Coffee is one of the most widely consumed beverages throughout the world, and its origin dates back to the fifteenth century or earlier( Reference D’Amicis and Viani 1 ). Epidemiological studies have identified coffee consumption as a factor associated with reduced risks of type 2 diabetes( Reference Greenberg, Boozer and Geliebter 2 , Reference Van Dam and Feskens 3 ), liver cirrhosis( Reference Tverdal and Skurtveit 4 ), Alzheimer’s disease( Reference Eskelinen, Ngandu and Tuomilehto 5 ) and Parkinson’s disease( Reference Hernán, Takkouche and Caamaño-Isorna 6 ). The coffee bean, and especially the green coffee bean, is known to be a rich source of biologically active compounds such as polyphenolic antioxidants including 5-caffeoylquinic acid (5-CQA) (formerly called 3-CQA or chlorogenic acid (CGA))( Reference Stalmach, Steiling and Williamson 7 ), 3-CQA, 4-CQA, 3,4-dicaffeoylquinic acid (3,4-diCQA), 3,5-diCQA, 4,5-diCQA, 3-feruloylquinic acid (3-FQA), 4-FQA, and 5-FQA, which consist of CGA( Reference Clifford and Wight 8 , 9 ).
Recent human studies have shown that daily consumption of 340–600 mg/d of CGA (equivalent to one–two cups of coffee( Reference Fukushima, Ohie and Yonekawa 10 )) induced favourable effects on fat oxidation( Reference Soga, Ota and Shimotoyodome 11 ) and lowered postprandial glucose( Reference Jokura, Watanabe and Umeda 12 ) and insulin resistance( Reference Lecoultre, Carrel and Egli 13 ) as well as had favourable effects on endothelial functions( Reference Ochiai, Sugiura and Otsuka 14 , Reference Ochiai, Sugiura and Shioya 15 ) and mood enhancement( Reference Camfield, Silber and Scholey 16 ). Accumulating evidence has suggested that CGA exhibit many biological properties including antioxidant( Reference Kono, Kobayashi and Tagawa 17 ), anti-obesity( Reference Cho, Jeon and Kim 18 ), hypoglycaemic and hypolipidaemic effects( Reference Meng, Cao and Feng 19 ). Moreover, a recent study demonstrated that CGA exerts anti-amnesic activity via inhibition of acetylcholinesterase and malondialdehyde in the hippocampus and frontal cortex( Reference Kwon, Lee and Kim 20 ). Foods and associated bioactive substances often affect multiple physiological functions, as exemplified by caffeine acting on the autonomic nervous system( Reference Porciúncula, Sallaberry and Mioranzza 21 ), energy metabolism( Reference Arciero, Gardner and Calles-Escandon 22 , Reference Bracco, Ferrarra and Arnaud 23 ) and sleep( Reference Júdice, Magalhães and Santos 24 ). Cross-talk between physiological functions exists within the body, and several lines of evidence suggest a link between sleep, the autonomic nervous system and energy metabolism( Reference Hirotsu, Tufik and Andersen 25 ). Neurosubstances such as orexin, leptin and melatonin act on sleep and wakefulness and on energy balance( Reference Nicolaidis 26 – Reference Sakurai 28 ). The effects of CGA ingestion on carbohydrate and fat metabolism warrant additional studies evaluating the effects of CGA ingestion on sleep, the lack of which has been identified as a risk factor for, amongst other conditions, obesity, hypertension and type 2 diabetes( Reference Patel and Hu 29 – Reference Nielsen, Danielsen and Sørensen 31 ). Metabolites of CGA potentiated pentobarbital-induced sleep by prolonging sleeping time and shortening sleep latency( Reference Tu, Cheng and Sun 32 ), but the effects of consuming CGA on human sleep have not been studied.
The present study evaluated the effects of ingesting 600 mg of CGA on sleep architecture, energy metabolism and autonomic nervous function in healthy male and female subjects. Simultaneous measurements of sleep architecture and respiratory analysis could provide new insights regarding which CGA exert possible sleep and energy metabolism improvements. To evaluate energy metabolism during sleep, whole-room indirect calorimetry was adopted, which provides a controlled environment in which energy metabolism can be continuously measured for a long period, including during sleep.
