Introduction
Tourmaline is a supergroup of minerals with general formula XY3Z6[T6O18](BO3)3V3W, with primary occupancy: X = Na+, Ca2+, K+ and □ [vacancy]; Y = Fe2+, Li+, Al3+, Mg2+, Zn2+, Cr3+, Ti4+, V3+ and Fe3+; Z = Al3+, Fe3+, Mg2+, Cr3+ and V3+; T = Si4+, Al3+ and B3+; B = B3+; V = OH– and O2–; W = OH–, F– and O2–. On the basis of the dominant X-site occupancy, tourmaline can be dived into the alkali group (Na), calcic group (Ca) and X-vacant group (□). Each group is further divided, depending on the dominant occupancy of the W-site, into fluor-, hydroxyl- and oxy-subgroups (Henry et al., Reference Henry, Novák, Hawthorne, Ertl, Dutrow, Uher and Pezzotta2011).
Oxy-dravite is an oxy-species, with oxygen as the dominant anion in the W-site, from the sodic group of the tourmaline supergroup of minerals (Henry et al., Reference Henry, Novák, Hawthorne, Ertl, Dutrow, Uher and Pezzotta2011). Since its original description (Bosi and Skogby, Reference Bosi and Skogby2013) from the quartz–muscovite schist from Osarara, Narok district, Kenya, the mineral has been found at several localities worldwide, typically in metamorphic environments, and typically in solid solution with other species (e.g. Čopjaková et al., Reference Čopjaková, Škoda and Vašinová-Galiová2012; Cempírek et al., Reference Cempírek, Houzar, Novák, Groat, Selway and Šrein2013; Pieczka et al., Reference Pieczka, Ertl, Sek, Twardak, Zelek, Szeleg and Giester2018).
Although oxy-tourmalines are relatively new among the tourmaline species (e.g. Bosi and Skogby, Reference Bosi and Skogby2013), their petrogenetic importance is significant. The tourmaline-supergroup minerals are widely regarded as a mineral that records a signature of the conditions and chemistry of its environment and, due to its high stability, preserves this signature (e.g. Henry and Dutrow, Reference Henry, Dutrow, Anovitz and Grew1996). In primitive pegmatites, oxy-tourmaline shows distinct evolution of W-site occupancy between hydroxy- and oxy-species (Novák et al., Reference Novák, Povondra and Selway2004). In marbles and calc-silicate rocks, oxy-tourmaline is shown to be a good monitor of fluctuations of fluid composition (Gadas et al., Reference Gadas, Novák, Cempírek, Filip, Galiová, Groat and Všianský2014; Bosi et al., Reference Bosi, Skogby, Ciriotti, Gadas, Novák, Cempírek, Všianský and Filip2017b; Krmíček et al., Reference Krmíček, Novák, Trumbull, Cempírek and Houzar2021; Scribner et al., Reference Scribner, Cempírek, Groat, Evans, Biagioni, Bosi, Dini, Hålenius, Orlandi and Pasero2021). Prior to its formal description, oxy-dravite was shown to be an indicator of metaevaporites (e.g. Henry et al., Reference Henry, Sun, Slack and Dutrow2008). In graphitic rocks, oxy-tourmaline records metamorphic reactions via episodic accumulation of Ti, Cr and V during its growth (Bosi et al., Reference Bosi, Reznitskii and Sklyarov2013; Cempírek et al., Reference Cempírek, Houzar, Novák, Groat, Selway and Šrein2013; Bosi et al., Reference Bosi, Reznitskii, Hålenius and Skogby2017a), and in ore systems, their increase of Fe3+ contents may indicate fluid boiling and metal discharge (Duchoslav et al., Reference Duchoslav, Marks, Drost, McCammon, Marschall, Wenzel and Markl2017; Drivenes Reference Drivenes2022).
In this paper, we report the occurrence, and physical and structural properties of oxy-dravite with a near-end-member composition. These data improve characterisation of physical properties of oxy-dravite, allow examination of oxy-dravite solid solutions with respect to other species, improve the interpretation of Al–Mg disorder among the Y- and Z-sites, and discuss its petrogenetic importance.
Occurrence and paragenesis of oxy-dravite in the Beluga occurrence
The Beluga property is located in the southern part of Baffin Island, Nunavut territory, Canada (Fig. 1). The locality is known chiefly due to the occurrence of sapphire-bearing calc-silicate pods. These pods are hosted in a shelf sequence of clastic rocks and carbonates along with garnet-bearing metapsammite, metapelite, metasemipelite and rarely orthoquartzite of the Lake Harbour Group, a Paleoproterozoic supracrustal suite (Scott, Reference Scott1997; Lepage and Rohtert, Reference Lepage and Rohtert2006; Corrigan et al., Reference Corrigan, Pehrsson, Wodicka and de Kemp2009; Belley et al., Reference Belley, Dzikowski, Fagan, Cempírek, Groat, Mortensen, Fayek, Giuliani, Fallick and Gertzbein2017; Belley and Groat, Reference Belley and Groat2020). The Lake Harbour Group rocks, considered as a cover sequence of the Meta Incognita microcontinent, were transformed by several stages of metamorphism (granulite and amphibolite facies, 810°C/8 kbar and 720°C/8 kbar, respectively) during convergent orogenesis (initiated ca. 1849–1835 Ma) in the Trans-Hudson orogeny (St-Onge et al., Reference St-Onge, Wodicka and Ijewliw2007). High-grade metamorphism was followed by final, localised retrograde overprints (ca. 1797–1785 Ma; St-Onge et al., Reference St-Onge, Wodicka and Ijewliw2007). The area has diverse geology and is an excellent source of gemstones; sapphires, spinel (Belley and Groat, Reference Belley and Groat2019) and lapis lazuli (Hogarth, Reference Hogarth1971) occur in gem quality specimens.

