Obesity is a consequence of dysregulation between food intake and energy expenditure, mostly due to increased high-energetic or high-fat (HF) diet consumption and sedentarism, resulting in excess of white adipose mass( Reference Burgio, Lopomo and Migliore 1 ). Obesity or overweight is present in two-thirds of women in the reproductive age in the USA, affecting not just their own health, but also their offspring( Reference Stang and Huffman 2 ). Offspring are extremely sensitive to metabolic environmental stimuli or insults during gestation and lactation, which can result in altered physiology throughout life, a phenomenon known as ‘metabolic programming’( Reference Langley-Evans 3 ).
In adult obesity or obesity ‘programmed’ by early life insults, adipose tissue dysfunction seems to be an important contributor to the metabolic and hormonal alterations in humans and rodents. The body adipose mass is distributed in fat depots that present structural and functional differences, including white and brown adipose depots( Reference Ma, Lee and Chisholm 4 ). White adipose tissue (WAT) is organised in anatomical depots identified as visceral (VIS) and subcutaneous (SUB); VIS expansion is a greater predictor of mortality than SUB excess( Reference Ibrahim 5 ). Compared with SUB, VIS is more vascular, innervated, sensitive to lipolysis, contains a larger number of inflammatory cells, has a greater percentage of large adipocytes, a greater capacity to generate free fatty acids and is more insulin-resistant, whereas SUB is more related to uptake of circulating free fatty acids and TAG levels( Reference Ibrahim 5 ). An excess of these fat depots impairs endocrine function, as white adipocytes release several hormones and cytokines, such as leptin, adiponectin, TNFα, monocyte chemoattractant protein-1 (MCP1) and plasminogen activator inhibitor 1 (PAI-1)( Reference Burgio, Lopomo and Migliore 1 , Reference Pellegrinelli, Carobbio and Vidal-Puig 6 ). Thus, overweight and obese subjects are at increased risk for several chronic diseases, including type 2 diabetes, hypertension and liver diseases( Reference Burgio, Lopomo and Migliore 1 ). On the other side, brown adipose tissue (BAT) is related to energy expenditure because of its thermogenic capacity. This thermogenic activity is completely dependent on catecholamine signalling via β3 adrenergic receptors and is highly modulated by catabolic hormones such as thyroid hormone triiodothyronine (T3). In BAT, catecholamine or T3 signalling results in increased uncoupling protein-1 (UCP1) and heat production in brown adipocytes( Reference Labbe, Caron and Lanfray 7 – Reference Saito, Yoneshiro and Matsushita 9 ).
Recently, a third adipocyte subset has been characterised, the ‘brite’ (brown-in-white) or ‘beige’ adipocytes, which presents molecular and functional characteristics of both white and brown adipocytes. Beige adipocytes are UCP1+ cells with a multilocular morphology mainly found within WAT SUB depots but are also found in the VIS depot( Reference Hepler, Vishvanath and Gupta 10 ). The characterisation of the developmental origins of beige adipocytes is still in progress. Currently, two mechanisms have been considered for the formation of beige adipocytes: de novo beige adipogenesis and ‘transdifferentiation’ from unilocular white adipocytes( Reference Hepler, Vishvanath and Gupta 10 , Reference Wankhade, Shen and Yadav 11 ). Beige adipocyte accumulation in WAT depots is rapidly stimulated by cold exposure or ARβ3 agonism, but it can also be triggered by several nutritional components( Reference Wankhade, Shen and Yadav 11 ) and exercise-derived myokines( Reference Rocha-Rodrigues, Rodriguez and Gouveia 12 ). This phenomenon is known as ‘browning’( Reference Cohen and Spiegelman 13 ).
WAT and BAT present all the components of the endocannabinoid system (ECS)( Reference Cristino, Becker and Di Marzo 14 ), and it has been demonstrated that the ECS is usually overactive in obese subjects( Reference D’Addario, Micioni Di Bonaventura and Pucci 15 ). The ECS consists mainly of two receptors, the cannabinoid type-1 (CB1) and the cannabinoid type-2 (CB2), several endogenous ligands named endocannabinoids, anandamide (AEA) and 2-arachidonoylglycerol (2-AG) being the most active compounds, and ECS-metabolising enzymes, the fatty acid amide hydrolase (FAAH) and the monoacylglycerol lipase (MAGL). The endocannabinoids trigger several intracellular pathways following the stimulation of CB1 and CB2 receptors and their associated G proteins, mainly inhibitory G protein( Reference Matias and Di Marzo 16 , Reference Maccarrone, Bab and Biro 17 ). In WAT, the endocannabinoids stimulate lipogenesis and adipogenesis, whereas in BAT they decrease thermogenesis( Reference Maccarrone, Bab and Biro 17 ). These effects contribute to obesity development, and the ECS is currently considered as a therapeutic target for metabolic diseases( Reference Krentz, Fujioka and Hompesch 18 ).
Despite the effects of the ECS in the adipocyte physiology and ECS deregulation in adult obesity, a few studies have been conducted to investigate the role of the ECS in the developmental origins of obesity, and they are mainly focused on the central regulation of metabolism at adulthood( Reference Keimpema, Calvigioni and Harkany 19 – Reference Ramirez-Lopez, Vazquez and Bindila 23 ). However, the differential effects of maternal HF diet on the ECS’s components in VIS WAT, SUB WAT and BAT of the offspring during early life are unknown.
Using a rat model of metabolic programming, we previously demonstrated that perinatal maternal consumption of a moderate HF diet increased pre-gestational body fat in rat dams, increased milk content of total proteins, TAG and cholesterol at weaning, and induced early obesity development in male offspring( Reference Franco, Fernandes and Rocha 24 ), suggesting overnutrition of the HF pups during lactation. Endocannabinoids are arachidonic acid-derived active lipids (n-6 fatty acid family), and the ECS’s components can be modulated by dietary fat composition, with the ECS’s overactivation triggered by HF ‘western diets’ and the ECS’s attenuation observed in response to n-3 fatty acid-enriched diets( Reference Freitas, Isaac and Malcher-Lopes 25 , Reference Kim, Carlson and Kuchel 26 ). Therefore, we hypothesised that the lipid overload during lactation would alter the ECS’s profile in adipose tissue depots of the HF offspring at weaning.
We have also demonstrated that perinatal maternal HF diet induces early obesity in male offspring in parallel to hyperleptinaemia and central leptin resistance( Reference Franco, Fernandes and Rocha 24 ). A tight crosstalk has been reported between the ECS and leptin. Central leptin infusion inhibits WAT lipogenesis and local endocannabinoids production in rats( Reference Buettner, Muse and Cheng 27 ). Moreover, leptin action is inversely associated with activation of the ECS, and leptin resistance in obese models can lead to overactivation of the ECS( Reference Cristino, Busetto and Imperatore 28 , Reference Cristino, Luongo and Imperatore 29 ). Therefore, we hypothesised that early obesity and leptin resistance could also modulate the ECS’s components in adipose tissue of the HF offspring. Because the presence of sex steroid hormone response elements in the promoter of the cannabinoid receptors and the ECS-metabolising enzymes, and an in vivo regulation of the ECS by oestrogen( Reference Proto, Gazzerro and Di Croce 30 – Reference Grimaldi, Pucci and Di Siena 34 ), we further hypothesised that perinatal maternal HF diet would alter the protein abundance of the ECS’s components in adipose tissue of rat offspring in a sex-specific manner, in parallel to adipose tissue morphological and molecular signature changes and early obesity development.
Methods
Ethics statement
All procedures with animals were approved by the Animal Care and Use Committee of the Carlos Chagas Filho Biophysics Institute (process no. 123/14). The handling of the experimental animals followed the principles adopted in the UK and Brazil according to Brazilian Law no. 11.794/2008( Reference Drummond 35 , Reference Marques, Morales and Petroianu 36 ).
