Introduction
The Himalaya comprise one of the largest collections of glaciers outside the polar regions, with a total glacier cover of ∼33 000 km2 (Reference Dyurgerov and MeierDyurgerov and Meier, 1997) and a total number of ∼9600 glaciers in the Indian Himalaya (Reference Raina and SrivastavaRaina and Srivastava, 2008). Himalayan glaciers are of interest for several reasons. Seasonal and monsoon precipitation along with snow and ice melt concomitantly governs flow regimes of Himalayan rivers. Water discharge from Himalayan glaciers contributes to the overall river runoff (Reference Immerzeel, van Beeke and BierkensImmerzeel and others, 2010). For instance, on average the annual snow and glacier melt contribution in the Ganga basin is estimated to be 97% at Bhojbasa (∼3780 m a.s.l.) near the terminus of Gangotri Glacier (Reference Singh, Haritashya and KumarSingh and others, 2008), ∼71% at Loharinag-Pala (∼2100 m a.s.l.; Reference Arora, Rathore, Singh, Kumar and KumarArora and others, 2010) and 29% at Devprayag (∼830 m a.s.l.; Reference Singh, Jain, Kumar and SinghSingh and others, 1993, Reference Singh, Polglase, Wilson, Jain, Singh, Kumar, Kumar, Singh and Sharma2009) (Fig. 1). One recent field study (Reference Thayyen and GerganThayyen and Gergan, 2010) indicates that observed flow variations in the Din Gad catchment (Bhagirathi basin, Garhwal Himalaya) is correlated with precipitation rather than glacier melt. Nevertheless, water discharge from Himalayan glaciers is important for irrigation and hydropower generation (Reference Singh, Polglase, Wilson, Jain, Singh, Kumar, Kumar, Singh and SharmaSingh and others, 2009). In addition, Himalayan glacier change is likely to contribute significantly to sea-level change (Reference Dyurgerov and MeierDyurgerov and Meier, 2005).

Fig. 1. (a) Location of study area in the Himalaya with major river systems of the Indian subcontinent. (b) Glacier coverage in the upper Bhagirathi and Saraswati/Alaknanda basins, based on ASTER (11 October 2006) imagery; stars represent glaciers mapped from Landsat Thematic Mapper (TM) (15 November 1990)
Historic instrumental weather records from the northwestern Himalayan region have shown an increase in temperature of ∼1.6°C over the past century, accompanied by a decreasing trend in monsoon precipitation (Reference Bhutiyani, Kale and PawarBhutiyani and others, 2007, Reference Bhutiyani, Kale and Pawar2009). Himalayan glaciers have been in a general state of recession since the 1850s (Mayewski and Jeschke, 1979; Reference Bhambri and BolchBhambri and Bolch, 2009). Existing studies of Himalayan glaciers indicate that many have exhibited an increased receding trend over the past few decades (Reference Kulkarni, Rathore, Mahajan and MathurKulkarni and others, 2005, Reference Kulkarni2007; Reference Bolch, Buchroithner, Pieczonka and KunertBolch and others, 2008; Reference ChaujarChaujar, 2009). However, some glaciers (e.g. Raikot Glacier in Nanga Parbat, Punjab Himalaya) have been stable during recent decades (Reference Schmidt and NüsserSchmidt and Nüsser, 2009) and others have advanced (e.g. tributaries of Panmah and Liligo Glaciers in the Karakoram region of Pakistan) (Reference HewittHewitt, 2005; Reference Belò, Mayer, Smiraglia and TamburiniBelò and others, 2008). Individual local glacier advances have also been reported in the Greater Himalayan Range of Ladakh (Reference Kamp, Byrne and BolchKamp and others, 2011). This advance could be attributed to surging (Reference HewittHewitt, 2005, Reference Hewitt2007). Advances may also be associated with a climate trend to increased precipitation in accumulation areas in recent decades (Reference Fowler and ArcherFowler and Archer, 2006). Generally, the regional irregularity of Himalayan glacier variability could be explained by differences in monsoonal intensity (Reference KargelKargel and others, 2005), characteristics and thickness of supraglacial debris cover (Reference Bolch, Buchroithner, Pieczonka and KunertBolch and others, 2008; Scherler and others, in press), altitude of accumulation zone and size of glaciers (Reference KulkarniKulkarni and others, 2007) and contributions from tributary glaciers in accumulation zones (Reference Nainwal, Negi, Chaudhary, Sajwan and GauravNainwal and others, 2008b).
Thus, regular monitoring of a large number of Himalayan glaciers is important for improving our knowledge of glacier response to climate change. Although field investigations are highly recommended, only a limited number of glaciers can be investigated owing to time and logistical constraints in remote mountain regions. Hence, glacier mass-balance studies including field measurements have been reported for only a few glaciers, such as Dokriani Glacier (1992–1995, 1997–2000 and 2007–present) and Chorabari Glacier (2003–present) in the Garhwal Himalaya (Reference DobhalDobhal, 2009; Reference Dobhal and MehtaDobhal and Mehta, 2010) and Chhota Shigri Glacier in Himachal Himalaya (Reference WagnonWagnon and others, 2007).
Multitemporal and multispectral satellite data provide abundant potential for mapping and monitoring the large spatial coverage of glaciers at regular temporal intervals, as they allow automated/semi-automated glacier mapping (Reference PaulPaul and others, 2009; Reference Racoviteanu, Paul, Raup, Khalsa and ArmstrongRacoviteanu and others, 2009; Reference Bolch, Menounos and WheateBolch and others, 2010b). Most of the glacier change studies from the Indian Himalaya are based on a comparison of satellite data with 1960s topographic maps. However, several studies have reported that mapping of glacier areas on Survey of India (SOI) topographical maps has serious accuracy issues (Reference VohraVohra, 1980; Reference Raina and SrivastavaRaina and Srivastava, 2008; Reference Bhambri and BolchBhambri and Bolch, 2009; Reference RainaRaina, 2009). Declassified imagery from the 1960s and 1970s (e.g. Corona and Hexagon) is ideal for mapping historic extents of glaciers (Reference Bolch, Buchroithner, Pieczonka and KunertBolch and others, 2008; Reference Narama, Kääb, Duishonakunov and AbdrakhmatovNarama and others, 2010) and can be used for comparison with glacier outlines derived from old topographic maps (Reference Bhambri and BolchBhambri and Bolch, 2009; Reference BolchBolch and others, 2010a).