Figure 1. Geology of southern Baffin Island; the Beluga occurrence is located in the Kimmirut area (3), after Belley and Groat (Reference Belley and Groat2020).
At Beluga, uncommon oxy-dravite occurs as dark brown, equant to short-prismatic, idiomorphic crystals with maximal dimensions of ca. 4 × 3 cm (Fig. 2) in the retrograde albite–muscovite–corundum–calcite domains in the calc-silicate rock (Belley et al., Reference Belley, Dzikowski, Fagan, Cempírek, Groat, Mortensen, Fayek, Giuliani, Fallick and Gertzbein2017). Belley et al. (Reference Belley, Dzikowski, Fagan, Cempírek, Groat, Mortensen, Fayek, Giuliani, Fallick and Gertzbein2017) did not have sufficient textural evidence to clearly establish the associated stable mineral assemblage for oxy-dravite (i.e. either the prograde/peak metamorphic Di + Ne, high-temperature retrograde Phl + Pl + Scp, or the retrograde corundum-bearing assemblage; see Belley et al., Reference Belley, Dzikowski, Fagan, Cempírek, Groat, Mortensen, Fayek, Giuliani, Fallick and Gertzbein2017 for details). However, additional field work at Beluga by Belley (2022 field season, unpublished) revealed the persistent, and seemingly exclusive, association of oxy-dravite with the retrograde corundum-bearing assemblage (albite–calcite–muscovite–corundum–graphite with accessory pyrrhotite). Oxy-dravite crystals observed in contact with corundum occur on the latter mineral's surface, conforming to the euhedral shape of corundum, and have not been observed as inclusions in corundum. Examination of 55 corundum crystals (Belley unpublished data, 2023) revealed common inclusions of calcite, three inclusions of phlogopite (partly altered to muscovite–amesite symplectites with subordinate V-bearing TiO2) and one inclusion of scapolite. These inclusions may indicate that, at least in the early stages of mineralisation, corundum formed a stable assemblage with calcite–phlogopite–scapolite – forming earlier than previously estimated by Belley et al. (Reference Belley, Dzikowski, Fagan, Cempírek, Groat, Mortensen, Fayek, Giuliani, Fallick and Gertzbein2017). Euhedral muscovite crystals occur on the surface of some corundum crystals and within fractures, thus it may not form a stable assemblage with the corundum. The textural relationships between corundum and oxy-dravite suggest that oxy-dravite probably formed simultaneously with, or later than, corundum.
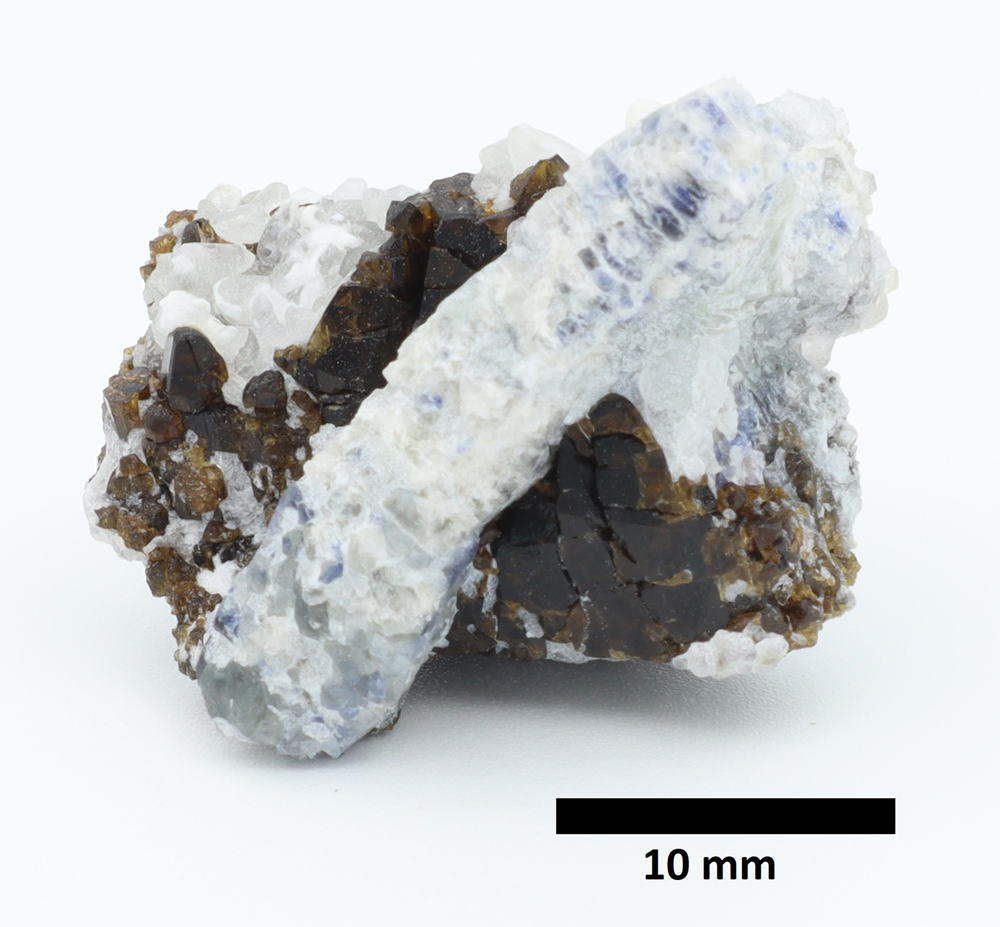
Figure 2. Photo of the sample containing the brown Beluga oxy-dravite in association with a prismatic light blue corundum crystal and late euhedral calcite (sample from collection of P. Belley).