Animals
In all, thirty female Wistar rats (Rattus norvegicus Berkenhout, 1769), 60 d old and weighing 180–220 g, were obtained from the Center of Reproduction Biology of the Federal University of Rio de Janeiro, Rio de Janeiro, Brazil. They were kept in a controlled temperature environment (23±2°C) with a photoperiod of 12 h (07.00–19.00 hours – light and 07.00–19.00 hours – dark). Water and the experimental diets were offered on an ad libitum basis to the animals throughout the study.
Dietary treatments and experimental design
Nulliparous 60-d-old female rats were randomly assigned to two dietary treatments (n 15/group): control group (C), which received a standard diet for rodents (9 % of the energy content from fat), and a HF group, which received a HF diet (28·6 % of the energy content from fat). In the HF diet, lard was used as fat source, and we also added 1 % soya oil to provide the minimal amount of n-3 fatty acid for adequate development of the rats. Both diets were pelleted and contained approximately 16·3 kJ/g of energy and followed the AIN-93 recommendations for micronutrients( Reference Franco, Fernandes and Rocha 24 , Reference Reeves, Nielsen and Fahey 37 ). Diet composition is described in Table 1. Female rats were fed these diets during 8 weeks before mating and throughout gestation and lactation. After mating in a 3:1 ratio (female:male), pregnant rats were housed in individual standard rat cages.
Table 1 Maternal diet macronutrient composition

At birth, the litters were adjusted to three males and three females per each dam, a number that maximises lactation performance( Reference Fischbeck and Rasmussen 38 ). During lactation, offspring body weight and naso-anal length were monitored, and, at weaning (postnatal day 21), male and female pups were euthanised. All pups were killed between 09.00 and 12.00 hours in a fed state. Blood samples were taken by cardiac puncture under anaesthesia (55 mg per/kg BW ketamine and 100 mg/kg BW xylazine) followed by decapitation. Plasma samples were stored at −80°C. Retroperitoneal (VIS) and inguinal (SUB) WAT and BAT were dissected, weighted, snap frozen in liquid N2 and stored at −80°C for determination of protein content. VIS WAT, SUB WAT and BAT samples were also collected for histology analysis. For each experimental procedure, rats from at least six different litters per group were used to avoid litter effects.
Plasma metabolites
Blood samples were collected in heparinised tubes and centrifuged (1233 g for 15 min, 4°C) for plasma separation. Plasma adiponectin levels were determined using a specific rat Adiponectin ELISA Kit from Merck Millipore, with an assay sensitivity of 0·155 ng/ml. Plasma leptin, insulin, PAI-1, ILβ, TNFα and MCP1 were measured by specific rat Milliplex Adipokine Panel Metabolism Assay from Merck Millipore. Intra-assay CV was <10 % for all analytes. Plasma oestradiol levels were determined using a RIA kit (MP Diagnostics) with intra-assay variation of 15·7 %. Plasma levels of total T3 and free T4 were measured by RIA, with intra-assay CV of 4·7 and 2·8 %, respectively.
Western blotting analysis
We used the Western blotting assay to detect the protein content of CB1, CB2, FAAH, MAGL, oestrogen receptor α (ERα) and ERβ in VIS WAT, SUB WAT and BAT samples. We also detected the protein content of perilipin, PPARγ, CCAAT/enhancer binding protein α (CEBPα), acetyl-CoA carboxylase (ACC), fatty acid synthase (FAS), UCP1 and tyrosine hydroxylase (TH) in VIS WAT and SUB WAT. As regards BAT, we detected UCP1, ARβ3 and TH. The protein content of glyceraldehyde-3-phosphate dehydrogenase, β-actin or cyclophilin was used as loading control. The WAT samples were homogenised in pH 7·4 lysis buffer (20 mm TRIS-HCl, 10 mm NaF, 1 % NP40, 150 mm NaCl, 5 mM EDTA, 0,1 % SDS) containing complete protease inhibitor cocktail (Roche Diagnostics). The BAT samples were homogenised in pH 8·0 lysis buffer (50 mm TRIS-HCl, 150 mm NaCl, 0·5 % deoxy glycolic acid, 1 % NP40, 0·1 % SDS). After centrifugation, the total protein content of the supernatant was quantified using a PierceTM BCA Protein Assay Kit (Thermo Scientific). The samples were denatured in sample buffer (50 mm TRIS-HCl, pH 6·8, 1 % SDS, 5 % 2-mercaptoethanol, 10 % glycerol, 0·001 % bromophenol blue) and heated at 95°C for 5 min. Total proteins were analysed by SDS-PAGE, with a 10 % polyacrylamide gel, and transferred onto polyvinylidene difluoride membranes (Hybond-P 0·45 μm PVDF; Amersham Biosciences). The membranes were incubated with Tween TBS buffer (T-TBS) containing 5 % non-fat dry milk for 90 min to block non-specific binding sites. Thereafter, the membranes were incubated overnight at 4°C with specific primary antibodies. The membranes were washed and incubated with peroxidase labelled-specific secondary antibodies for 2 h at room temperature. All blots were washed and incubated with a luminogen detection reagent (Amersham ECL Prime Western Blotting Detection Reagent; Amersam Bioscience). Information about primary and secondary antibodies is described in Table 2. Chemiluminescent signal was detected by ImageQuant LAS 4000 equipment followed by densitometric analyses (GE Healthcare Life Sciences). For each protein analysed by Western blotting, we used six or seven samples per group, and each sample was obtained from a different rat from a different litter.
Table 2 Primary and secondary antibodies used for Western blot

CB1, cannabinoid type-1 receptor; CB2, cannabinoid type-2 receptor; FAAH, fatty acid amide hydrolase; MAGL, monoacylglycerol lipase; ERα, oestrogen receptor α, ERβ, oestrogen receptor β, CEBPα, CCAAT/enhancer binding protein α; ACC, acetyl-CoA carboxylase; FAS, fatty acid synthase; UCP1, uncoupling protein 1; ARβ3, adrenergic receptor β3; TH, tyrosine hydroxylase; GAPDH, glyceraldehyde-3-phosphate dehydrogenase.
Histology
Fragments of WAT and BAT were fixed in 4 % w/v formaldehyde phosphate buffer (0·1 m, pH 7·2) for 48 h at room temperature. The fragments were processed and embedded in Paraffin, sectioned at 5 μm and stained with haematoxylin–eosin for light microscopy observation. After processing and histological staining, the slides were observed under the optical microscope (Olympus BX51 microscope; Olympus Optical Brazil). Digital images were acquired (LC Evolution camera, TIFF format, 36-bit colour, 1280×1024 pixels) and analysed using Image Pro plus 6.0 software (Media Cybernetics). In WAT samples, the diameter of the adipocytes was analysed. For this analysis, six non-consecutive fields were used for each animal, in a total of six to eight animals from a different litter per experimental group. In BAT samples, the lipid content in the histological sections of BAT was analysed by image segmentation, and the data were expressed as percentage of the field area occupied by lipid vesicles.
Statistical analysis
The sample size was calculated by using the G*Power 3.1.9.2 program( Reference Faul, Erdfelder and Lang 39 ) and on the basis of the effect of maternal consumption of a HF diet on plasma and molecular parameters of the offspring at postnatal day 21 from previous study( Reference Franco, Fernandes and Rocha 24 ). As regards plasma parameters, a sample size of eight animals per group would provide the appropriate power (1−β=0·8) to identify significant differences (α=0·05) in the variables analysed, with an effect size d=1·33, two-tailed test, and a sample size ratio=1. For molecular parameters, a sample size of six animals per group would provide the appropriate power (1−β=0·8) to identify significant differences (α=0·05) in the variables analysed, with an effect size d=1·81, two-tailed test, and a sample size ratio=1.
The statistical comparisons were performed using the software GraphPad Prism (GraphPad Software Inc.). For all analyses, normality was assessed by the Kolmogorov–Smirnov test, and Grubb’s test was used to detect outliers.