Several mapping and monitoring studies exist for Gangotri Glacier, the largest glacier in the Garhwal Himalaya (Reference Chaujar, Mazari and GerganChaujar and others, 1993; Reference SrivastavaSrivastava, 2004; Reference Bahuguna, Kulkarni, Nayak, Rathore, Negi and MatherBahuguna and others, 2007; Reference Kumar, Dumka, Miral, Satyal and PantKumar and others, 2008; Reference Bhambri and ChaujarBhambri and Chaujar, 2009) and a few studies have been published on mapping and variability of selected glaciers of the adjacent Saraswati/Alaknanda basin (e.g. Reference Nainwal, Sajwan, Bahuguna and KulkarniNainwal and others, 2008a, Reference Nainwal, Negi, Chaudhary, Sajwan and Gauravb). However, to our knowledge there are no published studies addressing glacier changes and influencing variables, such as debris cover, topography and climate parameters, for the larger glaciated region of the Garhwal Himalaya. In addition, no studies exist that use declassified imagery. Thus, the main goals of this study are to: (1) generate a current glacier inventory for the Garhwal Himalaya using Advanced Spaceborne Thermal Emission and Reflection Radiometer (ASTER) imagery and provide information on the general glacier characteristics; (2) analyze recent glacier area changes in the upper Bhagirathi and Saraswati/ Alaknanda sub-basins; and (3) evaluate air temperature and precipitation tendency.
Study Area
The Garhwal Himalaya are part of the Uttarakhand state of India, close to China. Based on the glacier atlas of India, 968 glaciers exist in the Garhwal Himalaya, covering ∼2885 km2 or ∼5.3% of the state (Srivastava, 2008). Our research area covers two sub-basins of the Ganga River in the Garhwal Himalaya: the upper Bhagirathi basin and the Saraswati/ Alaknanda basin. The Bhagirathi River is the main source stream of the Ganga River, which originates from the snout (Gaumukh; ∼3950 m a.s.l.) of Gangotri Glacier, the largest valley glacier (∼30 km) in the Garhwal Himalaya. The headwater of the Alaknanda River originates from the snouts of Bhagirath Kharak and Satopanth Glaciers. The Alaknanda basin has ∼407 glaciers covering ∼1229 km2, whereas the Bhagirathi basin contains ∼238 glaciers covering ∼755 km2 (Reference Raina and SrivastavaRaina and Srivastava, 2008). The study area of the upper Bhagirathi basin covers ∼700 km2 and an elevation range ∼3000–7000 m a.s.l. (Fig. 1). Gangotri Glacier originates in the Chaukhamba massif (∼6853–7138 m a.s.l.) and flows northwest towards Gaumukh. The equilibrium-line altitude (ELA) of Gangotri Glacier is ∼4875 m a.s.l. (Reference Ahmad and HasnainAhmad and Hasnain, 2004). The study area of the Saraswati/Alaknanda basin extends from Mana Pass to Badrinath Temple including Arwa Valley and the Western Kamet Group. It occupies ∼970 km2 with an elevation range of ∼3100–7756 m a.s.l. at Mount Kamet (Fig. 1). The Pawegarh Ridge (5288–6165 m a.s.l.) is a divide between the Alaknanda and Saraswati catchments (Reference Nainwal, Negi, Chaudhary, Sajwan and GauravNainwal and others, 2008b).
Garhwal Himalayan glaciers are fed by summer monsoon and winter snow regimes (Reference Thayyen and GerganThayyen and Gergan, 2010). However, maximum snowfall occurs from December to March, mostly due to western disturbances (Reference Dobhal, Gergan and ThayyenDobhal and others, 2008). At Mukhim station (∼1900 m a.s.l.; ∼70 km from the snout of Gangotri Glacier) the mean annual air temperature (MAAT) is 15.4°C and the annual precipitation is 1648 mm (Fig. 2). In December 1999, the Snow and Avalanche Study Establishment (SASE) established a manned meteorological observatory at Bhojbasa (∼3780 m a.s.l.) and an automatic weather station (AWS) ∼4 km from the snout of Gangotri Glacier (http://www.dst.gov.in/about_us/ar04–05himalayan.htm). From 2000 to 2008, average annual maximum (T MAX) and minimum (T MIN) temperatures at Bhojbasa were 11.0°C and −2.3°C, respectively, and average winter (DJ F) snowfall was 546 mm.

Fig. 2. Climate data for (a) Mukhim (∼1900 m a.s.l.; MAAT 15.4°C, annual precipitation 1648 mm, 1971–2000) and (b) Bhojbasa stations (∼3780 m a.s.l., 4.4°C; 2001–09) (see Fig. 1 for location). Data sources: Indian Meteorological Department (IMD) and Snow and Avalanche Study Establishment (SASE).
Field surveys and assessment of satellite images indicate that most glaciers in the study area are covered with debris in the ablation zone (e.g. Gangotri Glacier). The debris in upper Bhagirathi basin consists mainly of granite, granitic gneiss and sheared granitic gneiss (Reference Chaujar, Mazari and GerganChaujar and others, 1993). Knowledge of glacier changes and debris cover in the neighboring Bhagirathi and Saraswati/Alaknanda basins is limited.
Methods
Data sources
Three Corona KH-4A images (footprint ∼17 km × 233 km) from 27 September 1968, with minimal snow and cloud cover, were used to extract the historic extent of glaciers in the study area. A digital terrain model (DTM) was constructed from ASTER stereo images (2006) at horizontal grid spacing of 30 m. The DTM had a vertical accuracy of ±21 m (Reference Bhambri and BolchBhambri and Bolch, 2009; Reference Bhambri, Bolch and ChaujarBhambri and others, in press), similar to that of ASTER DTMs in other rugged terrain such as the Swiss Alps and the Andes (Reference KääbKääb, 2002; Reference Racoviteanu, Manley, Arnaud and WilliamsRacoviteanu and others, 2007).
Orthorectified 2006 high-resolution Cartosat-1 and multi-spectral Resourcesat-1 (IRS-P6) Linear Imaging Self-Scanning sensor (LISS) IV images, generated for a previous study (Reference Bhambri, Bolch and ChaujarBhambri and others, in press), were used to extract and evaluate recent glacier outlines (Table 1). Cartosat-1 data with 10 bit radiometric resolution covered the entire debris-covered area of Gangotri Glacier but only parts of Raktavan and Chaturangi Glaciers due to their limited swath (∼27 km); the data allowed for the interpretation of complex polygenetic glacier landscapes. 1976 Landsat multispectral scanner (MSS) and 1990 Landsat thematic mapper (TM) images were used to obtain additional information for glacier mapping and glacier change analysis (Table 1).
Table 1. Details of satellite data

Monthly average T MAX and T MIN and precipitation data for Mukhim station from 1957 to 2005 (missing years: 1962, 1963, 1967, 1983 and 1984) were acquired from the Indian Meteorological Department (IMD; Fig. 2a). Monthly data were used to obtain average indices for annual and seasonal (December, January, February: DJF; June, July, August: JJA) values of temperature (T MIN and T MAX) and precipitation. Data from Tehri and Mukhim stations have an overlap of 22 years. Mean monthly climate data (temperature and snowfall) for Bhojbasa station from 1999 to 2008 were acquired from SASE. A nonparametric Mann–Kendall test was used to determine statistical significance trend, and magnitudes of the trend were obtained by linear regression analysis (Reference Racoviteanu, Arnaud, Williams and OrdoñezRacoviteanu and others, 2008; Reference Sansigolo and KayanoSansigolo and Kayano, 2010). A correlation analysis was carried out to verify trend results from Mukhim station and to determine whether it is possible to transfer data from Mukhim station (longer time series) to Bhojbasa station (shorter time series).