The Beluga calc-silicate rock protolith is interpreted to be dolomitic marl (Belley et al., Reference Belley, Dzikowski, Fagan, Cempírek, Groat, Mortensen, Fayek, Giuliani, Fallick and Gertzbein2017; Belley and Groat, Reference Belley and Groat2019). Though the boron isotopic signature of the oxy-dravite is consistent with a marine boron source, this does not necessarily indicate that the Beluga rock consists of meta-evaporites. Evaporite-associated minor and trace elements, such as Mg, Mg/Ca ratio, B, B/Al ratio, Li, F and Cl, were not in concentrations sufficiently elevated as to indicate a definitive evaporitic signature (Belley et al., Reference Belley, Dzikowski, Fagan, Cempírek, Groat, Mortensen, Fayek, Giuliani, Fallick and Gertzbein2017).
Experimental methods
The sample, a polished section of a crystal (ca. 6 mm in diameter) oriented perpendicular to the c axis, was analysed using a fully automated CAMECA SX-100 electron microprobe (EMP) in the Electron Microprobe Laboratory of the Department of Geological Sciences, Masaryk University (joint workplace of the Faculty of Science of Masaryk University and the Czech Geological Survey). The tourmalines were analysed in wavelength-dispersive mode, with accelerating voltage of 15 kV, beam current of 10 nA, and spot size of 5 μm. The following standards were used for measurement of major and minor elements in the tourmalines on Kα X-ray lines: sanidine (Si, Al, K); albite (Na); pyrope (Mg); titanite (Ti); chromite (Cr); vanadinite (Cl); fluorapatite (P); wollastonite (Ca); almandine (Fe); spessartine (Mn); ScVO4 (V); gahnite (Zn); and topaz (F). Average detection limits and 1σ for individual elements are, respectively (in wt.%): 0.043/0.201 (Na); 0.041/0.334 (Si); 0.035/0.424 (Al); 0.024/0.124 (Mg); 0.031/0.038 (Ti); 0.027/0.022 (Cr); 0.017/0.015 (Cl); 0.033/0.027 (P); 0.037/0.055 (Ca); 0.024/0.022 (K); 0.068/0.125 (Fe); 0.063/0.053 (Mn); 0.048/0.043 (V); 0.093/0.079 (Zn); and 0.053/0.05 (F). The Al/Si ratio was verified on a kyanite secondary standard.
The tourmaline formulae were calculated on the basis of T + Z + Y = 15 atoms per formula unit (apfu), assuming B = 3 apfu, full occupancy and Li at the Y site, zero [4]B at the T site, and OH = (4 – V,WO – F) (Henry et al., Reference Henry, Novák, Hawthorne, Ertl, Dutrow, Uher and Pezzotta2011). All Fe was assumed to be divalent which is in agreement with bond-valence calculations. The assumption regarding Li is supported by the negligible Li contents detected by laser ablation inductively coupled plasma mass spectroscopy (LA-ICP-MS) analysis (<13 ppm). The tetrahedral bond length <T–O> ≈ 1.621 Å indicates full occupancy by Si or trace [4]Al at the T-site.
Trace-element analyses (Li, Be, B, P, Sc, Ti, V, Cr, Co, Ni, Cu, Zn, Ga, Y, Zr, REE, Th and U) were performed by LA-ICP-MS analysis at the Laboratory of Atomic Spectrochemistry of the Department of Chemistry (Faculty of Science, Masaryk University). The LA-ICP-MS equipment consists of an Analyte G2 (Teledyne CETAC Technologies) connected to a sector field ICP-MS spectrometer Element 2 (Thermo Fischer Scientific). An ArF* laser ablation device equipped with a HelEx II sample cell was operated at a wavelength of 193 nm. The ablated material was carried by He flow (0.65 L/min) and mixed with Ar (~1 L/min) prior to entering the IC-PMS spectrometer. Optimisation of LA-ICP-MS parameters was performed with the glass reference material NIST SRM 610 with respect to the maximum signal-to-background ratio and 248ThO+/232Th+ < 1. All element contents were normalised using Si determined from electron microprobe analysis (EMPA) as an internal standard. Data were processed using an in-house programmed optimisation spreadsheet in MS Excel. Analytical results and limits of detection (LOD) are reported in Table 1.
Table 1. Selected representative chemical compositions of the Beluga oxy-dravite (rim to core profile across a large crystal using EMPA and LA-ICP-MS, and the EMPA composition of the crystal used for SC-XRD).

Note: b.d.l – below detection limit; LOD – limits of element detection for LA-ICP-MS data; SC-XRD – single-crystal X-ray diffraction.
* calculated from stoichiometry, see Methods.
The crystal structure of an oxy-dravite fragment extracted from the rim of the studied crystal was determined with a Bruker X8 APEX II diffractometer with graphite-monochromated MoKα radiation at C-HORSE (the Centre for Higher Order Structure Elucidation, in the Department of Chemistry at University of British Columbia). The crystal-to-detector distance was 40 mm. The data were collected at room temperature in a series of φ and ω scans in 0.50° oscillations with 10 s exposures (see Table 2). Data collection and integration was done using the Bruker SAINT software package (Bruker, Reference Bruker2007). Data were corrected for absorption effects using the multi-scan technique (SADABS, Sheldrick, Reference Sheldrick2008) and also for Lorentz and polarisation effects.
Table 2. Crystal data, data collection and refinement parameters for near end-member oxy-dravite from Beluga.

Polarised single-crystal Raman spectra of the Beluga oxy-dravite (rim of the polished section used for EMPA) were collected at room temperature using a Horiba LabRAM–HR spectrometer equipped with an Olympus BX41 optical microscope. The Raman signal was collected in the range of 100–4000 cm−1 using grating of 600 gr/mm and a 50× objective from four 60 s accumulations. The system was operated in the confocal mode with a beam diameter of ~1 μm. No visual damage to the analysed surface was observed after the excitation. Raman shift calibration was done using a Si wafer. The wavenumber accuracy was ~0.5 cm−1 and the spectral resolution was ~2 cm−1. Band fitting was completed after appropriate background correction, assuming combined Lorentzian-Gaussian band shapes using the Voight function.