Body weight and length during lactation were analysed using three-way ANOVA test with maternal diet, offspring sex and postnatal day (PND) as factors, and multiple comparisons were assessed by Tukey’s post hoc test, considering P<0·05 as statistically significant. Adipose tissue mass, plasma parameters, white adipocyte diameter and lipid accumulation in BAT at weaning were analysed by two-way ANOVA with maternal diet and offspring sex as factors, and multiple comparisons were assessed by Tukey’s post hoc test, considering P<0·05 as statistically significant. As regards molecular data, statistical tests were used for comparisons between control and HF offspring of the same sex. For measures that were normally distributed, a parametric test was used (Student’s unpaired t test), and, for those measures that were not normally distributed, we used non-parametric statistics (Mann–Whitney U test). Results are shown as mean values with their standard errors, considering P<0·05 as statistically significant.
Results
Body weight of the offspring during lactation was significantly affected by postnatal day (PND) of pups (P<0·0001), maternal diet (P<0·0001) and sex (P<0·0001). There was also an interaction between PND and maternal diet (P<0·0001). Multiple comparisons indicated that maternal HF diet increased body weight of male and female offspring from the 12th PND until weaning compared with sex-matched C pups (12th PND: male +16·6 %, P<0·0001; female +15·2 %, P<0·001; 15th PND: male +19·7 %, P<0·0001; female +16·9 %, P<0·0001; 18th PND: male +20·0 %, P<0·0001; female +21·2 %, P<0·0001; 21th PND: male +13·7 %, P<0·0001; female +17·5 %, P<0·0001) (Fig. 1(a)).
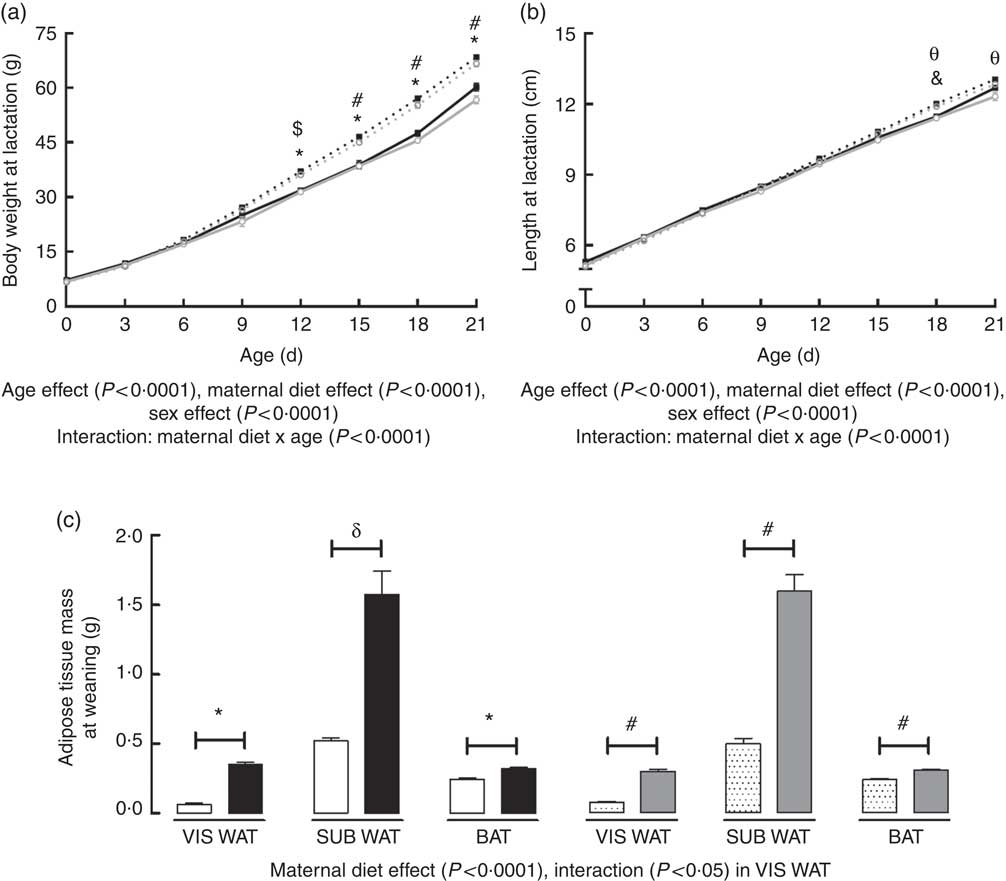
Fig. 1 Effect of maternal high-fat (HF) diet on body weight and naso-anal length during lactation and adiposity of rat offspring at weaning. (a) Body weight of control (C) and HF male and female offspring during lactation, (b) naso-anal length of C and HF male and female offspring during lactation, (c) mass of visceral white adipose tissue (VIS WAT), subcutaneous WAT (SUB WAT) and brown adipose tissue (BAT) of C and HF male and female offspring at weaning. Values are means (n 10 pups from different litters/group), with their standard errors represented by vertical bars. a and b: , C male;
, HF male;
, C female;
, HF female; c:
, C male;
, HF male;
, C female;
, HF female. Statistically significant differences were determined by three-way ANOVA (factors: postnatal day, maternal diet and sex) to analyse the body weight and length data, and by two-way ANOVA (factors: maternal diet and sex) to analyse the adipose tissue mass data. Tukey’s post hoc test: * P<0·0001, δ P<0·001, & P<0·01 for C male v. HF male offspring; #P<0·0001, $ P<0·001, θ P<0·01 C for female v. HF female offspring.
Body length of offspring was significantly affected by PND of pups (P<0·0001), maternal diet (P<0·0001) and sex (P<0·0001). There was also an interaction between PND and maternal diet (P<0·0001). Maternal HF diet increased male and female offspring length at the 18th PND compared with sex-matched C pups (male +4·7 %, P<0·01; female +4·3 %, P<0·01), but this difference remained only in female pups at weaning (+4·1 %, P<0·01) (Fig. 1(b)).
At weaning, maternal HF diet affected the retroperitoneal (VIS WAT), inguinal (SUB WAT) and BAT mass of the offspring (P<0·0001), and an interaction between maternal diet and sex was only observed in VIS WAT (P<0·05). Multiple comparisons indicated that maternal HF diet increased the mass of VIS WAT (male: +5·52-fold, P<0·0001; female +3·87-fold, P<0·0001), SUB WAT (male +3-fold, P<0·001; female +3·20-fold, P<0·0001), and BAT (male +29·4 %, P<0·0001; female +27·6 %, P<0·0001) compared with sex-matched C pups (Fig. 1(c)).
Maternal HF diet affected leptin and adiponectin plasma levels of the offspring at weaning (P<0·0001), being leptinemia was also affected by sex (P<0·05). Maternal HF diet increased leptinemia in male and female offspring (male +53·2 %, P<0·05; female +84·2 %, P<0·0001) as well as adiponectinemia (male +84·6 %, P<0·0001; female +61·1 %, P<0·0001) compared with sex-matched C pups (Table 3). Both maternal diet and sex affected plasma levels of insulin (P<0·01 and P<0·05, respectively). Multiple comparisons indicated that maternal HF diet increased insulin levels only in female offspring (+65·9 %, P<0·05) compared with sex-matched C pups (Table 3). Offspring sex also affected plasma levels of T3 (P<0·0001), PAI-1 (P<0·05) and IL-1β (P<0·05). However, multiple comparisons did not show maternal HF diet effect. We did not find maternal diet or sex effects on plasma levels of oestradiol, T4, TNF-α and MCP1 (Table 3).
Table 3 Effect of maternal high-fat (HF) diet on plasma metabolites of male and female offspring at weaning (Mean values with their standard errors)

C, control; T3, triiodothyronine; T4, thyroxine; PAI-1, plasminogen activator inhibitor 1; MCP1, monocyte chemoattractant protein-1.
* Statistical difference between C male and HF male groups (P<0·05; two-way ANOVA followed by Tukey’s post hoc test).
† Statistical difference between C female and HF female groups (P<0·05; two-way ANOVA followed by Tukey’s post hoc test).