Rectification of Corona satellite images
Individual Corona strips were merged using the unregistered mosaic module of PCI Geomatica 10.1. Owing to difficult geometry of the Corona imagery, we generated 26 Corona subsets for the Saraswati/Alaknanda basin and eight subsets for the upper Bhagirathi basin. All subsets were co-registered based on a two-step approach: (1) a projective transformation was performed based on ground-control points (GCPs) and the ASTER digital elevation model (DEM) using ERDAS Imagine 9.3; followed by (2) a spline adjustment using ESRI ArcGIS 9.3. Stable river junctions and road stream junctions were used to collect GCPs assuming no changes occurred on the ground. For each Corona subset, 30–255 GCPs were acquired from ASTER imagery for co-registration (Fig. 3). We focused on the adjustment of the area around the glaciers on Corona images in respect of ASTER imagery for consistency of results during rectification of Corona imagery. It was assumed that no change occurred at the upper glacier boundaries during the time covered by the study. To assess positional accuracy, 20 common location points such as river junctions were identified in both ASTER images and Corona subsets; the horizontal shift between both images was 11 m (1.4 pixels). Landsat MSS and TM images were co-registered with the ASTER DEM and ASTER imagery using the projective transformation algorithm. Horizontal shifts of ∼40 m and ∼20 m, respectively, were found.

Fig. 3. Satellite images of Tara Bamak Glacier, Saraswati/Alaknanda basin (see Fig. 1 for location). (a) Rectified subset of Corona image (27 September 1968) based on spline method with similar-year glacier outline. (b) ASTER image (11 October 2006) with ASTER and Corona glacier outlines.
Glacier mapping
We used the modified classification of the Global Land Ice Measurements from Space (GLIMS) initiative (www.glims.org/MapsAndDocs/guides.html) to define glacierized areas, and excluded ice parts above the bergschrund because they only occasionally and indirectly contribute snow and ice to the glacier mass through avalanches and creep flow. Previous studies have shown that normalized-difference snow index (NDSI) and band ratio methods could not differentiate debris-covered glacier ice from surrounding moraines/rocky surface due to similar spectral signatures (Reference Bolch, Buchroithner, Kunert, Kamp and GomarascaBolch and others, 2007). However, when compared with manual delineation, thresholding of NDSI and band ratio methods are time-efficient and robust approaches for mapping clean glacier ice (Reference RottRott, 1994; Reference Hall, Riggs and SalomonsonHall and others, 1995; Reference Paul, Kääb, Maisch, Kellenberger and HaeberliPaul and others, 2002; Reference Bolch, Kamp, Kaufmann and SulzerBolch and Kamp, 2006; Reference Racoviteanu, Arnaud, Williams and OrdoñezRacoviteanu and others, 2008, Reference Racoviteanu, Paul, Raup, Khalsa and Armstrong2009). It has been reported that for shaded areas with thin debris cover the band ratio near-infrared/ shortwave infrared (NIR/SWIR) performs better than red/ SWIR and NDSI (Reference Paul and KääbPaul and Kääb, 2005; Reference Andreassen, Paul, Kääb and HausbergAndreassen and others, 2008). In our study, several image band ratios (1/3; 3/4), NDSI (1−4/1+4) and classifications were tested using ASTER imagery. Band ratio NIR/SWIR was most suitable for mapping clean glacier ice. The clean glacier-ice mask was generated in a binary image using threshold values of 1.0 for ASTER and 2.0 for TM imagery, and the binary image was then converted into vector format. Misclassified glacier areas such as water bodies, shadows and isolated rocks were eliminated manually. Morphometric and thermal band information was used for mapping debris-covered glaciers (Reference Bhambri, Bolch and ChaujarBhambri and others, in press) to assist the manual delineation based on ASTER imagery and the ASTER DTM (Reference Racoviteanu, Arnaud, Williams and OrdoñezRacoviteanu and others, 2008; Reference Bolch, Menounos and WheateBolch and others, 2010b). We found that the separation of stagnant glacier ice from active debris-covered ice is an enormous challenge in glacier terminus mapping. Therefore, Cartosat-1 and LISS IV band combination 2–3–4 were used to improve the debris-covered glacier outlines derived from ASTER imagery. Several characteristics such as supraglacial ponds or creeks were used to determine the most likely position of the termini. Cartosat-1 and LISS IV data were also used to evaluate the clean-ice glacier polygons derived from ASTER imagery (Table 2). The 1968 glacier extents were manually delineated from Corona images. Small high-altitude glaciers that were mapped from Corona images were not included in the comparison with glacier outlines derived from the ASTER imagery, because close to mountain ridges significant errors occurred during co-registration. The minimum glacier size we took into account for comparison was 0.17 km2 in 1968. The ice divides were visually identified using the shaded ASTER DTM and ASTER bands 3–2–1 and then manually digitized (Reference Bhambri, Bolch and ChaujarBhambri and others, in press). It was assumed that the divides were fixed over the time under investigation. This approach avoids errors that may occur due to different delineation of the upper glacier boundary, for example different snow conditions (Fig. 3). Landsat MSS and TM scenes were only partially suitable for glacier mapping due to large shadows and fresh snow cover. Hence, from Landsat TM imagery we could only map 29 glaciers in the upper Bhagirathi and Saraswati/Alaknanda basins (Fig. 1). MSS data were mainly used to assist in identifying glacier terminus positions from Corona images.
Table 2. Comparison between glacier areas derived from ASTER (11 October 2006) and LISS IV sensor (1 October 2006)

Topographic information was computed from the ASTER DTM using Geographic Information System (GIS) tools as suggested by Reference PaulPaul and others (2009). We investigated the characteristics of glacier distribution in the study region by statistically analyzing the relations between topographic parameters and glacier polygons. Glacier changes between 1968 and 2006 could be compared for 69 glaciers in the Saraswati/Alaknanda basin and for 13 glaciers in the upper Bhagirathi basin.