Chemical composition
The tourmaline sample is weakly zoned, with only minor changes from the crystal core to its rims (Fig. 3; Table 2). The X-site is occupied predominantly by Na (0.87 to 0.91 apfu), its Ca content varies from 0.03 to 0.12 apfu, and vacancy contents are negligible (<0.06 pfu). The V- and W-sites are characterised by high OH (2.92–3.00 apfu) and O contents (0.80–0.95 apfu), with minor F (0.12–0.17 apfu) only.

Figure 3. Composition of the Beluga oxy-dravite in several compositional spaces compared to compositions from other studies (Ertl et al., Reference Ertl, Hughes, Brandstätter, Dyar and Prasad2003; Čopjaková et al., Reference Čopjaková, Škoda and Vašinová-Galiová2012; Bosi and Skogby, Reference Bosi and Skogby2013; Pieczka et al., Reference Pieczka, Ertl, Sek, Twardak, Zelek, Szeleg and Giester2018; Sȩk et al., Reference Sȩk, Włodek, Stachowitz, Woźniak and Pieczka2022). Note the high miscibility with vacancy-, Fe- and OH-dominated species.
The octahedral Y- and Z-sites contain dominant Al (6.87–7.31 apfu) and contain high Mg (1.73–1.98 apfu), and low contents of Fe2+ (0.13–0.18 apfu); Li is negligible (<13 ppm based on LA-ICP-MS). The tetrahedral site is occupied mostly by Si (5.73–5.95 apfu) with minor substituted Al (0.06–0.27 apfu).
Amounts of trace elements determined by LA-ICP-MS analyses are generally very low (Table 1 – EMPA and LA-ICP-MS). The only exceptions are Ti and V with maximum values up to 4495 ppm (up to 0.75 wt.% TiO2) and 312 ppm (0.05 wt.% V2O3), respectively; they show a slight decrease from the crystal rims towards the core. Contents of Sc, P, Li, Cr, Mn, Co, Ni, Ga and Zn are close to their detection limits and approach tens of ppm in maximum values. The amounts of REE, Be, Cu and Zr are below their detection limits.
Crystal structure and bond valence refinement
The crystal structure of the oxy-dravite sample was refined from single-crystal X-ray diffraction data. The refinements were performed using the SHELXTL crystallographic software package (Sheldrick, Reference Sheldrick2008, Reference Sheldrick2015) of Bruker AXS. Scattering factors for neutral atoms were employed for the cations and ionic factors for O2– were used for oxygen (Hovestreydt, Reference Hovestreydt1983). The structure of dravite after Foit and Rosenberg (Reference Foit and Rosenberg1979) was introduced as an initial model for refinement that was processed (by refining occupancy of Na at the X-site, and Fe vs. Al at the Y- and Z-sites) to a final R index of ~1.45% for an anisotropic displacement model. The H3-atom site was located in residual electron-density maps whereas the H1 site was impossible to locate. The H3 site isotropic displacement parameter was constrained to be equal to 1.2 times of that of the O3 site; its distance from the donor oxygen atom was constrained to 0.92(2) Å.
The mean <Z–O> bond length of 1.928 Å indicates that the Z-site is not fully occupied by Al (ideally <ZAl–O> = 1.906 Å; Bosi and Andreozzi, Reference Bosi and Andreozzi2013) but partially replaced by Mg, suggesting Y,Z(Al–Mg) disorder. Occupancies of octahedral sites were refined using bond-valence optimisation (Table 3) which confirmed disorder of Mg and Al between the Y- and Z-sites, typical for Mg-rich tourmalines (e.g. Grice and Ercit, Reference Grice and Ercit1993; Hawthorne et al., Reference Hawthorne, MacDonald and Burns1993; Ertl et al., Reference Ertl, Hughes, Brandstätter, Dyar and Prasad2003; Bosi and Lucchesi, Reference Bosi and Lucchesi2004; Bosi and Skogby, Reference Bosi and Skogby2013; Bačík, Reference Bačík2015; Pieczka et al., Reference Pieczka, Ertl, Sek, Twardak, Zelek, Szeleg and Giester2018; Scribner et al., Reference Scribner, Cempírek, Groat, Evans, Biagioni, Bosi, Dini, Hålenius, Orlandi and Pasero2021; Bosi et al., Reference Bosi, Biagioni, Pezzotta, Skogby, Hålenius, Cempírek, Hawthorne, Lussier, Abdu, Day, Fayek, Clark, Grice and Henry2022; Sȩk et al., Reference Sȩk, Włodek, Stachowitz, Woźniak and Pieczka2022).
Table 3. Fractional atomic coordinates, isotropic/equivalent isotropic displacement parameters (Å2), and anisotropic atomic displacement parameters (Å2).

The occupancy of the T-site is dominated by Si but its content varies: 5.73–5.95 apfu. With the elongated <T–O> bond length, it is evident that some amount of Al3+ (or, less likely, a combination of Al3+ + B3+) is present in the T-site (e.g. Grice and Ercit, Reference Grice and Ercit1993; MacDonald and Hawthorne, Reference MacDonald and Hawthorne1995; Hawthorne, Reference Hawthorne2002; Ertl et al., Reference Ertl, Henry and Tillmanns2018). The final empirical structural formula, with hydrogen distribution adjusted between the V- and W-sites, using the formula of Bosi (Reference Bosi2013), is:

For the final step of the structure refinement, atom fractions from the final empirical formula were fixed to the individual sites; final bond lengths (rare changes on third decimal place) were used again for the bond-valence optimisation (using values R 0 and B from Gagné and Hawthorne, Reference Gagné and Hawthorne2015) which resulted in results identical to the previous step. Final atomic parameters and refined scattering values expressed as site occupancies are given in Table 4 and selected interatomic distances in Table 5. The crystallographic information file has been deposited with the Principal Editor of Mineralogical Magazine and is available as Supplementary material (see below).