Methods
Subjects
In all, four healthy male subjects and five healthy female subjects with a mean age of 25·7 years and BMI of 21·8 kg/m2 participated in this study. Subjects had normal sleeping profiles based on the Pittsburgh sleep quality index (≤5). No subject had engaged in regular exercise more than twice a week or used any regular or temporary medication. Exclusion criteria for the subjects were a clinical diagnosis of sleep apnoea hypopnea syndrome, a medical or psychiatric condition, smokers and shift workers. Subjects who drank more than a bottle of alcohol (350 ml) per day were also excluded from the study. For female subjects, those with a severe menstrual cycle problem and those who were pregnant were excluded. One female subject was excluded from the statistical analysis of energy metabolism because of mechanical trouble (unstable flow control of the metabolic chamber) during the trial with CGA. This study was conducted according to the guidelines laid down in the Declaration of Helsinki and all procedures involving human subjects were approved by the Ethics Committee of the University of Tsukuba. All subjects provided written informed consent before study commencement; the protocol was registered with Clinical Trials UMIN, ID no.: UMIN000022889.
Protocol
The present study was a placebo-controlled, double-blinded, cross-over intervention study. During each 5-d session, a test beverage containing 0 (control (CON)) or 600 mg of CGA was consumed 10 min before bedtime and subjects were asked to refrain from ingesting beverages containing caffeine and alcohol. During the study, the subjects maintained their usual activities and dietary habits, which were confirmed by actigraphy recording and a daily diary. On days 3–5, the subjects ingested specified meals for breakfast, lunch and dinner, which were provided by the study coordinators.
On the 5th day, subjects consumed lunch in the laboratory and stayed indoors. After consuming dinner, subjects entered the whole-room metabolic chamber and remained sedentary. Subjects went to bed at their usual bedtime and slept for 8 h. Energy metabolism was measured for 16 h (from 3 h before bedtime on the 5th day to 5 h after waking the next morning).
The meals provided in the laboratory were based on energy requirements estimated from the BMR equation( 33 ) with a physical activity level of 1·3. The meal composition (energy percentage (E%)) was 15 E% protein, 25 E% fat and 60 E% carbohydrates, and both groups received meals identical in quantity and composition. The energetic distribution was 30 E% for breakfast, 30 E% for lunch and 40 E% for dinner.
The experiment was preceded by an adaptation night in the metabolic chamber, during which the sensors and electrodes of the polysomnographic recording system were attached to the subjects. The two trials were separated by a washout period of 2–4 weeks, and studies with female subjects were performed during the same phase of the menstrual cycle; three female subjects were evaluated during the follicular phase and the other two female subjects were evaluated during the luteal phase.
Beverages
The CGA and placebo test beverages were prepared to be indistinguishable on the basis of appearance and flavour, and were canned (100 ml). All CGA isomers are presented according to the IUPAC nomenclature( 9 ). The test beverage contained 0 or 600 mg of CGA, which consisted of CQA (68 %), FQA (14 %) and diCQA (19 %) (Table 1). CGA were prepared from green coffee beans using hot water extraction followed by spray drying and girding. After activated carbon filtration, caffeine was not detected (<0·5 mM). The CGA composition in test beverages was measured by HPLC with a UV detector (325 nm). All peaks of the isomers were confirmed by LC-MS( Reference Matsui, Nakamura and Kondou 34 ). The standard, 5-CQA, was purchased from Sigma, and other standards, 3-CQA, 4-CQA, 3-FQA, 4-FQA, 5-FQA, 3,4-diCQA, diCQA and 3,5-diCQA, were obtained by repeated fractionations of green coffee bean extract (purity >96 %, confirmed by LC-UV detection and 1H-NMR) using preparative chromatography with an octa decyl silyl (ODS) column( Reference Matsui, Nakamura and Kondou 34 ). Both beverages were caffeine-free, and the energy contents were 29 kJ/100 ml (7 kcal/100 ml) and 8 kJ/100 ml (2 kcal/100 ml) in the CGA and placebo beverages, respectively.
Table 1 Chlorogenic acid (CGA) compositions of the test beverages (mg/100 ml)
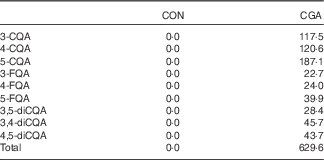
CON, control; CQA, caffeoylquinic acid; FQA, feruloylquinic acid; diCQA, dicaffeoylquinic acid.
Sleep recording
The recording system consisted of four electroencephalography derivations (C3-A2, C4-A1, O2-A1, and O1-A2), submental electromyography and bilateral electro-oculography using a PSG-1100 (Nihon Kohden). Sleep parameters were classified in 30-s intervals as wakefulness and stage 1, stage 2, slow-wave sleep (SWS), and rapid eye movement (REM) sleep according to the method developed by Rechtschaffen & Kales( Reference Rechtschaffen and Kales 35 ). In addition, total sleep time, sleep onset latency, REM sleep latency and sleep efficiency percentages were measured.