In the present study, we characterised the effect of maternal HF diet on the protein content of the ECS’s components in different adipose tissue compartments. In VIS WAT, maternal HF diet did not change the CB1 content in male pups, whereas it increased CB1 in female pups (+52·4 %, P<0·05) (Fig. 2(a)). In contrast, maternal HF diet did not affect CB2 content in male or female pups (Fig 2(b)). Concerning the metabolising enzymes, maternal HF diet increased FAAH content in male pups (92·2 %, P<0·05), without changes in female pups (Fig. 2(c)), whereas it did not change MAGL content in male or female pups (Fig. 2(d)).
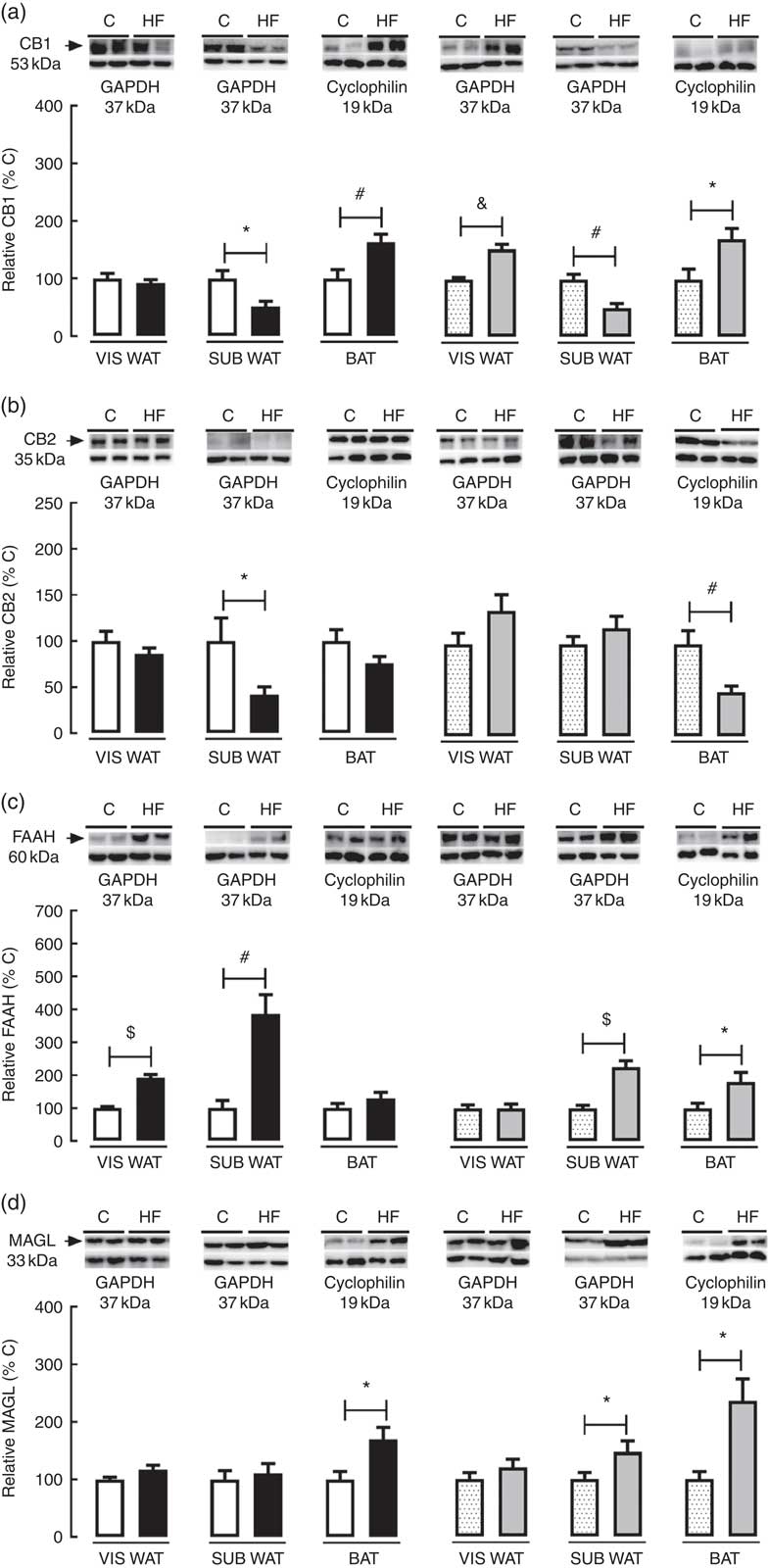
Fig. 2 Effect of maternal high-fat (HF) diet on the endocannabinoid system (ECS) components in white and brown adipose tissue of rat offspring at weaning. (a) Protein content of type-1 cannabinoid receptor (CB1) in visceral white adipose tissue (VIS WAT), subcutaneous WAT (SUB WAT) and brown adipose tissue (BAT) of control (C) and HF male and female offspring, (b) protein content of type-2 cannabinoid receptor (CB2) of C and HF male and female offspring, (c) protein content of fatty acid amide hydrolase (FAAH) of C and HF male and female offspring, and (d) protein content of monoacylglycerol lipase (MAGL) of C and HF male and female offspring. Values are means (n 6–7 pups from different litters/group), with their standard errors represented by vertical bars. , C male;
, HF male;
, C female;
, HF female; GAPDH, glyceraldehyde-3-phosphate dehydrogenase. Statistically significant differences were determined by student’s unpaired t test between C and HF offspring per each sex. * P<0·05, # P<0·01, & P<0·001, $ P<0·0001.
In SUB WAT, maternal HF diet decreased CB1 content in both male (−48·9 %, P<0·05) and female (−49·6 %, P<0·05) pups (Fig. 2(a)), whereas it decreased CB2 content only in male pups (−58·14 %, P<0·05) (Fig. 2(b)). In addition, maternal HF diet increased FAAH content in both male (+3·8-fold, P<0·05) and female (+2·2-fold, P<0·05) pups (Fig. 2(c)), whereas it increased MAGL content only in female pups (+47·6 %, P<0·05) (Fig. 2(d)).
In BAT, maternal HF diet increased CB1 content in both male (+63·8 %, P<0·05) and female (+70·5 %, P<0·05) pups (Fig. 2(a)), whereas it decreased CB2 content only in female pups (−52·0 %, P<0·05) (Fig. 2(b)). In addition, maternal HF diet increased FAAH content only in female pups (+81·4 %, P<0·05) (Fig. 2(c)), whereas it increased MAGL content in both male (+70·2 %, P<0·05) and female (+1·3-fold, P<0·05) pups (Fig. 2(d)).
As regards VIS WAT morphology, the adipocyte diameter was significantly affected by maternal diet (P<0·0001) and sex (P<0·01). Multiple comparisons indicated that maternal HF diet increased adipocyte diameter in both male (+39·2 %, P<0·05) and female (+35·3 %, P<0·05) offspring compared with sex-matched C pups (Fig. 3(a) and (b)). Maternal HF diet did not change perilipin content in VIS WAT of male or female pups (Fig. 3(c)). In contrast, maternal HF diet decreased PPARγ content in VIS WAT only in female pups (−17·7 %, P<0·05) (Fig. 3(d)), and it decreased CEBPα content only in male pups (−30·9 %, P<0·05) (Fig. 3(e)). Maternal HF diet increased ACC content in VIS WAT of male pups (+25·8 %, P<0·05) and decreased ACC content in VIS WAT of female pups (−66 %, P<0·05) (Fig. 3(f)). Maternal HF diet did not change FAS content in male or female offspring (Fig 3(g)).