Table 4. Bond distances of the crystal studied (Å).*

* Symmetry codes: (iv) –y+⅔, x–y+⅓, z–⅔; (v) –x+y+⅓, –x+⅔, z–⅓
Table 5. Empirical weighted bond valences (in valence units) and other structural site parameters for the Beluga oxy-dravite.*

* Note: obs. = observed; cal. = calculated. §Includes 0.99 valence units contribution from H3.
Physical properties
The Beluga oxy-dravite is strongly pleochroic, light yellowish-brown colour in the omega direction and colourless in the epsilon direction. The Beluga oxy-dravite is uniaxial (–) with ω = 1.6453(5) and ɛ = 1.6074(18), measured using a low pressure Na-vapour lamp. The density calculated using the observed cell volume and the empirical composition is 3.069 g.cm–3. The compatibility index reflecting the difference between the physical (K p) and the chemical specific-refractivity (Mandarino, Reference Mandarino1981) is 0.016, corresponding to the superior category. A comparison of the Beluga oxy-dravite optical properties with those for other tourmaline Mg-bearing end-members is shown in Table 6.
Table 6. The comparison of oxy-dravite optical properties with other tourmaline Mg-bearing end-members.

Raman spectra
The Raman spectrum of the Beluga oxy-dravite (Fig. 4) was (after background correction) fitted using Pseudo-Voight peaks. The spectrum pattern generally corresponds well to that of oxy-dravite and other Mg-bearing tourmalines (Watenphul et al., Reference Watenphul, Burgdorf, Schlüter, Horn, Malcherek and Mihailova2016a, Reference Watenphul, Schlüter, Bosi, Skogby, Malcherek and Mihailova2016b; Watenphul et al., Reference Watenphul, Malcherek, Wilke, Schlüter and Mihailova2017). According to the equations provided by Watenphul et al. (Reference Watenphul, Schlüter, Bosi, Skogby, Malcherek and Mihailova2016b), there is a dependence of measured ω(ZO6) (ω = Raman shift) on the content of non-aluminium cations (Mg and Fe3+) at the Z-site. The calculated values for the Beluga oxy-dravite are 0.98(19) apfu ZMg and 0 apfu ZFe3+; the error of the calculated ZMg value overlaps with the error of the structurally refined ZMg value (0.66±0.30 apfu). Despite the large difference, the result shows that the Raman calculation above is a valid tool for estimation of Y,ZMg disorder.

Figure 4. Polarised Raman spectra (black) and the main fitted bands (grey) for the Beluga oxy-dravite (orientation || c).
The Beluga oxy-dravite Raman peaks related to OH at the W-site (range 3711–3772 cm–1) generally agree with data described by Watenphul et al. (Reference Watenphul, Burgdorf, Schlüter, Horn, Malcherek and Mihailova2016a). The most intense peak at 3739 cm–1 is related to the YMgYMgYAl–XNa configuration of atoms around the O1 atom whereas the peak at 3772 cm–1 matches values reported for the YMgYMgYMg–XNa configuration in dravite and uvite (Watenphul et al., Reference Watenphul, Burgdorf, Schlüter, Horn, Malcherek and Mihailova2016a). For the peak at 3711 cm–1 we propose the configuration YMgYAlYAl–XNa as it must be present in a significant amount due to the Mg–Al disorder between the Y- and Z-sites revealed by the structural refinement (below). The presence of peaks related to WOH indicates that the VOH must be present in a significant amount. In the disorder calculation (Bosi, Reference Bosi2013), WOH is negatively correlated to F content; the Raman results may suggest slight fluorine overestimation, possibly due to use of topaz as an EMPA standard (Ottolini et al., Reference Ottolini, Cámara and Bigi2000).
By comparison with Raman spectra of dravite (Watenphul et al., Reference Watenphul, Burgdorf, Schlüter, Horn, Malcherek and Mihailova2016a), the two most intense VOH-peaks of the Beluga oxy-dravite at 3531 and 3574 cm–1 (Fig. 4) probably correspond to the 2YMgZAlZAl–YAlZAlZAl, and 3YMgZAlZAl configurations, respectively. The middle peak at 3556 cm–1 is closest to the 2YMgZMgZAl–YMgZAlZAl configuration (located at 3547 cm–1 in uvite; Watenphul et al., Reference Watenphul, Burgdorf, Schlüter, Horn, Malcherek and Mihailova2016a) which corresponds with Mg–Al disorder between the Y- and Z-sites. Peaks at similar wavenumbers (3548–3560 cm–1) were observed by Fantini et al. (Reference Fantini, Tavares, Krambrock, Moreira and Righi2014) in dravite, magnesio-foitite and uvite.