Data analysis: spectral analysis of the electroencephalogram
The C3-A2 electroencephalogram (EEG) recording was analysed using discrete fast Fourier transform techniques. The fast Fourier transform was conducted on an EEG record length of 5 s to obtain a frequency resolution of 0·2 Hz. Each 5-s segment of the EEG signal was first windowed with a Hanning tapering window before computing the power spectra. The spectral distribution was categorised into the following frequency bands: delta (0·75–4·00 Hz), theta (4·10–8·00 Hz), alpha (8·10–12·00 Hz), sigma (12·10–14·00 Hz) and beta (14·10–30·00 Hz). The power content of the delta band for each 30-s epoch of sleep was determined as the average power across the six 5-s segments of the EEG (expressed as µV2)( Reference Zhang, Samet and Caffo 36 ).
Indirect calorimetry
The airtight metabolic chamber measures 2·00×3·45×2·10 m (FHC-15S; Fuji Medical Science Co., Ltd), and was used from day 5, 4 h before bedtime, to day 6, 5 h after waking (total, 16 h). Air in the chamber is pumped out at a rate of 80 litres/min. The temperature and relative humidity of incoming fresh air were controlled at 25°C and 55 %, respectively. The chamber was furnished with an adjustable hospital bed, desk, chair and toilet. Concentrations of O2 and carbon dioxide (CO2) in outgoing air were measured with high precision by online process MS (VG Prima δB; Thermo Electron Co.). The precision of MS, defined as the standard deviation for continuous measurement of the calibrated gas mixture (O2, 15 %; CO2, 5 %), was 0·0016 % for O2 and 0·0011 % for CO2. Every minute, VO2 and CO2 production (VCO2) rates were calculated using an algorithm for improved transient response( Reference Tokuyama, Ogata and Katayose 37 ). Oxidation of macronutrients and energy expenditure were calculated from VO2, VCO2 and urinary N excretion( Reference Ferrannini 38 ). Rates of N, an index of protein oxidation, were assumed to be constant during calorimetry:
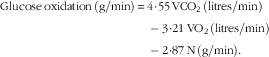
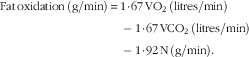

Once the rates of glucose, fat and protein oxidation had been computed, the total rate of energy production could be estimated by taking energetic equivalents of the three substrates into account. Conversion factors for energetic equivalents were 17·15 kJ/g (4·10 kcal/g) for protein (107·215 kJ/g (25·625 kcal/g) for urinary N), 15·65 kJ/g (3·74 kcal/g) for carbohydrates and 39·75 kJ/g (9·50 kcal/g) for fat( Reference Ferrannini 38 ).
Autonomic nervous system activity
The R-R intervals of the electrocardiogram were continuously monitored using a telemetric heart-rate monitor (LX−3230, 207; Fukuda Denshi Co., Ltd) and the power spectrum of heart-rate variability was estimated using the maximum entropy method. The spectra measured were computed as amplitudes (i.e. areas under the power spectra) and are presented in ms2. Parasympathetic and sympathetic nervous system activities were estimated at a high frequency (HF; 0·15−0·4 Hz) and as the power ratio of low frequency (LF; 0·04−0·15 Hz):high frequency (LF:HF), respectively( Reference Stein and Kleiger 39 ).
Statistical analysis
Results are expressed as means with their standard errors. Sample size was calculated on the basis of a power analysis using previous data. A study group of nine subjects was required for a power of 80 % at a two-sided α of 0·05. We performed a power analysis; the actual power was >80 % for each comparison. Paired t tests were used to compare values of energy metabolism, sleep parameters and autonomic nervous system activity between the groups. The effects of CGA on the time course of autonomic nervous system activity were assessed by a two-way repeated measures ANOVA and a Bonferroni multiple comparison. Differences were considered significant when the error probability was <0·05.
Results
Body weight, BMI and body fat did not change during the 5-d trial with control and CGA beverages (Table 2). Sleep architecture (lengths of stage 1, stage 2, SWS, REM and wakefulness after sleep onset) was similar between the trials, except that sleep latency was shorter in trials with ingestion of CGA (Table 3). The time course of delta power, a quantitative index of SWS, was similar between the two trials, but those during the 1st hour of sleep during CGA trials tended to be higher than those during CON trials. A two-factor repeated measures ANOVA identified a significant interaction effect. The average delta power during the 1st hour (CON: 29 165 (sem 5711) µV2 v. CGA: 40 758 (sem 5128) µV2, P=0·08) seemed to be higher in the CGA trials than in the CON trials, but no statistically significant difference was found in multiple comparisons (Fig. 1).