Fig. 3 Effect of maternal high-fat (HF) diet on adipocyte morphology and adipogenic and lipogenic markers in white adipose tissue of rat offspring at weaning. (a) Adipocyte diameter in the visceral white adipose tissue (VIS WAT) and subcutaneous WAT (SUB WAT) of control (C) and HF male and female offspring, (b) photomicrography of C and HF male and female offspring, (c) protein content of perilipin in C and HF male and female offspring, (d) protein content of PPARγ of C and HF male and female offspring, (e) protein content of CCAAT/enhancer binding protein α (CEBPα) of C and HF male and female offspring, (f) protein content of acetyl-CoA carboxylase (ACC) of C and HF male and female offspring, and (g) protein content of fatty acid synthase (FAS) of C and HF male and female offspring. Values are means (n 6 or 8 pups from different litters/group), with their standard errors represented by vertical bars. , C male;
, HF male;
, C female;
, HF female. Statistically significant differences were determined by two-way ANOVA (factors: maternal diet and sex) to the adipocyte diameter data. Tukey’s post hoc test: $ P<0·0001. Student’s unpaired t test between C and HF offspring per each sex was used to analyse the lipogenic and adipogenic markers’ data. * P<0·05. # P<0·01.
As regards SUB WAT morphology, the adipocyte diameter was significantly affected by maternal diet (P<0·0001), and there was an interaction between maternal diet and sex (P<0·0001). Multiple comparisons indicated that maternal HF diet increased adipocyte diameter in both male (+61·7 %, P<0·05) and female (+37·8 %, P<0·05) offspring (Fig. 3(a) and (b)). Maternal HF diet did not change perilipin content in SUB WAT of male pups (Fig. 3(c)), but it increased the perilipin content in female pups (+2·8-fold, P<0·05) (Fig. 3(c)). Maternal HF diet did not change PPARγ and CEBPα content in male or female offspring (Fig. 3(d) and (e)), but it increased ACC content only in female pups (+63·5 %, P<0·05) (Fig. 3(f)). Maternal HF diet did not change FAS content in male or female offspring (Fig. 3(g)).
In BAT, maternal diet affected tissue lipid accumulation (P<0·0001). Multiple comparisons indicated that maternal HF diet increased lipid accumulation in BAT of both male (+14·1 %; P<0·05) and female (+28·8 %; P<0·05) offspring (Fig. 4(a) and (b)) compared with sex-matched C pups. Maternal HF diet did not change ARβ3 content in BAT of male or female pups (Fig. 4(c)).
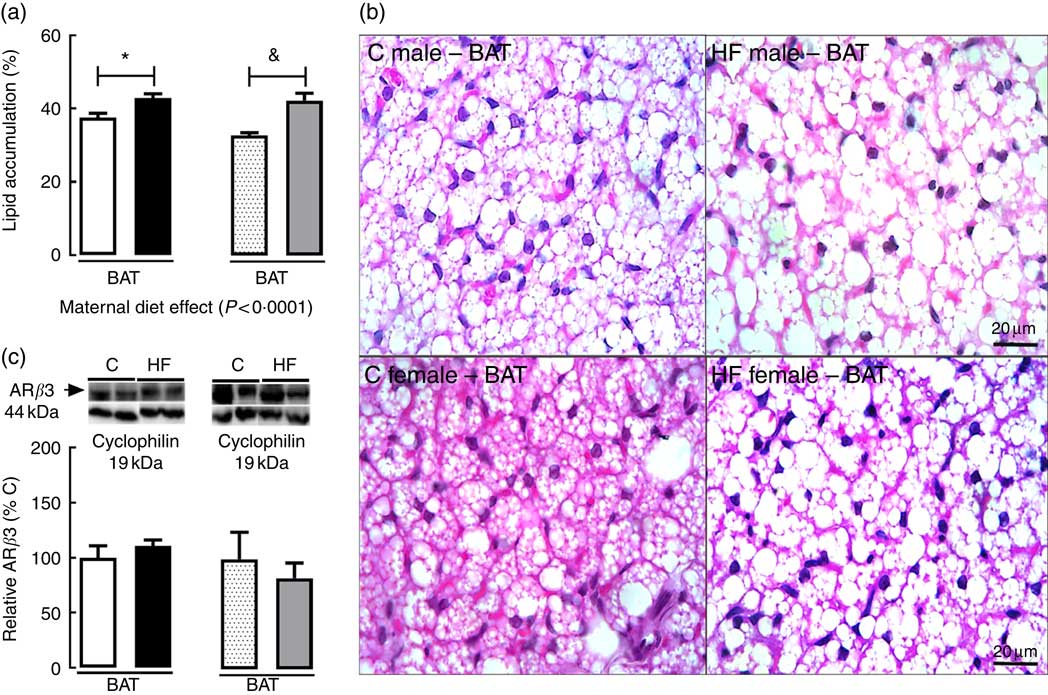
Fig. 4 Effect of maternal high-fat (HF) diet on adipocyte morphology and protein content of β3 adrenergic receptor (ARβ3) in brown adipose tissue of rat offspring at weaning. (a) Lipid accumulation in brown adipose tissue (BAT) of control (C) and HF male and female offspring, (b) photomicrography of C and HF male and female offspring, (c) protein content of ARβ3 of C and HF male and female offspring. Values are means (n 6 or 8 pups from different litters/group), with their standard errors represented by vertical bars. , C male;
, HF male;
, C female;
, HF female. Statistically significant differences were determined by two-way ANOVA (factors: maternal diet and sex) to analyse lipid accumulation data. Tukey’s post hoc test: * P<0·05, & P<0·001. Student’s unpaired t test between C and HF offspring per each sex was used to analyse ARβ3 content data.
To access the effect of maternal HF diet on markers of catecholamine tissue levels and thermogenesis in VIS WAT, SUB WAT and BAT of the offspring, we measured the tissue content of TH and UCP1 (Fig. 5). Maternal HF diet decreased TH content in VIS WAT of male pups (−32 %, P<0·05) and SUB WAT of female pups (−35 %, P<0·05), whereas it increased TH in BAT of female pups (+3-fold, P<0·05) (Fig. 5(a)). Maternal HF diet decreased UCP1 content in SUB WAT of male pups (−56 %, P<0·05), and it increased UCP1 content in BAT of female pups (+3·4-fold, P<0·05) (Fig. 5(b)).

Fig. 5 Effect of maternal high-fat (HF) diet on thermogenic markers in white and brown adipose tissue of rat offspring at weaning. (a) Protein content of tyrosine hydroxylase (TH) in visceral white adipose tissue (VIS WAT), subcutaneous WAT (SUB WAT) and brown adipose tissue (BAT), (b) protein content of uncoupling protein-1 (UCP1) of control (C) and HF male and female offspring. Values are means (n 6 or 8 pups from different litters/group), with their standard errors represented by vertical bars. , C male;
, HF male;
, C female;
, HF female; GAPDH, glyceraldehyde-3-phosphate dehydrogenase. Statistically significant differences were determined by Student’s unpaired t test between C and HF offspring per each sex. * P<0·05, # P<0·01.
As regards ERα and ERβ content in WAT and BAT, maternal HF diet decreased ERα (−47·8 %, P<0·05) and ERβ (−46·8 %, P<0·05) in VIS WAT of female pups (Fig. 6(a) and (b)). In SUB WAT, maternal HF diet increased ERα in male pups (+77·4 %, P<0·05) and decreased ERα (−39·1 %, P<0·05) in female pups, whereas it did not change ERβ in both sexes (Fig. 6(a) and (b)). In BAT, maternal HF diet increased ERα only in male offspring (+77·0 %, P<0·05), whereas it increased ERβ only in female pups (+89·4 %, P<0·05) (Fig. 6(a) and (b)).

Fig. 6 Effect of maternal high-fat (HF) diet on oestrogen receptor (ER) content in white and brown adipose tissue of rat offspring at weaning. (a) Protein content of ERα in visceral white adipose tissue (VIS WAT), subcutaneous WAT (SUB WAT) and brown adipose tissue (BAT) of control (C) and HF male and female offspring, and (b) protein content of ERβ of C and HF male and female offspring. Values are means (n 6 or 7 pups from different litters/group), with their standard errors represented by vertical bars. , C male;
, HF male;
, C female;
, HF female; GAPDH, glyceraldehyde-3-phosphate dehydrogenase. Statistically significant differences were determined by Student’s unpaired t test between control and HF offspring per each gender. * P<0·05, # P<0·01, $ P<0·0001.
