthrusting and heating.
Basement involvement in FTBs raises the question of the way the orogen is mechanically coupled to the foreland and how orogenic stresses are transmitted through the heterogeneous basement of the foreland/plate interior. Development of thick-skinned belts within cratons remains somewhat enigmatic and likely requires specific boundary conditions (strong interplate coupling, such as provided by flat-slab subduction) ensuring efficient transmission of stresses (crustal/lithospheric stress guide) and propagation of deformation in the pro- and retro-foreland by crustal/lithospheric buckling or deep crustal décollement, in addition to local structural and/or possible physical/compositional weakening.
Whatever its control(s) (structural inheritance, thermal state, composition), the rheology of the continental lithosphere appears to be central to understanding mountain-building processes, and understanding the wide range in structural styles of FTBs and their relationships to crustal/lithospheric architecture/rheology will still remain a key area of research for years.
Acknowledgements
The authors would like to warmly thank P. Ryan, F. Roure and D. Brown for their constructive comments that greatly improved the manuscript, R. Chuang and K. Johnson for kindly providing the original figure on the Nantou fault, G. Camanni and D. Brown for kindly providing the original figure on the Shuilikeng fault and V. Scisciani for kindly providing the original figure on structural styles in the Umbria–Marches.