Mapping uncertainty
Glacier outlines derived from various satellite datasets with different resolutions, obtained at different times with varying snow, cloud and shadow conditions, have different levels of accuracy. Thus, estimation of the uncertainty is crucial in determining the accuracy and significance of the results. Previous studies report a mapping uncertainty of ±2–3% for clean-ice glaciers (Reference Paul, Kääb, Maisch, Kellenberger and HaeberliPaul and others, 2002; Reference Bolch, Kamp, Kaufmann and SulzerBolch and Kamp, 2006). Comparison between the glacier boundaries derived from ASTER (2006) and those derived from high-resolution LISS IV images (2006) for six selected glaciers suggested an uncertainty of ±1.76% (Table 2). Similarly, we estimated an additional uncertainty of ±0.2% for debris-covered glaciers based on comparison between the ASTER outline of the debris-covered area of Gangotri Glacier and the outline derived from the Cartosat-1 image. Thus, the mapping uncertainty is about ±2% for glacier delineation from ASTER imagery. In addition, we estimated the uncertainty for each glacier based on a buffer method suggested by Reference Granshaw and FountainGranshaw and Fountain (2006) and Reference Bolch, Menounos and WheateBolch and others (2010b). The final mapping error, a combination of the mapping uncertainty and the uncertainty of the misregistration, was estimated to be ±3.15%, ±4.0% and ±2.6% for ASTER, Landsat TM and Corona images, respectively. The total error was estimated to be ±4.0% when comparing the data from the ASTER and Corona images. These mapping uncertainties are within the range of previous estimates (Reference Paul, Huggel, Kääb, Kellenberger and MaischPaul and others, 2003; Reference Racoviteanu, Arnaud, Williams and OrdoñezRacoviteanu and others, 2008; Reference BolchBolch and others, 2010a,b).
Results
Glacier characteristics
We identified 83 glaciers in the Saraswati/Alaknanda basin from ASTER images (2006), covering an area of 311.4 ± 9.8 km2 (Table 3). Of the 83 glaciers, 51 had debris-covered tongues, which have an area of 76.7 ± 2.4 km2 (∼24.6% of the total glacierized area). Glacier size ranges from 0.11 to 32.9 km2, with a mean size of 3.7 km2. Some topographic parameters clearly show regional characteristics of glacier distribution. For instance, larger compound basin glaciers tend to extend down to lower elevations, while smaller glaciers have higher termini. On average, glaciers <1 km2 in area terminate at 5308 m and glaciers >5 km2 in area at 4706 m in the Saraswati/Alaknanda basin. There are 43 glaciers with an area less than 1.0 km2, which cover 20.8 ± 0.65 km2 in this basin (Table 3). Median elevation of the glaciers, widely used for the estimation of long-term ELA based on topographic data (Reference Braithwaite and RaperBraithwaite and Raper, 2009), ranges from 4749 to 5896 m, with an average of 5494 m. We found no significant correlation between glacier size and mean/median elevation or aspect in the Saraswati/Alaknanda basin.
Table 3. Derived glacier parameters (2006) for the upper Bhagirathi and Saraswati/Alaknanda basins

The 20 glaciers identified in the upper Bhagirathi basin in 2006 cover an area of 274 ± 8.6 km2. The overall debris-covered area is about 72.6 ± 2.3 km2 (∼26.4% of the whole ice cover). This shows that the upper Bhagirathi basin has a slightly higher debris-covered glacier area than the Saraswati/Alaknanda basin. Glaciers <1 km2 in area terminate at 5178 m, and glaciers >5 km2 in area at 4613 m, on average in the upper Bhagirathi basin. Five glaciers that are <1.0 km2 cover a total area of 2.8 km2. The median elevation of the glaciers ranges from 4712 to 6214 m, with an average of 5636 m (Table 3). Glaciers range in size from 0.21 to 132.7 km2, with a mean of 13.7 km2 in the upper Bhagirathi basin.
On average, glacier termini are 142 m higher in the Saraswati/Alaknanda basin (5105 m) than in the upper Bhagirathi basin (4963 m) (Table 3). Large glaciers (>10 km2) cover ∼83% of the glacial area in the upper Bhagirathi basin and ∼57% in the Saraswati/Alaknanda basin. Small glaciers tend to have smaller altitudinal ranges while larger glaciers have higher altitudinal ranges in both the Saraswati/Alaknanda and upper Bhagirathi basins (r 2 = 0.51) (Fig. 4). The maximum number of glaciers is in the <0.5 km2 class (Fig. 5).
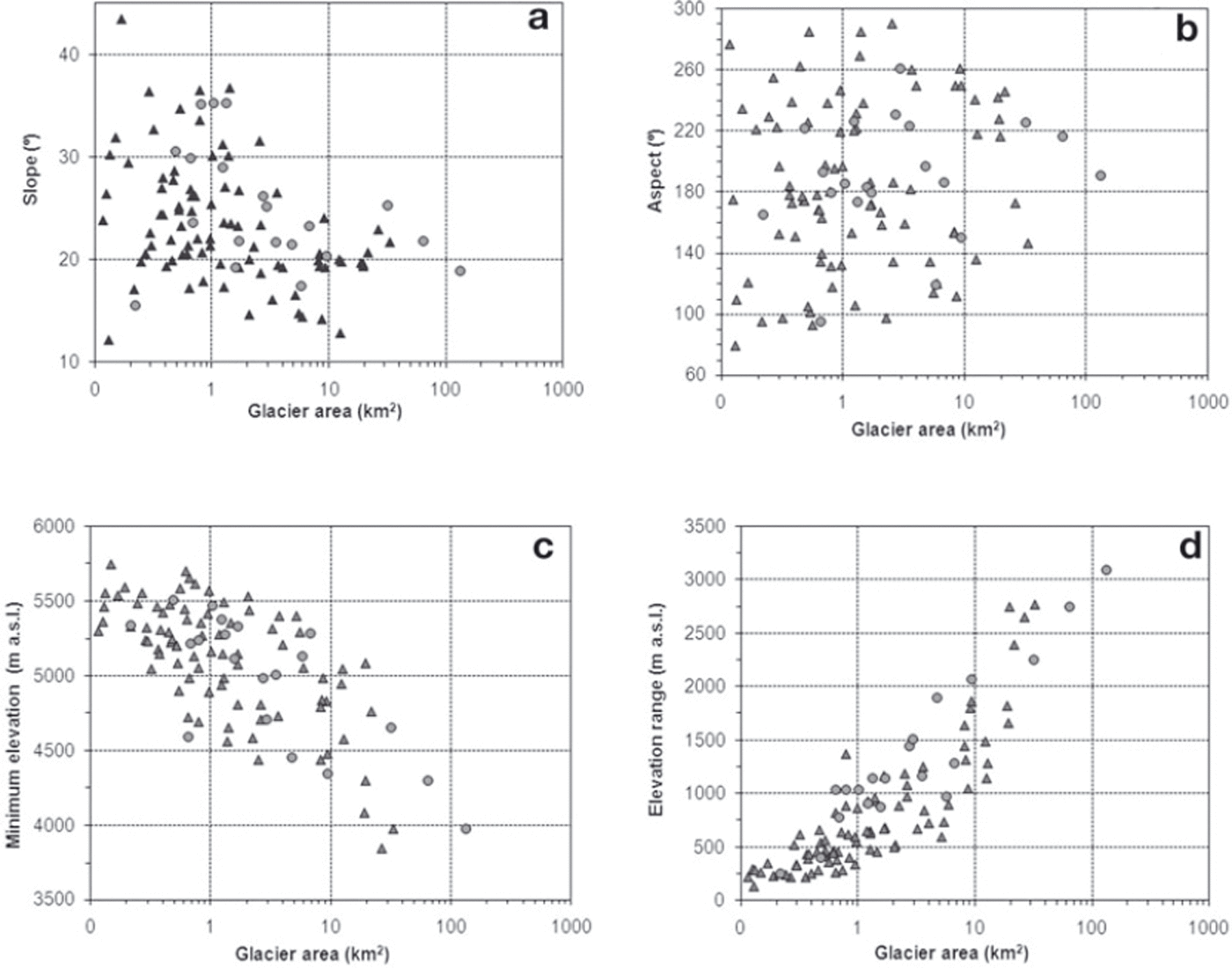
Fig. 4. Scatter plots of (a) glacier area vs slope, (b) glacier area vs aspect, (c) glacier area vs minimum elevation and (d) glacier area vs elevation range. Triangles are for the Saraswati/Alaknanda basin and circles are for the Bhagirathi basin. Glacier area and inventory data derived from ASTER (11 October 2006) image and DEM.