Discussion
Comparison of reported oxy-dravite compositions and structures
Tourmaline with oxy-dravite composition has been described from multiple occurrences (Ertl et al., Reference Ertl, Hughes, Brandstätter, Dyar and Prasad2003; Čopjaková et al., Reference Čopjaková, Škoda and Vašinová-Galiová2012; Bosi and Skogby, Reference Bosi and Skogby2013; Pieczka et al., Reference Pieczka, Ertl, Sek, Twardak, Zelek, Szeleg and Giester2018; Sȩk et al., Reference Sȩk, Włodek, Stachowitz, Woźniak and Pieczka2022). Its composition typically deviates from the ideal end-member by elevated amounts of Fe and vacancies (e.g. Novák et al., Reference Novák, Povondra and Selway2004; Čopjaková et al., Reference Čopjaková, Škoda and Vašinová-Galiová2012; Bosi et al., Reference Bosi, Reznitskii and Sklyarov2013), or Ca (e.g. Pieczka et al., Reference Pieczka, Ertl, Sek, Twardak, Zelek, Szeleg and Giester2018). The vacancy and Fe contents result mainly from foitite, oxy-schorl, or schorl components due to the substitutions (□FeOH)(NaMgO)–1, Fe1Mg–1, and (Fe3OH)(Mg2AlO)–1, respectively. The oxy-dravite from Beluga shows a slight excess (Altot = 7.06 apfu) of Al, which might indicate substitution to ‘oxy-magnesio-foitite’ via the (□Al)(NaR2+)–1 mechanism analogous to the schorl–foitite transition (e.g. Novák et al., Reference Novák, Povondra and Selway2004). The slightly elevated Ca-content is most likely to be compensated by the (CaMg)(NaAl)–1 exchange (magnesio-lucchesiite component). The compositional shift from dravite/fluor-dravite to oxy-dravite can be expressed by the substitution (MgOH/F)–1(AlO) (Bačík et al., Reference Bačík, Uher, Cempírek and Vaculovič2012; Fig. 5).

Figure 5. Composition of the Beluga oxy-dravite for X,Y,Z,W,V sites compared to compositions from other studies (Ertl et al., Reference Ertl, Hughes, Brandstätter, Dyar and Prasad2003; Čopjaková et al., Reference Čopjaková, Škoda and Vašinová-Galiová2012; Bosi and Skogby, Reference Bosi and Skogby2013; Pieczka et al., Reference Pieczka, Ertl, Sek, Twardak, Zelek, Szeleg and Giester2018; Sȩk et al., Reference Sȩk, Włodek, Stachowitz, Woźniak and Pieczka2022). Red arrows and symbols indicate position shift in Fe3+-bearing tourmalines if (Altot + Fe3+) is used instead of Altot.
Data from studies of Bosi and Skogby (Reference Bosi and Skogby2013) and Sȩk et al. (Reference Sȩk, Włodek, Stachowitz, Woźniak and Pieczka2022) plot in the oxy-dravite field (Fig. 5b); however, samples in both of those studies contain significant amounts of Fe3+ (0.610 apfu and 0.565 apfu, respectively). Adjustment of the YAl3+ values to YR3+ (which considers YFe3+) justifies their classification as oxy-dravite, at least in the case of the holotype described by Bosi and Skogby (Reference Bosi and Skogby2013). Sȩk et al. (Reference Sȩk, Włodek, Stachowitz, Woźniak and Pieczka2022) correctly considered dravitic to oxy-dravitic compositions with significant Fe3+ component.
In comparison with other data on oxy-dravite (Ertl et al., Reference Ertl, Hughes, Brandstätter, Dyar and Prasad2003; Bosi and Skogby, Reference Bosi and Skogby2013; Pieczka et al., Reference Pieczka, Ertl, Sek, Twardak, Zelek, Szeleg and Giester2018; Sȩk et al., Reference Sȩk, Włodek, Stachowitz, Woźniak and Pieczka2022), the sample studied shows similar chemical composition and structural parameters (Table 7). Oxy-dravite from the quartz vein located in a granitic gneiss of the Kowary gneiss series (Wołowa Góra Mountain; Pieczka et al., Reference Pieczka, Ertl, Sek, Twardak, Zelek, Szeleg and Giester2018), has comparable values of Si4+, Ti4+ and F–. However, the Beluga oxy-dravite has lower contents of Ca2+ (0.08 vs. 0.15 apfu) and Mg2+ (1.89 vs. 2.53 apfu) but higher contents of Fe2+ (0.15 vs. 0.04 apfu), Na+ (0.88 vs. 0.80 apfu) and Al3+tot (7.06 vs. 6.36 apfu).
Table 7. Comparison of mean bond lengths of oxy-dravite from different studies.

‘n.d.’ – not determined
Structurally, the X-site of the Beluga and holotype tourmalines is occupied predominantly by Na with minor amounts of Ca and vacancies, supported by the mean <X–O> bond length of 2.673 Å. The comprehensive review of Ertl and Tillmanns (Reference Ertl and Tillmanns2012) showed that <X–O> values range between 2.67 and 2.68 Å for tourmalines with Na = 1 apfu, whereas solely calcic tourmalines are characterised by shorter (<2.63 Å) <X–O> distances (e.g. Ertl et al., Reference Ertl, Hughes, Prowatke, Ludwig, Prasad, Brandstätter, Körner, Schuster, Pertlik and Marschall2006; Scribner et al., Reference Scribner, Cempírek, Groat, Evans, Biagioni, Bosi, Dini, Hålenius, Orlandi and Pasero2021; Bosi et al., Reference Bosi, Biagioni, Pezzotta, Skogby, Hålenius, Cempírek, Hawthorne, Lussier, Abdu, Day, Fayek, Clark, Grice and Henry2022); on the other hand, X-vacant tourmalines typically feature longer <X–O> distances (Selway et al., Reference Selway, Novák, Hawthorne, Černý, Ottolini and Kyser1998; Ertl et al., Reference Ertl, Rossman, Hughes, Prowatke and Ludwig2005; Bosi and Lucchesi, Reference Bosi and Lucchesi2007; Bačík et al., Reference Bačík, Ertl, Števko, Giester and Sečkár2015).