The effect of maternal HF diet on the molecular profile in VIS WAT, SUB WAT and BAT of male and female offspring is presented in an integrated view in Table 4.
Table 4 General effect of maternal high-fat (HF) diet on the protein content of the endocannabinoid system components, adipocyte function markers and thermogenic markers in visceral white adipose tissue (WAT), subcutaneous WAT and brown adipose tissue (BAT) of the offspring

CB1, cannabinoid type-1 receptor; =, unchanged; ↑, increased; ↓, decreased; CB2, cannabinoid type-2 receptor; FAAH, fatty acid amide hydrolase; MAGL, monoacylglycerol lipase; un, unmeasured; CEBPα, CCAAT/enhancer binding protein α; ACC, acetyl-CoA carboxylase; FAS, fatty acid synthase; UCP1, uncoupling protein 1; ARβ3, adrenergic receptor β3; TH, tyrosine hydroxylase; ERα, oestrogen receptor α, ERβ, oestrogen receptor β.
Discussion
In the present study, we used an experimental model of obesity induced by maternal HF diet consumption during pre-gestational period, gestation and lactation. First, we confirmed and expanded the characterisation of the obese phenotype already observed in male offspring at weaning( Reference Franco, Fernandes and Rocha 24 , Reference Oliveira, Souza and Souza 40 ). In addition, we also characterised the phenotype of female offspring, as we hypothesised that there would be a differential molecular ECS profile in adipose tissue associated with early obesity in male and female HF offspring.
Maternal HF diet increased body weight, body length and adiposity in both male and female offspring at weaning. According to our previous study, early obesity in HF offspring is likely a consequence of overnutrition during lactation, as breast milk of the HF progenitors presents a higher concentration of lipids, protein and carbohydrates( Reference Franco, Fernandes and Rocha 24 ). Increased adiposity is commonly associated with reduced plasma levels of adiponectin and increased levels of leptin( Reference Lopez-Jaramillo, Gomez-Arbelaez and Lopez-Lopez 41 , Reference Ahima 42 ). However, in neonates, the association between body mass and adiponectinemia seems to be a direct relationship, whereas children and adults exhibit an inverse association( Reference Nakano, Itabashi and Sakurai 43 ). Here, we showed that maternal HF diet increased plasma levels of adiponectin in male and female offspring at weaning. In agreement with our results, the adiponectinemia of human neonates is positively related to body weight and length during the three weeks after birth( Reference Hansen-Pupp, Hellgren and Hard 44 ), and it presents a positive association with increased SUB adiposity( Reference Nakano, Itabashi and Sakurai 43 ). In contrast, the hyperleptinaemia and increased adiposity observed in male pups confirmed our previous results( Reference Franco, Fernandes and Rocha 24 ) and corroborates results from other studies( Reference Friedman and Halaas 45 – Reference Desai, Jellyman and Han 47 ). We have previously shown that this phenotype of HF male offspring persists during adolescence and adulthood( Reference Franco, Fernandes and Rocha 24 , Reference Oliveira, Souza and Souza 40 , Reference Franco, Dias-Rocha and Fernandes 48 ). At weaning, hyperleptinaemia was associated with decreased hypothalamic leptin-signalling markers, suggesting central leptin resistance( Reference Franco, Fernandes and Rocha 24 ). This is important because leptin resistance is associated with positive energy balance and contributes to obesity development by increasing food intake and decreasing energy expenditure( Reference Bjorbaek 49 ).
Obesity and leptin resistance have been associated with tissue-specific alterations of the ECS’s signalling in the central nervous system and peripheral tissues of adult rodents and humans( Reference Iannotti, Piscitelli and Martella 50 – Reference Jelsing, Larsen and Vrang 55 ). In the present study, we demonstrated that maternal HF diet resulted in differential regulation of the ECS’s components across WAT and BAT depots in male and female offspring at weaning, a period of life considered as a critical window for developmental origins of health and disease (DOHaD)( Reference Armitage, Poston and Taylor 56 ).
Adequate levels of endocannabinoids in early life likely have a key role in feeding and body weight regulation, as it was demonstrated that AEA and 2-AG are present in placenta and breast milk of healthy subjects( Reference Fonseca, Correia-da-Silva and Taylor 57 – Reference Vaswani, Chan and Peiris 59 ). In addition, AEA treatment during lactation programmes energy metabolism in a short-term and long-term manner( Reference Aguirre, Castillo and Llanos 60 , Reference Aguirre, Castillo and Llanos 61 ). The local levels of endocannabinoids are regulated by the action of important metabolising enzymes, such as FAAH and MAGL. Maternal HF diet increased FAAH content in VIS WAT of male offspring at weaning, suggesting decreased local content of AEA( Reference Henry, Kerr and Flannery 62 , Reference Engeli, Bohnke and Feldpausch 63 ), which is preferentially metabolised by this enzyme( Reference Henry, Kerr and Flannery 62 , Reference Engeli, Bohnke and Feldpausch 63 ). Increased FAAH content in male VIS WAT may be related to the obesity phenotype, as an increase of FAAH content in the adipose tissue of obese men( Reference Murdolo, Kempf and Hammarstedt 64 ) and a positive correlation between FAAH activity in adipocytes and BMI( Reference Cable, Tan and Alexander 65 ) has been demonstrated.
In white adipocytes, endocannabinoids activate de novo lipogenesis( Reference Silvestri and Di Marzo 66 ). Maternal HF diet increased the content of the lipogenic enzyme ACC in VIS WAT of male offspring and decreased the adipogenic factor CEBPα ( Reference Tang, Gronborg and Huang 67 ). This profile may contribute to hypertrophy of the preexisting adipocytes, as the differentiation of new pre-adipocytes to store extra lipids generated by increased lipogenesis might be impaired, based on the reduced content of CEBPα. In accordance, we observed increased adipocyte diameter in VIS WAT of HF male offspring. Hypertrophic adipocytes present a high metabolic turnover, with increased lipogenesis, reduced capacity of free fatty acids uptake and increased fatty acid release, resulting in increased lipid flux into non-adipose organs, raising the risk to develop insulin resistance and cardiovascular diseases throughout life( Reference Gaggini, Saponaro and Gastaldelli 68 ).
As regards, CB1 regulation in VIS WAT of female offspring, maternal HF diet increased CB1 content, which can also contribute to increased lipogenesis, adipocyte hypertrophy and higher adipose tissue mass( Reference Silvestri and Di Marzo 66 ), as we observed in this group. Previous study showed that maternal exposure to a highly energetic and palatable diet increases CB1 mRNA levels in visceral adipose tissue of female adult rat offspring( Reference Ramirez-Lopez, Arco and Decara 21 ). Here, we demonstrated that maternal HF diet also increased the protein levels of CB1, and this alteration occurs already in early life. Increased expression of CB1 in WAT is associated with hyperinsulinaemia, which is restored by CB1 antagonist administration( Reference Jourdan, Djaouti and Demizieux 69 ). In the present study, we observed increased CB1 and hyperinsulinaemia only in female HF offspring, which may contribute to insulin resistance( Reference Shanik, Xu and Skrha 70 , Reference Juan, Chen and Wang 71 ).