Fig. 5. (a) Area frequency distribution of glaciers for both the Saraswati/Alaknanda and upper Bhagirathi basins derived from analysis of ASTER (11 October 2006) image. (b) Glacier area change and area measurements (%) based on Corona (27 September 1968) and ASTER (11 October 2006) images in both the Saraswati/Alaknanda and upper Bhagirathi basins.
The 29 glaciers mapped from Landsat TM can be seen as a representative subset of all glaciers as they cover different size classes and elevation ranges (Fig. 1). There are 12 glaciers <1.0 km2 in area, covering 7.3 km2 in both the Saraswati/Alaknanda and upper Bhagirathi basins, while the two glaciers >10 km2 in area cover 25.7 km2.
Glacier and climate change
Glaciers have fluctuated noticeably within the study area since 1968 (Table 4). In the Saraswati/Alaknanda basin, the area of the glaciers investigated changed from 324.7 ± 8.4 km2 (1968) to 306.3 ± 9.5 km2 (2006), a decrease of 18.42 ± 9.0 km2 or 5.7 ± 2.7%. The number of glaciers increased from 69 (1968) to 75 (2006) due to the disintegration of ice bodies. The loss in glacier area of individual glaciers ranged from 0.9% to 42.5%. On average, small glaciers (<1 km2) lost ∼19% of their area between 1968 and 2006 in the Saraswati/Alaknanda basin. South-facing glaciers lost ∼19.4% of their area, about four times more than for north-facing glaciers (∼4.7%).
Table 4. Changes in total ice area, clean-ice area and debris-covered ice area in the Garhwal Himalaya between 1968, 1990 and 2006 based on spaceborne imagery

In the upper Bhagirathi basin, the glacierized area changed from 275.1 ± 7.5 km2 (1968) to 266.1 ± 8.3 km2 (2006), a decrease of 8.9 ± 7.9 km2 or 3.3 ± 2.8%. The loss in glacier area ranged from 2% to ∼28% in this basin.
The glacierized area across both basins decreased from 599.9 ± 15.6 km2 (1968) to 572.5 ± 18.0 km2 (2006), a decrease of 27.4 ± 16.8 km2 or 4.6 ± 2.8% (Fig. 6). In both basins, on average over the study period, glaciers with areas <1.0 km2 lost a larger percentage of their area (19.4 ± 2.5% or 0.51 ± 0.07% a−1), than glaciers with areas >50 km2 (2.8 ± 2.7% or 0.074 ± 0.068% a−1) (Fig. 5). For all glaciers, we found strong positive correlation between absolute area change of glaciers and glacier area (r 2 = 0.89) (Fig. 7). This indicates that smaller glaciers are more sensitive to climate change than larger glaciers.

Fig. 6. Glacier change in the Garhwal Himalaya from 1968 to 2006.

Fig. 7. Scatter plots of (a) median elevation vs glacier area change (%), (b) elevation range vs glacier area change (%), (c) glacier area (km2) vs glacier area change (%) and (d) glacier area (km 2)vs absolute glacier area change (km2). Triangles are for the Saraswati/Alaknanda basin and circles are for the Bhagirathi basin. Glacier area change (%) derived from analysis of Corona (27 September 1968) and ASTER (11 October 2006) images.
The 29 mapped glaciers lost 3.48 ± 3.1 km2 (∼3.49 ± 3.1% or 0.15 ± 0.13% a−1) of their area from 1968 to 1990, and 5.67 ± 3.3 km2 (5.89 ± 3.3% or 0.36 ± 0.21% a−1) from 1990 to 2006, in both the upper Bhagirathi and Saraswati/Alaknanda basins; that is, the rate of glacier recession has increased over the past 16 years compared with the previous 22 years. The debris-covered glacier area increased by 17.8 ± 3.1% (0.46 ± 0.08% a−1) in the Saraswati/Alaknanda basin and 11.8% ± 3.0% (0.3 ± 0.08% a−1) in the upper Bhagirathi basin from 1968 to 2006. In total, 45 glaciers were covered with debris in 1968. The number of debris-covered glaciers increased to 56 in 2006.
The annual and JJA T MIN indices from Mukhim station show significant trends (correlation coefficient = −0.50) of −0.072°C a−1 and −0.19°C a−1 between 1957 and 2005, while T MIN for DJF shows no trend. In contrast, the annual (+0.05°C a−1), DJF (+0.1°C a−1) and JJA (+0.06°C a−1) T MAX values display a significant warming trend. There is a slight but not significant decrease in annual precipitation between 1957 and 2005, mainly due to summer precipitation (Fig. 8; Table 5). The mean annual temperature at Mukhim station shows an insignificant decreasing trend (−0.006°Ca−1) over the study period. The JJA T MIN indices for Mukhim and Tehri stations show, for the overlapping 22 years of data, trends of −0.109°C a−1 and −0.088°C a−1, respectively, during 1957–2005. There is no correlation between Bhojbasa and Mukhim climate data for 5 years of overlapping data (2000–05). Meteorological data from Bhojbasa indicate that annual T MIN, T MAX, DJF T MIN and JJA T MAX increased, whereas DJF T MAX and JJA T MIN decreased from 1999 to 2008. Solid precipitation (snowfall) increased during the same period. However, results from Bhojbasa are not statistically significant due to the short time series.

Fig. 8. Climate parameters for Mukhim station: (a) mean T MAX and T MIN for DJF and JJA from 1957 to 2005; (b) mean annual T MAX and T MIN; (c) mean minimum and maximum precipitation for DJF and JJA; and (d) annual mean precipitation. DJF: December, January, February; JJA: June, July, August.