In comparison with solely calcic tourmalines, the mean bond length <X–O> of oxy-dravite is longer. The B-site is characterised by the mean <B–O> bond length of 1.375 Å, which is considered to be evidence of full occupancy by B (Bosi and Lucchesi, Reference Bosi and Lucchesi2007; Pieczka et al., Reference Pieczka, Ertl, Sek, Twardak, Zelek, Szeleg and Giester2018). The Y-site is occupied mainly by Mg and Al, in a lesser amount also by Fe and Ti. The mean bond length for <Y–O> is 1.999 Å, only slightly lower than in the YFe3+-rich oxy-dravites studied by Bosi and Skogby (Reference Bosi and Skogby2013) and Pieczka et al. (Reference Pieczka, Ertl, Sek, Twardak, Zelek, Szeleg and Giester2018). The ionic radius value of Fe3+ in tourmaline (0.697 Å; Bosi and Lucchesi, Reference Bosi and Lucchesi2007) is between those for Mg and Al (0.723 and 0.547 Å) and is much lower than that of Fe2+ (0.778 Å); the presence of YFe3+ therefore has a much smaller effect on <Y–O> lengths than the dominant Y,Z(Al, Mg) disorder. The Y–O1 distance is one of the shortest reported in tourmaline (e.g. Bačík, Reference Bačík2018) and indicates high WO2– content. The Z-site is occupied by Al and minor amount of Mg. The slightly extended mean <Z–O> bond length of 1.928 Å (ideally ~1.906 Å; Bosi and Andreozzi, Reference Bosi and Andreozzi2013) indicates, along with the mismatch in the size of the cations occupying the Z-site, Al–Mg disorder among the Y- and Z-sites (Grice and Ercit, Reference Grice and Ercit1993; Hawthorne, Reference Hawthorne1996 and Reference Hawthorne2002; Bosi and Luccesi, Reference Bosi and Lucchesi2007; Bosi and Skogby, Reference Bosi and Skogby2013; Pieczka et al., Reference Pieczka, Ertl, Sek, Twardak, Zelek, Szeleg and Giester2018). The arrangement of Mg and Al at the Y- and Z-sites is, in the case of oxy-dravite, closely connected with the presence of O2– at the W-site and its incorporation is controlled by the following substitution: 3YMg2+ + 2ZAl3+ + W(OH)1– ↔ 3YAl3+ + 2ZMg2+ + WO2– (Hawthorne, Reference Hawthorne1996; Henry et al., Reference Henry, Novák, Hawthorne, Ertl, Dutrow, Uher and Pezzotta2011; Bosi and Skogby, Reference Bosi and Skogby2013). The short-range bond-valence requirements at the W-site (O1) suggest two possibilities of end-members if fully occupied by WO2–: an unstable Y(Al + 2Mg)–W(O2–) configuration (or substitution) and the preferred stable configuration Y(3Al)–W(O2–) or Y(2Al + Mg)–W(O2–) (Hawthorne, Reference Hawthorne1996, Reference Hawthorne2002; Bosi and Skogby, Reference Bosi and Skogby2013). It is noteworthy that in tourmalines with significant Fe, the structure can be stabilised (and the <Y–O> distance reduced, see discussion of ionic radii above) by simple oxidation of Fe2+ to Fe3+ (exchange mechanism 3YFe2+ + 2ZFe3+ + W(OH)1– ↔ 3YFe3+ + 2ZFe2+ + WO2–) without necessity of physical octahedral cation disorder.
According to Bosi and Lucchesi (Reference Bosi and Lucchesi2007), there are structural-stability limits for tourmaline-supergroup minerals which can be expressed by the maximum difference of ca. 0.15 Å between <Y–O> and <Z–O>. In Fig. 6, we compare the <Y–O> and <Z–O> average bond lengths for natural oxy-dravites to theoretical values of some ordered and disordered ideal end-members (marked as stars in Fig. 6) calculated using the ionic radii method (Bosi and Lucchesi, Reference Bosi and Lucchesi2007). The Y-site ordered form of oxy-dravite with YAl3 Z(Al4Mg2) configuration falls into the unstable field; in contrast, both the Z-site ordered and Y,Z-site disordered forms of oxy-dravite fit the stability field (Fig. 6).

Figure 6. Mean atom distances of the Y- and Z-sites for the Beluga oxy-dravite compared to published data, theoretical values, and tourmaline structural stability limits (calculations and stability limits after Bosi and Lucchesi, Reference Bosi and Lucchesi2007). The diagram shows the general similarity of average octahedral bond lengths despite high YFe3+ in some data (Bosi and Skogby, Reference Bosi and Skogby2013; Sek et al., Reference Sȩk, Włodek, Stachowitz, Woźniak and Pieczka2022) and shift of the Beluga Fe-free composition <Y–O> towards the theoretical value for the Y(Al2Mg)(Al5Mg) configuration.
The structure of oxy-dravite is influenced significantly by the presence of WO2–, unlike dravite, so it is necessary to consider short-range bond-valence requirements around O1 (Bosi and Skogby, Reference Bosi and Skogby2013). As the configuration YMgYMgYMg–WO is unstable due to underbonding of the O1 anion, the incorporation of WO2– requires disorder of Mg and Al between the Y and Z sites (expressed by the substitution 2YMg2+ + ZAl3+ + W(OH)1– ↔ 2YAl3+ + ZMg2+ + WO2–) to keep the structure stable and electroneutral.
Petrogenesis of Beluga oxy-dravite
The Beluga occurrence of oxy-dravite in calc-silicate pods associated with marbles represents another example of its formation in Mg-rich environments related to metamorphism of a sedimentary sequence (Belley et al., Reference Belley, Dzikowski, Fagan, Cempírek, Groat, Mortensen, Fayek, Giuliani, Fallick and Gertzbein2017). Here, marine dolomitic argillaceous marl was influenced by (B,Cl)-rich fluids, probably proximally-derived from mineral breakdown reactions, in the retrograde stage of metamorphism. The localised nature of retrograde mineralisation in scapolite-bearing calc-silicate pods at Beluga and in its vicinity suggest relatively closed system processes except for the addition or loss of volatiles (Belley et al., Reference Belley, Dzikowski, Fagan, Cempírek, Groat, Mortensen, Fayek, Giuliani, Fallick and Gertzbein2017). The textural relationship between corundum and oxy-dravite indicates that oxy-dravite could have formed (1) simultaneously with corundum or (2) after corundum. Breakdown of phlogopite to muscovite and other phases (Belley, unpublished data 2023), possibly subsequent to corundum genesis, could provide Mg and other elements required to form oxy-dravite. The volume of oxy-dravite at Beluga is very low, therefore trace amounts of B in phlogopite could be sufficient if the volume of phlogopite destroyed in retrograde metamorphism is sufficient. Alternatively, B could be sourced from the breakdown of an unknown mineral phase.