CB1 expression in adipocytes is down-regulated by PPARγ ( Reference Pagano, Pilon and Calcagno 72 ), and maternal HF diet decreased PPARγ content in the VIS WAT of female offspring. Therefore, the decreased PPARγ content may contribute to increased CB1 content observed in this depot. PPARγ is an important factor for both lipogenesis and adipogenesis in WAT( Reference Tang, Gronborg and Huang 67 ), and it increases the number of small adipocytes and decreases large adipocytes in WAT of obese rats( Reference Okuno, Tamemoto and Tobe 73 ). PPARγ knockout mice fed with a HF diet accumulate a similar amount of excess fat compared with wild-type mice, but this extra lipid storage is associated with more hypertrophied adipocytes( Reference Zhang, Fu and Cui 74 , Reference Medina-Gomez, Virtue and Lelliott 75 ). In contrast, a contrary profile is observed with PPARγ agonist treatment, such as thiazolidinedione (TZD), which increases the adipocyte number and decreases the adipocyte area in WAT depots under control or HF diet in rats( Reference Johnson, Trasino and Ferrante 76 ). In addition, WAT PPARγ down-regulation was observed in adult rats programmed by maternal obesity( Reference Lecoutre, Deracinois and Laborie 46 ). We speculate that decreased PPARγ in VIS WAT of HF female offspring is associated with decreased adipogenesis, and therefore this could lead to hypertrophy of preexisting adipocytes in consequence of fat overload imposed by maternal HF diet. Surprisingly, we observed decreased ACC content in VIS WAT of female HF offspring. As ACC is a lipogenic enzyme and HF females presented increased VIS WAT adipocyte diameter, we speculated that this profile might be related to decreased PPARγ in this group, as the simultaneous down-regulation of PPARγ and ACC( Reference Li, Liu and Shiu 77 – Reference Sharma, Oh and Kim 79 ) has been shown in adipocytes.
To better understand the sex-specific effect of maternal HF diet on the ECS in VIS WAT, we evaluated the content of ERα and ERβ in adipose tissue of male and female offspring. Maternal HF diet reduced ERα and ERβ content only in VIS WAT of female pups in parallel to increased CB1. In a very recent paper, it was demonstrated that oestradiol increases cannabinoid receptor expression in the uterus of ovariectomised rats( Reference Maia, Almada and Silva 33 ), but the direct role of oestrogen on adipose tissue CB1 content is unknown, and, possibly, oestrogen regulates CB1 in a tissue-specific manner.
It has been proposed that ERα mediates beneficial metabolic effects of oestrogens, such as anti-lipogenesis, improvement of insulin sensitivity, reduction of body weight, decrease of adipose tissue mass( Reference Bluher 80 ) and prevention of white adipocyte hypertrophy( Reference Heine, Taylor and Iwamoto 81 ). Therefore, the reduced VIS WAT ERα content corroborates the increased VIS adiposity, VIS adipocyte hypertrophy and hyperinsulinaemia presented in HF female offspring. ERα is the predominant ER in WAT, whereas the role of ERβ is poorly known in this tissue( Reference Barros, Gabbi and Morani 82 ). It was previously demonstrated that ERβ knockout mice presented increased body weight gain and fat mass when fed a HF diet( Reference Foryst-Ludwig, Clemenz and Hohmann 83 ), and ERβ agonist reduced VIS fat mass and adipocyte size in ovariectomised rats( Reference Weigt, Hertrampf and Zoth 84 ). Therefore, the decreased ERβ content in VIS WAT of HF female offspring also corroborates the increased body weight, VIS adiposity and VIS adipocyte hypertrophy in female HF offspring.
In SUB WAT, in contrast to VIS WAT, maternal HF diet decreased CB1 content and increased FAAH content in both male and female offspring. This profile indicates reduced CB1 signalling and decreased AEA levels( Reference Henry, Kerr and Flannery 62 , Reference Engeli, Bohnke and Feldpausch 63 ). Furthermore, in female pups, maternal HF diet also increased MAGL content, which indicates reduced 2-AG levels( Reference Pasquarelli, Porazik and Hanselmann 85 , Reference Vander Tuig, Kerner and Romsos 86 ). Here, we demonstrated that the reduced CB1 content in SUB WAT was sex independent and may occur because of metabolic features of this depot. CB1 knockout SUB adipocytes showed increased lipid accumulation during adipogenesis and decreased apoptosis compared with wild-type SUB adipocyte( Reference Wagner, Perwitz and Drenckhan 87 ).
In SUB WAT of male offspring, maternal HF diet also decreased CB2 content. The role of CB2 is still unclear in energy metabolism regulation( Reference Soethoudt, Grether and Fingerle 88 ). Generally, CB2 expression has been associated with inflammatory processes, but in vivo and in vitro studies show controversial results with regard to proinflammatory v. anti-inflammatory profile( Reference Verty, Stefanidis and McAinch 89 – Reference Deveaux, Cadoudal and Ichigotani 92 ). It was already shown that the CB2 agonist treatment reduced white adipose mass and white adipocyte cell size in mice( Reference Verty, Stefanidis and McAinch 89 ). Thus, the decreased CB2 content might be associated with increased SUB adiposity and adipocyte hypertrophy in SUB WAT of HF male offspring. In addition, maternal HF diet increased ERα in SUB WAT of HF male offspring. However, these molecular changes in SUB WAT of male offspring were not associated with changes in lipogenic or adipogenic markers. It is possible that the ECS and oestrogen signalling changes in this fat depot may be associated with lipolysis markers not evaluated in the present study. In contrast, in SUB WAT of female offspring, maternal HF diet decreased ERα content, which may be associated with lipogenic stimulus and corroborates the higher content of perilipin and ACC with adipocyte hypertrophy observed in this group.
The present findings with regard to the effect of maternal HF diet on the ECS and ER in different white adipose compartments of the offspring clearly show a dimorphic effect of this maternal nutritional insult. However, it is still unclear why a similar phenotype in VIS WAT of female HF offspring and SUB WAT of HF male offspring was observed, with quite different molecular signatures. Although VIS WAT of HF female offspring presented a ‘deleterious’ molecular profile, SUB WAT of male HF offspring presented a ‘beneficial profile’ characterised by decreased CB1 and CB2 and increased FAAH and ERα. This discordant association between the ECS, ER and lipogenesis/hypertrophy in HF offspring may arise from several reasons. First, CB1 differentially regulates VIS and SUB WAT. In mice, decreased CB1 in VIS depot is associated with decreased differentiation, whereas it is associated with increased differentiation in SUB depot( Reference Wagner, Perwitz and Drenckhan 87 ). In this distinct regulation, CB1 may favour fat redistribution from SUB to VIS compartments, as previously suggested by Di Marzo( Reference Di Marzo 93 ). Moreover, VIS WAT and SUB WAT present distinct gene expression modulation by Rimonabant (CB1 antagonist) in obese mice( Reference Jourdan, Djaouti and Demizieux 69 ), suggesting the differential role of CB1 in each adipose depot. Here, we observed decreased CB1 in SUB WAT of male and female HF offspring, but only HF females presented higher CB1 VIS WAT content, showing that the dimorphism of CB1 is more related to VAT. A similar profile was observed in overweight humans, with decreased content of CB1 in SUB WAT and increased CB1 content in VIS WAT( Reference Sarzani, Bordicchia and Marcucci 94 ). Second, the absolute content of CB1 is likely different between VIS and SUB, which can differently impact the energy metabolism( Reference Bennetzen, Nielsen and Paulsen 51 , Reference Bluher, Engeli and Kloting 52 , Reference Sarzani, Bordicchia and Marcucci 94 ). However, there is still no consensus with regard to the relative CB1 expression between these two WAT depots. Third, the association between obesity and CB1 content in WAT is also contradictory. It was demonstrated that CB1 expression is decreased in SUB WAT of obese patients,( Reference Bluher, Engeli and Kloting 52 ) and its expression in rescued after weight lost( Reference Bennetzen, Wellner and Ahmed 95 ). Contrarily, Engeli et al.( Reference Engeli, Lehmann and Kaminski 96 ) did not find any difference in CB1 content between lean and obese patients.