Table 5. Temperature and precipitation trends for the period 1957–2005 for Mukhim station, based on the Mann–Kendall non-parametric test

Discussion
Glacier inventory and changes in the Garhwal Himalaya
We have generated a glacier inventory for 2006 for parts of the Garhwal Himalaya based on digital sources that are time-efficient and largely reproducible (Manley, 2008; Reference Paul and SvobodaPaul and Svoboda, 2009; Reference PaulPaul and others, 2009; Reference Bolch, Menounos and WheateBolch and others, 2010b). The Geological Survey of India (GSI) has compiled a glacier inventory for the selected basins of the Indian Himalaya, including Jhelum, part of Satluj, Bhagirathi, Tista and the entire eastern Himalaya, based on SOI topographic maps, vertical aerial photographs and satellite images, wherever possible with field checks (Reference KaulKaul, 1999). Recent work of the GSI on a comprehensive glacier inventory for entire basins of the Indian Himalaya is relevant and provides a good overview (Reference Raina and SrivastavaRaina and Srivastava, 2008; Reference Sangewar and ShuklaSangewar and Shukla, 2009). The glaciological community will benefit greatly when these data, which are currently assembled in statistical records only (Reference KulkarniKulkarni, 2007; Reference BraithwaiteBraithwaite 2009), are made available in digital GIS format. Information is included concerning topographic parameters (e.g. mean slope, aspect, median and mean elevation) and the percentage of debris cover, which are not available in the Indian glacier inventory in digital GIS format, but are valuable for glaciological and hydrological modeling (Reference BraithwaiteBraithwaite, 2009).
The areal changes of the glaciers investigated in the Garhwal Himalaya confirm an expected and widely published trend of glacier retreat. However, the rate of retreat is less than previously estimated by remote sensing. For example, the present study suggests that Gangotri Glacier shrank by 4.4 ± 2.7 km2 (0.11 ± 0.07 km2 a−1) between 1968 and 2006, whereas Reference Kumar, Areendran and RaoKumar and others (2009) claimed that it reduced by 15.5 km2 (∼0.51 km2 a−1) between 1976 and 2006, and Reference Ahmad and HasnainAhmad and Hasnain (2004) found that it reduced by 10 km2 (∼0.62 km2 a−1) between 1985 and 2001. The main reason for the discrepancy could be that the debris-covered terminus of this glacier is not distinct and snout retreat is obscured. An additional explanation may concern interpretation of debris cover, shadow area and seasonal snow on the coarse satellite images (e.g. Landsat MSS as used by Reference Kumar, Areendran and RaoKumar and others (2009); personal communication from R. Kumar, 2010) and topographic maps (used by Reference Ahmad and HasnainAhmad and Hasnain, 2004), which is known to be one of the major challenges in glacier inventories (Reference Bhambri and BolchBhambri and Bolch, 2009; Reference PaulPaul and others, 2009; Reference Racoviteanu, Paul, Raup, Khalsa and ArmstrongRacoviteanu and others, 2009; Reference BolchBolch and others, 2010a). Reference Kumar, Areendran and RaoKumar and others (2009) reported that Gangotri Glacier area decreased from all sides, a fact that could not be confirmed from our study based on high-resolution Corona and Cartosat-1 data. Our results suggest that Gangotri Glacier lost 0.38 ± 0.03 km2 (0.01 km2 a−1) from its front between 1968 and 2006, based on high-resolution Corona and Cartosat-1 images (Fig. 9). These results are supported by the ground-based plane-table survey by Reference SrivastavaSrivastava (2004). This study indicates that between 1935 and 1996 the area of Gangotri Glacier reduced by 0.58 km2 (∼0.01 km2 a−1) at its terminus. In addition, Reference AudenAuden (1937) reported a single ice cave at the left side of Gangotri Glacier snout. Reference JangpangiJangpangi (1958) noticed two caves, one small and one large, on the terminus of Gangotri Glacier in 1956. However, after 1956 the larger and prominent ice cave had been considered for the recession studies (Reference TiwariTiwari, 1972; Reference SrivastavaSrivastava, 2004). Shadows of ice caves on the 1968 Corona satellite image suggest two caves at the terminus of Gangotri Glacier (Fig. 9). This indicates that high-resolution satellite data possibly provide more reliable results than coarse-resolution satellite data such as Landsat MSS and TM and/or topographic maps, and thus constitute a valuable resource for glacier monitoring.

Fig. 9. Frontal area images of Gangotri Glacier. (a) Corona image (27 September 1968) with same-year glacier outline, (b) Cartosat-1 image (11 October 2006) with glacier outline of 1968 and 2006.
However, inconsistencies in the rectification of Corona images probably are the main source of uncertainty in our estimations of glacier change. The main challenges with Corona data are the complex image geometry and the absence of satellite camera specifications. The adopted spline method for rectification of the imagery in the present study is time-consuming, depends on local knowledge of topography and requires interpreter expertise for collection of GCPs. However, the data are very useful for providing insight into glacier changes since the 1960s, and the above-mentioned field studies (e.g. Reference SrivastavaSrivastava, 2004) support our results for Garhwal Himalayan glaciers. These data are also available for other remote mountain areas where no aerial images are available or accessible. However, existing suitable aerial photographs from the early 1960s from the Indian authorities may provide better insight into glacier change as derived from Corona images in the Garhwal Himalaya.
In other parts of the Garhwal Himalaya, such as the Alaknanda basin, the glacier ice area of Satopanth Glacier shrank by 0.314 km2 (1.5%; 0.007 km2 a−1) near the snout between 1962 and 2006. During a similar period, Bhagirathi Kharak Glacier lost 0.129 km2 (0.4%; 0.002 km2 a−1) (Reference Nainwal, Negi, Chaudhary, Sajwan and GauravNainwal and others, 2008b). Our results, based on Corona and ASTER imagery, suggest that from 1968 to 2006 Satopanth Glacier lost 0.280 km2 (0.007 km2 a−1) near the snout, whereas Bhagirathi Kharak Glacier lost only 0.083 km2 (0.002 km2 a−1). The reason for the minor difference could be the use of the SOI topographic map by Reference Nainwal, Negi, Chaudhary, Sajwan and GauravNainwal and others (2008b). Dokriani Glacier (area ∼7 km2), in the Bhagirathi basin, lost ∼9.5% (0.2% a−1) between 1962 and 2007 (Reference Dobhal and MehtaDobhal and Mehta, 2010). In addition, the GSI has monitored several glaciers based on plane-table survey in Uttarakhand; for example, Pindari Glacier lost 0.111 km2 (0.0026 km2 a−1) at its front from 1958 to 2001 (Oberoi and others, 2001). These field-based studies also support our results.