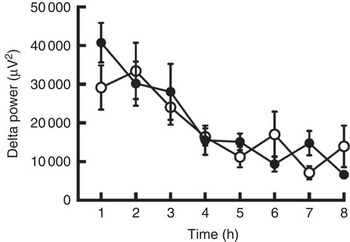
Fig. 1 The delta power of the sleep electroencephalogram after 5 d of ingestion of the chlorogenic acids beverage (●) or placebo beverage (○) is shown. Values are means (n 9 per group), with standard errors.
Table 2 Anthropometric variables before and after ingesting either the chlorogenic acids (CGA) or placebo beverage for 5 dFootnote * (Mean values with their standard errors)
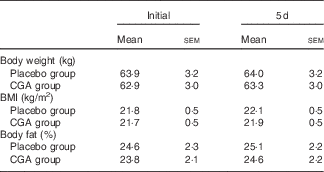
* Mean values did not differ significantly between the groups.
Table 3 Sleep architecture(Mean values with their standard errors)
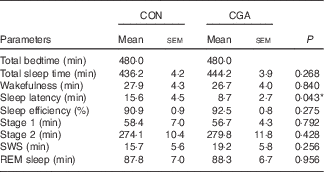
CON, control; CGA, chlorogenic acids; SWS, slow-wave sleep; REM, rapid eye movement.
* P<0·05.
The average sympathetic nervous system activity during 16 h of calorimetry was similar (CON: 2·20 (sem 0·09) v. CGA: 2·36 (sem 0·16)), but parasympathetic nervous system activity was enhanced by CGA consumption (CON: 919 (sem 55) ms2 v. CGA: 999 (sem 78) ms2, P<0·05). Visual inspection of the time course of autonomic nervous system activity suggests that parasympathetic activity and sympathetic activity increased during the second half of sleep and after awakening, respectively, by CGA consumption (Fig. 2). However, a statistically significant difference was not detected by a two-factor repeated measures ANOVA.
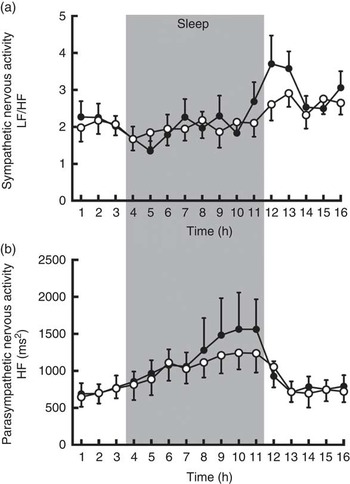
Fig. 2 Sympathetic (a) and parasympathetic (b) nervous system activities during calorimetry after 5 d of ingestion of the chlorogenic acids beverage (●) or placebo beverage (○) are shown. Values are means (n 9 per group), with standard errors. LF, low frequency, HF, high frequency.
Fat oxidation during sleep (8 h) was higher in trials with chlorogenic ingestion than in control trials (Fig. 3). The difference in fat oxidation was also significant when entire 16-h calorimetry periods were compared (CON: 638·5 (sem 118·4) kJ/16 h (152·6 (sem 28·3) kcal/16 h) v. CGA: 958·1 (sem 152·7) kJ/16 h (229·0 (sem 36·5) kcal/16 h), P<0·05). Energy expenditure was similar under both conditions, and the difference in carbohydrate oxidation was not statistically significant. Urinary N excretion as a marker of protein catabolism was similar between the two experimental conditions.
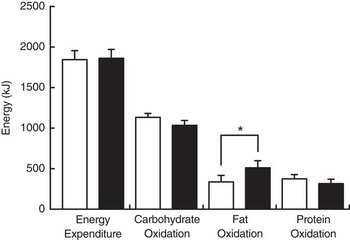
Fig. 3 Energy expenditure and substrate oxidation during sleep after 5 d of ingestion of the chlorogenic acids beverage (■) or placebo beverage (□). Values are means (n 8 per group), with standard errors represented by vertical bars. *P<0·05.
Discussion
In the present study, we assessed whether ingestion of beverages containing CGA for over 5 d had any effect on sleep, autonomic nervous system activity or energy metabolism in healthy humans. Our findings revealed that CGA shortened sleep latency as opposed to the controls, whereas no effect on sleep architecture such as SWS, REM or waking after sleep onset was observed. Indirect calorimetry revealed that consumption of CGA increased fat oxidation but did not affect energy expenditure during sleep. Consumption of CGA enhanced parasympathetic activity, which was assessed on the basis of heart-rate variability. These results support the possibility that beverages containing CGA possess beneficial effects to prevent obesity and improve sleep.