To further investigate the mechanisms that could be involved in the differential regulation of the ECS in WAT of HF offspring, we assessed the levels of ‘browning’ in VIS WAT and SUB WAT by measuring UCP1 and TH tissue content. It has been proposed that increased adrenergic signalling in SUB WAT stimulates UCP1 expression, a marker of ‘browning’, in parallel to increased CB1 and decreased CB2 expression( Reference Krott, Piscitelli and Heine 97 ). In agreement, here we observed decreased content of UCP1 in SUB WAT of male HF offspring in parallel to decreased CB1 in these depots, but also increased levels of CB2. Increased UCP1 in SUB WAT of male offspring corroborates a recent study by Zou et al.( Reference Zou, Chen and Yang 98 ). In addition, it was demonstrated that decreased levels of endocannabinoids in SUB WAT decreases local thermogenic markers( Reference Geurts, Everard and Van Hul 99 ). In contrast, in SUB WAT of female HF offspring, we observed decreased levels of TH, suggesting decreased adrenergic signalling, which was also associated with decreased CB1 content without changes in CB2. Interestingly, an important distinction between male and female SUB WAT is the content of ERα, which was increased in male and decreased in female HF offspring. These data suggest that maternal HF diet modulates cannabinoid receptors in SUB WAT of the offspring at least in part by integrative changes in adrenergic signalling, ‘browning’ levels and oestrogen signalling.
The thermogenic function of BAT is completely dependent on SNS and can be inhibited by the ECS( Reference Quarta, Bellocchio and Mancini 100 ). Maternal HF diet increased BAT weight and lipid content in male and female offspring, corroborating data from maternal HF diet and litter reduction experimental models( Reference de Almeida, Fabricio and Trombini 101 , Reference Liang, Yang and Zhang 102 ). In BAT, maternal HF diet increased CB1 and MAGL content in the offspring, independently of sex, whereas it increased FAAH content only in female offspring. The increased content of degrading enzymes suggests reduced local levels of AEA and 2-AG, as previously shown in genetic and diet obese models( Reference Iannotti, Piscitelli and Martella 50 ), and might be an adaptive consequence of up-regulation of CB1 in BAT. To investigate whether the ECS changes induced by maternal HF diet in BAT occurred in parallel with changes in thermogenic markers, we evaluated BAT content of UCP1, and the catecholamine signalling markers TH and ARβ3. Maternal HF diet did not alter thermogenic markers in BAT of male pups but increased TH and UCP1 content in female pups. The relationship between the ECS and thermogenesis has been characterised using in vivo and in vitro experimental models. In vivo, CB1 antagonism results in increased BAT UCP1 content, increased BAT temperature and energy expenditure, with reduced weight gain in intact and BAT-denervated rats( Reference Verty, Allen and Oldfield 103 , Reference Hsiao, Shia and Wang 104 ). Similar results were observed in vitro using differentiated brown adipocytes( Reference Boon, Kooijman and van Dam 105 ). In contrast, pharmacological or cold activation of the sympathetic signalling in BAT of mice results in increased CB1 mRNA levels and decreased CB2 mRNA levels in parallel to increased UCP1( Reference Krott, Piscitelli and Heine 97 ). Therefore, using in vivo models, it is difficult to establish causality between the ECS changes and BAT markers. Moreover, in the present study, we used weanling rats, which are still under the effect of maternal HF diet through breast milk, and hyperenergetic food intake can activate diet-dependent thermogenesis( Reference Lettieri Barbato, Tatulli and Vegliante 106 ). We speculate that maternal HF-activated diet induced thermogenesis stimulating sympathetic activation of BAT by increasing TH content, which increased UCP1 and differentially regulated CB1 and CB2 in female pups, similar to cold-induced effects on the ECS( Reference Krott, Piscitelli and Heine 97 ).
Besides catecholamine signalling, the UCP1 expression and thermogenesis are stimulated by oestrogen and T3( Reference Townsend and Tseng 107 , Reference Lopez and Tena-Sempere 108 ). Female HF offspring presented increased plasma levels of T3, suggesting higher T3 signalling in BAT, without changes in oestrogen serum levels. However, maternal HF diet increased ERβ content in female offspring, whereas it increased ERα content in male offspring, suggesting higher sensitivity to oestrogen in BAT.
There are several mechanisms known to be associated with DOHaD that could contribute to the sex-dependent and depot-dependent programming of adipose tissue in the present study. In a general view, programming is associated with epigenetic regulation of transcription by changing the levels of DNA methylation, histone acetylation/methylation and ncRNA. Offspring epigenome can be influenced by age, sex, and nutritional factors among other modulators( Reference Lillycrop and Burdge 109 ). For example, obese rat offspring from obese dams present altered fatty acid composition within WAT, with decreased content of PUFA( Reference Benkalfat, Merzouk and Bouanane 110 ), key regulators of the ECS’s components and adipogenic markers such as PPARγ. More specifically, some of the ECS’s components are regulated by epigenetic mechanisms( Reference D’Addario, Di Francesco and Pucci 111 ), which deserves deeper investigation in future experiments of programming by maternal nutritional insults.
In addition, each adipose tissue depot presents its unique signature of developmental gene expression showing differential profile even within the same general type, that is, VIS or SUB depots. This gene expression signature includes different levels of PPAR and CEBP, which are highly influenced by the hormonal milieu. Leptin differentially regulates PPARγ2 in WAT, as leptin-deficient obese mice present higher PPARγ2 content in the SUB WAT and decreased PPARγ2 in VIS WAT, compared with control mice( Reference Yamamoto, Gesta and Lee 112 ). Altered leptin level in early life is likely a key imprinting factor of energy homoeostasis and obesity in several programming models, including maternal HF diet( Reference Breton 113 ). One of the marked features of the present model is that HF offspring present hyperleptinaemia across life, which could differentially modulate the ECS’s components in VIS WAT and SUB WAT.
As regards the sex-specific programming of the ECS, we speculated that it may be related to oestrogen regulation, as the presence of response elements to oestrogen in Cnr1 and Faah promoter region( Reference Proto, Gazzerro and Di Croce 30 , Reference Waleh, Cravatt and Apte-Deshpande 32 ) has been reported. Androgen and progesterone may also be involved in the ECS’s programming. Androgen response element was also described in the Cnr1 promoter region( Reference Grimaldi, Pucci and Di Siena 34 ). However, very little is known as regards the direct ECS regulation by sex steroids, even in control rats/mice/humans. Another important fact that requires attention is the age of the offspring in the present study. We used weanling rats, that is, before puberty and the rise of circulating levels of sex hormones, as we observed unchanged oestradiol serum levels. We speculate that the ECS’s modulation is taking place, at least in part, through the regulation of oestrogen sensitivity and ER differential content among the several adipose compartments analysed in our experiment. Finally, the modulation of ECS linked to chromosomal sex (XX v. XY) should be considered as a sexual dimorphism mechanism( Reference Valencak, Osterrieder and Schulz 114 ).
The main limitations of the present study include the lack of measurement of tissue content of the endocannabinoids AEA and 2-AG to provide a clearer interpretation of the results concerning the content of the metabolising enzymes FAAH and MAGL. Moreover, although we showed the sex difference in the expression pattern of ER in the different adipose depots, the biological mechanisms mediating sex bias have not been clearly defined. Further investigation into the direct effect of oestrogens and androgens on the ECS’s components is necessary in future studies.
In conclusion, maternal HF diet during the perinatal period induced early obesity in the offspring, independently of the sex, but induced differential regulation of the adipose tissue’s ECS’s components between male and female offspring at weaning. Sex-specific ECS changes seem to be more evident in white adipose depots compared with brown adipose depots. The ECS changes occurred in parallel to alteration of molecular markers of adipogenesis, lipogenesis and thermogenesis as well as differential ER content across the adipose tissue depots. Collectively, our results suggest that the ECS is highly regulated in early life in response to nutritional milieu and may be involved in the early origins of metabolic disorders.
Acknowledgements
The authors are grateful for the technical support provided by Luela Dias and Juliana Penna.
This study was supported by the Carlos Chagas Filho Research Foundation of the State of Rio de Janeiro (FAPERJ) (grant no. E-26/202.816/2015) and National Council for Scientific and Technological Development (CNPq) (grant no. 473807/2013-0).
M. M. A., C. P. D.-R., A. S. S. and M. F. M. have participated in animal experiments, collection and interpretation of data. L. S. M. contributed with the morphological analysis. I. H. T. and C. C. P.-M delineated experimental design and supervised the study. M. M. A., C. P. D.-R., C. C. P.-M. and I. H. T. have written the manuscript.
None of the authors has any conflicts of interest to declare.