Comparison between the upper Bhagirathi and Saraswati/Alaknanda basins
The present study gives insight into the differences in the debris-covered and clean-ice areas from 1968 to 2006 in the upper Bhagirathi and Saraswati/Alaknanda basins. Glacier shrinkage in the Saraswati/Alaknanda basin was found to be high compared with the upper Bhagirathi basin. The difference in recession patterns of each glacier may be explained by regional relief (e.g. altitudinal range), quantity and distribution of debris load, orientation or aspect, which vary considerably from glacier to glacier in the upper reaches of these basins. The slower rate of area loss in the upper Bhagirathi basin (−3.3 ± 2.8%) compared with the Saraswati/Alaknanda basin (−5.7 ± 2.7%) from 1968 to 2006 can be explained by: (1) the higher altitudinal range (1352 m) of the glaciers in the upper Bhagirathi basin than in the Saraswati/Alaknanda basin (791 m); (2) the mean glacier size, which is approximately four times higher in the upper Bhagirathi basin (13.7 km2) than in the Saraswati/ Alaknanda basin (3.7 km2); and (3) the three large compound debris-covered glaciers, Gangotri, Raktavan and Chaturangi glaciers, ∼83% of the upper Bhagirathi basin area. Generally, larger compound debris-covered glaciers respond more slowly than small glaciers to perturbations in climate (Reference Bahr, Pfeffer, Sassolas and MeierBahr and others, 1998).
Several studies have reported that debris cover has increased over time in the Himalaya (e.g. Reference Iwata, Aoki, Kadota, Seko and YamaguchiIwata and others, 2000; Reference Bolch, Buchroithner, Pieczonka and KunertBolch and others, 2008; Reference Kamp, Byrne and BolchKamp and others, 2011). We find that debris-covered glacier area increased significantly by 17.8 ± 3.1% (0.46 ± 0.08% a−1) in the Saraswati/ Alaknanda basin and by 11.8 ± 3.0% (0.31 ± 0.08% a−1) in the upper Bhagirathi basin between 1968 and 2006. This supports the assumption that, concomitantly with the higher rate of glacier retreat, the debris-covered area increased more in the Saraswati/Alaknanda basin than in the upper Bhagirathi basin. The reason for the increase needs further investigation as debris cover depends on debris supply and debris transport. However, study of englacial transport can be addressed only marginally using remote-sensing data.
Comparison with other Himalayan regions
The present study indicates that the recession rate of Saraswati/Alaknanda basin glaciers (0.15 ± 0.07% a−1) from 1968 to 2006 is less than reported for other Himalayan regions. For instance, from 1962 to 2001 in the Himachal Himalaya, glaciers in the Chenab basin retreated at ∼0.53% a−1, in Parbati at 0.56% a−1 and in Baspa at ∼0.48% a−1 (Reference KulkarniKulkarni and others, 2007). However, an apparent higher rate of glacier retreat in the Himachal Himalaya could be the result of overestimation of glacier cover in the old datasets due to the use of SOI topographic maps. Previous studies have found some inaccuracies in these maps (Reference VohraVohra, 1980; Reference AgarwalAgarwal, 2001; Reference Raina and SrivastavaRaina and Srivastava, 2008; Reference RainaRaina, 2009). This is most likely in the case of Himachal Himalaya glaciers (e.g. Parbati basin) where aerial photographs were acquired during March–June when snow is not fully melted (Reference Bhambri and BolchBhambri and Bolch, 2009). In this condition, differentiation between snow cover and glacier ice is very difficult and will make glacier delineation on topographic maps problematic.
In the Khumbu Himalaya, Reference Bolch, Buchroithner, Pieczonka and KunertBolch and others (2008) estimated glacier area loss at 5.2% (∼0.12% a−1) based on space imagery from 1962 to 2005. Based on topographic maps, Reference Salerno, Buraschi, Bruccoleri, Tartari and SmiragliaSalerno and others (2008) reported 4.9% (∼0.12% a−1) of the glacier area was lost between the 1950s and 1990s in Sagarmatha National Park, Nepal. Problems encountered in comparing maps, however, were also highlighted. Reference Ye, Zhong, Kang, Stein, Wei and LiuYe and others (2009) reported ∼10.41% (∼0.3% a−1) glacier area loss from 1974 to 2008 at the northern slope of Mount Everest. In contrast, 34 surges have been reported in the Karakoram since the 1860s (Reference HewittHewitt, 2007). Similarly, Reference Raina and SangewarRaina and Sangewar (2007) and Reference Ganjoo and KoulGanjoo and Koul (2009) reported, on the basis of field observation, that Siachen Glacier had shown hardly any retreat in the past 50 years. Raikot Glacier, Nanga Parbat, Punjab Himalaya, showed similar behavior (Reference Schmidt and NüsserSchmidt and Nüsser, 2009). This suggests that the Karakoram area is showing a different response to climate change than other parts of the Himalaya. High reductions in glacierareaof the Himachal Himalaya and surging effects of glaciers of the Karakoram do not appear in the central Himalayan glaciers such as those in the Garhwal and Khumbu Himalaya. Garhwal Himalayan glacier recession rate (∼0.12% a−1) matched that of the Khumbu Himalayan glaciers (∼0.12% a−1; Reference Bolch, Buchroithner, Pieczonka and KunertBolch and others, 2008).
We found that the number of glaciers increased during 1968–2006 due to glacier fragmentation. Similar fragmentation trends have been reported in other glacierized areas of the Himalaya such as in the Himachal region (Reference KulkarniKulkarni, 2007; Reference KulkarniKulkarni and others, 2007). The number of debris-covered glaciers increased in 2006, possibly due to melting of clean-ice surfaces resulting in the exposure of debris-covered areas. Since 1990, the rate of recession has increased in selected glaciers of the Garhwal Himalaya. Similar acceleration in glacier recession has been reported in the Khumbu Himalaya (Reference Bolch, Buchroithner, Pieczonka and KunertBolch and others, 2008).