Fat oxidation during sleep was increased by consumption of CGA. An animal experiment reported an inhibitory effect of the green coffee bean extract, which is rich in CGA and its related compounds, on visceral fat accumulation and body weight gain in mice. CGA and its related compounds up-regulated carnitine palmitoyltransferase activity in the liver( Reference Shimoda, Seki and Aitani 40 ). Using continuous and prolonged indirect calorimetry, the present study confirmed the findings of a previous report on use of indirect calorimetry for a short duration (3·5 h), which showed increased fat oxidation after ingestion of CGA (359 mg)( Reference Noriyasu, Satoko and Takatoshi 41 ). These indirect calorimetry findings are consistent with another human study reporting that daily consumption of coffee enriched with CGA for 12 weeks resulted in body weight reduction( Reference Thom 42 ).
The effect of CGA in stimulating fat oxidation during sleep was manifested without an adverse effect on sleep architecture, which is in contrast with the effects of sympathomimetics such as capsaicin, catechins and caffeine. Capsaicin, in addition to stimulating epinephrine secretion, acts as a transient receptor potential cation channel subfamily V member 1 agonist( Reference Edwards, Montgomery and Colquhoun 43 ) and disturbs sleep via changes in body temperature. Caffeine, in addition to inhibiting the phosphodiesterase-induced degradation of intracellular cyclic AMP, acts as an adenosine A2A receptor antagonist and increases wakefulness( Reference Huang, Qu and Eguchi 44 ). Effects of catechins, which inhibit norepinephrine degradation, on sleep remain to be evaluated. A potential mediator of CGA that can shorten sleep latency is ferulic acid, which is a metabolite of CGA; ferulic acid also potentiates pentobarbital-induced sleep in mice by prolonging sleeping time and shortening sleep latency in a dose-dependent manner( Reference Tu, Cheng and Sun 32 ). At 1-2 h after the oral ingestion of CGA, they are metabolised via methylation and appear as ferulic acids in plasma( Reference Matsui, Nakamura and Kondou 34 , Reference Renouf, Guy and Marmet 45 ). Contrary to the present human study, sleep latency in rats was significantly increased by ingestion of CGA (CGA, 500 mg/kg and caffeic acid, 200 mg/kg)( Reference Shinomiya, Omichi and Ohnishi 46 ). The dose of CGA in the animal experiment was several orders of magnitude greater than that in the present human study. Human subjects, as opposed to experimental animals, were exposed to CGA via daily consumption of coffee and vegetables.
The function of the autonomic nervous system was also affected by ingestion of CGA through up-regulation of parasympathetic nervous system activity and a decrease in heart rate. An increase in parasympathetic nervous system activity may explain the antihypertensive effects of CGA, for which several mechanisms have been proposed: reduction of free radical production, scavenging free radicals, stimulation of NO production and inhibition of angiotensin-converting enzyme( Reference Zhao, Wang and Ballevre 47 ). In addition to vasodilation effects in peripheral tissue, NO acts as a neuromodulator within the central and peripheral nervous systems, and modulates sympathetic and parasympathetic activities( Reference Zanzinger 48 ). Close inspection of the time courses of sympathetic nervous system activity revealed the following. First, before and during the first 3 h of sleep, activities of the sympathetic and parasympathetic nervous systems were similar between the trials. The mechanism by which ingestion of CGA shortened sleep latency cannot be explained by the effect of chlorogenic ingestion on autonomic nervous system activity. Second, relative to that in the placebo-control trial, parasympathetic activity was higher during the second half of sleep, whereas sympathetic activity was higher after awakening in the morning in trials with ingestion of CGA. Thus, ingestion of CGA induced a greater decrease in parasympathetic activity and increase in sympathetic activity after awakening.
In conclusion, ingestion of CGA stimulated fat oxidation without an adverse effect on sleep architecture; rather, it shortened sleep latency. Ingestion of CGA increased parasympathetic activity during sleep, and the causal relation to its effects on sleep and fat oxidation remains to be evaluated. To generalise the present findings, an experiment with a larger sample size of obese and/or aged subjects should be performed.
Acknowledgements
We appreciate the technical support from Fuji Medical Science (Chiba, Japan) and Thermo Fisher Scientific (Winsford, UK).
I. P., M. H., M. S., and K. T. designed the study and edited the manuscript. S. H. provisioned study materials (test beverages). I. P. performed indirect calorimetry and M. K., H. O. and M. S. performed polysomnography (PSG) analyses of sleep. R. O. and Y. K. contributed to the ‘Discussion’ section and reviewed the manuscript.
M. H., R. O., S. H. and Y. K. are employees of the Kao Corporation. The authors declare that there are no conflicts of interest.