The purity of Beluga oxy-dravite with regard to elevated Fe, Ti and F observed at other localities could be explained by: (1) the relatively low concentrations of Fe and Ti in Beluga calc-silicate rock relative to other oxy-dravite host rocks (i.e. Pieczka et al., Reference Pieczka, Ertl, Sek, Twardak, Zelek, Szeleg and Giester2018); (2) conservation of these elements in phlogopite, which is abundant in unaltered phlogopite–oligoclase domains contiguous to corundum- and oxy-dravite-bearing zones, reducing the availability of these elements to oxy-dravite growing in the corundum-bearing domain (see Belley et al., Reference Belley, Dzikowski, Fagan, Cempírek, Groat, Mortensen, Fayek, Giuliani, Fallick and Gertzbein2017); and/or (3) in the case of oxy-dravite formation subsequent to phlogopite breakdown in the corundum-bearing domain, the preferential incorporation of Fe and Ti in pyrrhotite and Ti-oxide, respectively. Beluga oxy-dravite's high Mg/Fe values may reflect the presence of reduced S during retrograde metamorphism (forming pyrrhotite), where Fe would strongly partition to pyrrhotite relative to silicates (see the related discussion relating to Fe partitioning in metamorphic pyrrhotite and spinel in metacarbonates in the same field area; Belley and Groat, Reference Belley and Groat2019). Ultimately, the exact factors that have resulted in the formation of near end-member oxy-dravite are difficult to establish with high accuracy due to the complex metamorphic history, large grain sizes, and extensive retrograde overprints at the Beluga sapphire occurrence.
The predominance of Na over Ca and K at the X site correlates with an end-member albite composition in plagioclase in this zone (Belley et al., Reference Belley, Dzikowski, Fagan, Cempírek, Groat, Mortensen, Fayek, Giuliani, Fallick and Gertzbein2017) which matches the experimental results of von Goerne et al. (Reference von Goerne, Franz and van Hinsberg2011). The low amounts of vacancies found in the Beluga oxy-dravite indicate high ionic strength of the fluid phase (von Goerne et al., Reference von Goerne, Franz and van Hinsberg2011) equivalent to ca. 0.33–0.40 mol/l (fluid at 300–400°C; Dutrow and Henry, Reference Dutrow and Henry2016). Berryman et al., (Reference Berryman, Wunder, Rhede, Schettler, Franz and Heinrich2016) showed that Ca-rich tourmalines are expected to form at low P and high T conditions in Ca-rich rocks; other conditions (low T, high P) show tourmaline preference for Na whereas Ca is partitioned to the coexisting fluid. These data correspond well with the Beluga tourmaline-bearing assemblage (albite + muscovite + calcite) which formed at relatively low P,T conditions; the presence of oxy-dravite therefore indicates high Na (±K) in acidic (tourmaline is not stable under alkaline conditions) fluid.
Implications for oxy-dravite occurrence
Pieczka et al. (Reference Pieczka, Ertl, Sek, Twardak, Zelek, Szeleg and Giester2018) reported that the main petrogenetic environments of oxy-dravites are aluminous metapelites and metapsammites (e.g. Bosi and Skogby, Reference Bosi and Skogby2013; Čopjaková et al., Reference Čopjaková, Škoda and Vašinová-Galiová2012; Ertl et al., Reference Ertl, Hughes, Brandstätter, Dyar and Prasad2003; Pieczka et al., Reference Pieczka, Ertl, Sek, Twardak, Zelek, Szeleg and Giester2018), low-Ca ultramafics (Sȩk et al., Reference Sȩk, Włodek, Stachowitz, Woźniak and Pieczka2022), and (Cr,V)-rich metasediments (e.g. Bosi and Lucchesi, Reference Bosi and Lucchesi2004; Cempírek et al., Reference Cempírek, Houzar, Novák, Groat, Selway and Šrein2013).
The fourth, rather rare type of oxy-dravite occurrence is in metamorphosed calcareous sediments and limestones. It was reported in metaevaporite layers in dolomite marble near Prosetín, Czech Republic (Bačík et al., Reference Bačík, Uher, Cempírek and Vaculovič2012) and at contacts of calcite–dolomite marbles with metamorphic rocks found at the Blažkov and Třebenice localities, Czech Republic (Krmíček et al., Reference Krmíček, Novák, Trumbull, Cempírek and Houzar2021). The Beluga occurrence represents a rather unique (near-end-member composition, unique association with corundum) occurrence from this type of environment. The polyphase evolution of its host rock was the key to the observed extreme depletion in typical minor elements (Fe, Cr, V, Ti, Ca and F) that are found in oxy-dravite worldwide.
Acknowledgements
The authors are grateful to Barb Dutrow and Fernando Cámara for their detailed reviews and useful suggestions that improved the quality of the manuscript. The study was supported by the Masaryk University project MUNI/IGA/1607/2020 to LS and by the Grant Agency of the Czech Republic project GAČR 19–05198S.
Supplementary material
The supplementary material for this article can be found at https://doi.org/10.1180/mgm.2023.59.
Competing interests
The authors declare none.