Our results indicate that glaciers <1 km2 in area lost 19.4 ± 2.5% (∼0.51% a−1) of their area from 1968 to 2006 in both the Bhagirathi and Saraswati/Alaknanda basins, whereas in the Himachal Himalaya glaciers <1 km2 in area lost 38% (∼0.97% a−1) of their area from 1962 to 2001–04 (Reference KulkarniKulkarni and others, 2007). The combined influence of altitudinal distribution and little or no accumulation zone is likely to be the major cause of the rapid recession of the small glaciers. Larger compound basin glaciers are less sensitive to climate change due to contributions from tributary glaciers in accumulation zones and high supraglacial debris cover in the Garhwal Himalaya. North-facing glaciers in the Saraswati/Alaknanda basin were found to recede less than glaciers with other aspects. Generally, northern slopes receive less radiation than the south-facing Himalayan slopes (Reference Ahmad and RaisAhmad and Rais, 1999). Thus north-facing glaciers are expected to react more slowly to climate change than the south-facing glaciers of the Saraswati/ Alaknanda basin. Similar patterns have been reported in previous studies, for example in the Alaknanda basin (Reference Nainwal, Negi, Chaudhary, Sajwan and GauravNainwal and others, 2008b)
Climate set-up and consideration
The Garhwal Himalaya obtain precipitation from the southwest monsoon in summer and from westerlies during the winter season. This climatic regime distinguishes this region from the eastern and Karakoram Himalaya. Our study area (Bhagirathi and Alaknanda catchments) has few climate observatories at higher altitude (e.g. Mukhim and Tehri stations) with breaks in long-term climate data series. The observation of weather conditions near the Gaumukh is limited to the summer period in previous studies (Reference Singh, Haritashya, Ramasastri and KumarSingh and others, 2005, Reference Singh, Haritashya and Kumar2007). Our study shows a significant increasing trend in the annual T MAX, but a decreasing trend in the annual T MIN, during the study period. Reference Shekhar, Chand, Kumar, Srinivasan and GanjuShekhar and others (2010) also report an increase (of 2.8°C) in annual T MAX between 1984/85 and 2007/08 in the western Himalaya, while annual T MIN increased by about 1°C during a similar period in the western Himalaya. Reference Dash, Jenamani, Kalsi and PandaDash and others (2007) described decreasing trends (1.9°C) in T MIN over the western Himalaya during 1955–72, followed by an increasing trend in recent years. The present study notes a significant decreasing trend in T MIN in JJA at Mukhim station. The Tehri station data support these findings. Similarly, summer cooling has been reported in some parts of the western Himalaya and upper Indus basin during the latter part of the 20th century (Reference Yadav, Park, Singh and DubeyYadav and others, 2004; Reference Fowler and ArcherFowler and Archer, 2006). We report a significant increasing trend in T MAX during the winter at Mukhim station (Table 5). Reference Bhutiyani, Kale and PawarBhutiyani and others (2007) also found that winter mean T MAX for the northwestern Himalaya had increased significantly, by ∼3.2°C, during the past two decades.
The present study shows an insignificant decreasing trend in the monsoon precipitation, whereas no trend was found in the winter precipitation at Mukhim station. Reference Basistha, Arya and GoelBasistha and others (2009) also reported a precipitation decrease in the Uttarakhand Himalaya during 1965–80. Additionally, in the northwestern Himalaya, a significant decreasing trend has been reported in the monsoon precipitation during the period 1866–2006 (Reference Bhutiyani, Kale and PawarBhutiyani and others, 2009). We did not find any correlation between precipitation and temperature at Mukhim or Bhojbasa stations. This may be due to the short overlapping period of only 5 years of observations. It may also indicate that climate at the higher altitudes is influenced by local factors that suppress the general synoptic situation. This is the subject of further investigation.
Annual T MAX values show a significant increasing trend in the northwestern Himalaya as well as in the Garhwal Himalaya. However, to date, we are not able to determine the significance of effects of particular climate elements on glacier variability because: (1) availability of climate data in the study area near the glaciers (e.g. >4000 m a.s.l.) is insufficient; (2) analysis of T MAX and T MIN data for Mukhim station suggests diverse characteristics and results of T MIN data for Mukhim station and the western Himalaya do not show similar tendencies; (3) some climate parameters from Mukhim and Bhojbasa stations do not show similar tendencies; and (4) glacier changes in the Garhwal Himalaya show irregularities in amount, rate and time of occurrence during the study period which mainly depend on glacier response times. Sufficient knowledge on the response of Garhwal glaciers (fed by both winter and summer accumulation) to climate changes at different timescales is still not available. In addition, topographic parameters such as glacier size, shape, motion, thickness and distribution of debris-covered area, contribution of tributary glaciers to the accumulation and the local topography also influence glacier response. The summer-accumulation-type glaciers are more susceptible to temperature increase than winter-accumulation-type glaciers (Reference Fujita and AgetaFujita and Ageta, 2000; Reference FujitaFujita, 2008). Nevertheless current analysis of direct mass-balance measurements and meteorological measurements near Chorabari, Dokriani and Gangotri glaciers in the Garhwal Himalaya and geodetic estimates of glacier mass changes by DTM differencing will provide a valuable database in the near future, and will help to adjust existing glacier models to the specific situation in the Garhwal Himalaya.
Conclusion
This study provides a comprehensive multitemporal glacier fluctuation record for the upper Bhagirathi and Saraswati/Alaknanda basins of the Garhwal Himalaya from 1968 to 2006. Declassified Corona images are efficient for mapping glacier terrain in the absence of historical aerial images. Glacier area decreased from 599.9 ± 15.6 km2 (1968) to 572.5 ± 18.0 km2 (2006 ), i.e. 4.6 ± 2.8%. In contrast to the basin-wide ice loss, the absolute area loss in the Saraswati/ Alaknanda basin (18.4 ± 9.0 km2; 5.7 ± 2.7%) was almost two times higher than in the Bhagirathi basin (9.0 ± 7.7 km2; 3.3 ± 2.8%). This difference can be attributed mainly to the differences in mean glacier size. Smaller glaciers (<1 km2) lost 19.4 ± 2.5% (0.51 ± 0.07% a−1) of their ice, which is a higher rate than that of larger glaciers: for example, glaciers >50 km2 in area lost 2.8% ± 2.7% (0.074 ± 0.071% a−1) of their ice. This indicates that the average shrinkage rate is influenced by glacier size. Overall, south- and southwest-facing glaciers shrank at higher rates. Debris-covered glacier area increased by 17.8 ± 3.1% (0.46% a−1) from 1968 to 2006 in the Saraswati/Alaknanda basin and by 11.8 ± 3.0% (0.31 ± 0.08% a−1) in the upper Bhagirathi basin. Mean annual T MAX for Mukhim station increased significantly during 1957–2005, while precipitation during monsoon months decreased. However, the current availability of climate data in the study area is insufficient to conclude complex glacier–climate interactions, and thus further climatological investigations are needed.
Acknowledgements
We thank the Director of Wadia Institute of Himalayan Geology for support for this work, and the Director, Snow and Avalanche Study Establishment (SASE), Dr Snehmani, and the Additional Director General of Meteorology (Research), Pune, for providing climate data. R. Bhambri thanks P. Kawishwar (Resource Scientist, Department of Science and Technology, Chattisgarh) for guidance and support. We also thank: M. Sethi (Central Soil Salinity Research Institute, Karnal), S.K. Goyal (Principal, Guru Nanak Khalsa College (GNKC), Karnal) and P. Kaur (GNKC) for their support and provision of remote-sensing and GIS laboratory facilities; T.A. Scambos (Scientific Editor), J. Kargel and A. Racoviteanu for insightful comments and suggestions which significantly improved this paper; J. Fiddes and U. Kamp for proofreading; and L. Sharma (Librarian, Ratan Tata Library, Delhi University) and S.M.H. Rizvi (Librarian, GS, Lucknow) for providing valuable references. T. Bolch was funded partly by the European Space Agency project ‘GlobGlacier’. ASTER data were provided at no cost by NASA/US Geological Survey under the umbrella of the Global Land Ice Measurements from Space (GLIMS) project. DFG (Deutsche Forschungsgemeinschaft) provided financial support for the publication (Code BU 949/15-1).