Introduction
The field of astrobiology is broad and interdisciplinary encompassing not just life's evolution and its occurrence throughout the universe, but also the future of life on Earth and beyond. Life and the environment in which it lives are inextricably intertwined. Here we address the future of life on Earth with respect to its changing environment especially in light of global climate change. Astrobiology has as one of its core goals to understand the evolving relationship between Earth and its biota and how life responds to environmental change on a planetary scale. In this paper, we review how the space environment can help us lessen the negative impact of environmental change, especially global climate change, on terrestrial life.
Earth's changing environment
The human population is growing exponentially and is estimated to reach between 9.4 and 10.2 billion people by 2050 (Roser et al., Reference Roser, Ritchie and Ortiz-Ospina2020). With the rapid spread of industrialization and the human population's growth to unprecedented levels, environmental pollution has become a global problem. Human activities, such as the overuse of fossil fuels and land, unrestrained deforestation and intensive animal farming, to meet the growing population's demands have led to climate change (Masson-Delmotte et al., Reference Masson-Delmotte, Zhai, Pörtner, Roberts, Skea, Shukla, Pirani, Moufouma-Okia, Péan, Pidcock, Connors, Matthews, Chen, Zhou, Gomis, Lonnoy, Maycock, Tignor and Waterfield2018; Grossi et al., Reference Grossi, Goglio, Vitali and Williams2019). This is adversely affecting and transforming life on Earth (Edenhofer, Reference Edenhofer2015; Xu et al., Reference Xu, Li, Zheng, Si, Shi, Huang, Zhang, Cui and Cui2015; Zhang et al., Reference Zhang, Li and Zhu2018; Grossi et al., Reference Grossi, Goglio, Vitali and Williams2019). Changes in weather and climate are associated with the occurrence, attack and spread of opportunistic invasive species (Anderson et al., Reference Anderson, Cunningham, Patel, Morales, Epstein and Daszak2004; Backlund et al., Reference Backlund, Janetos and Schimel2008; Kernan, Reference Kernan2015; Bellard et al., Reference Bellard, Cassey and Blackburn2016; Gallardo et al., Reference Gallardo, Aldridge, González-Moreno, Pergl, Pizarro, Pyšek, Thuiller, Yesson and Vilà2017), thus increasing the stress on affected ecosystems. Climate warming is affecting plant growth and development, as well as causing the extinction of many species of plants and animals (Moritz and Agudo, Reference Moritz and Agudo2013). It is predicted that nearly 37% of species would become extinct by 2050 because of climate change (Thomas et al., Reference Thomas, Cameron, Green, Bakkenes, Beaumont, Collingham, Erasmus, Ferreira de Siqueira, Grainger, Hannah, Hughes, Huntley, van Jaarsveld, Midgley, Miles, Ortega-Huerta, Peterson, Phillips and Williams2004) and up to 50% by 2080 (Warren et al., Reference Warren, VanDerWal, Price, Welbergen, Atkinson, Ramirez-Villegas, Osborn, Jarvis, Shoo, Williams and Lowe2013). This clearly illustrates that global climate change impacts the future of life on Earth negatively and directly addresses goal 6 of the astrobiology roadmap to understand the principles that will shape the future of life, both on Earth and beyond. To address the Earth's problems listed above, several paths must be taken. One potential path is to use the space environment to quickly obtain mutant stains that could help alleviate these problems and simultaneously be advantageous for space exploration.
Food security is a serious issue facing humanity. It is predicted that food demand will increase by 60% by 2050 (Breene, Reference Breene2016). Intensive farming and poor soil management practices have destroyed the soil structure and composition in many areas, making it unsuitable for plant growth (Vitousek et al., Reference Vitousek, Naylor, Crews, David, Drinkwater, Holland, Johnes, Katzenberger, Martinelli, Matson, Nziguheba, Ojima, Palm, Robertson, Sanchez, Townsend and Zhang2009; Borrelli et al., Reference Borrelli, Robinson, Fleischer, Lugato, Ballabio, Alewell, Meusburger, Modugno, Schütt, Ferro, Bagarello, van Oost, Montanarella and Panagos2017). It is essential to increase the efficiency of crops and food systems to reduce pressure on soil and the environment. With climate change, extreme climate events and the emergence of new pathogens, agriculture's long-term sustainability is questionable. Agricultural losses due to attacks by pests, pathogens and invasive species are a major threat to agricultural production (Oerke and Dehne, Reference Oerke and Dehne2004; Vurro et al., Reference Vurro, Bonciani and Vannacci2010; Savary et al., Reference Savary, Willocquet, Pethybridge, Esker, McRoberts and Nelson2019). The use of agrochemicals (pesticides and fertilizers) has significantly increased crop production globally (Gomiero, Reference Gomiero2013; Popp et al., Reference Popp, Pető and Nagy2013), and has also caused severe adverse effects to beneficial soil organisms (e.g. bacteria, fungi, worms, etc.), animals, humans and plant growth and nutritional values (Udeigwe et al., Reference Udeigwe, Teboh, Eze, Stietiya, Kumar, Hendrix, Mascagni, Ying and Kandakji2015). Also, the extensive use of pesticides has led to the development of pesticide-resistant pests and pathogens (Gomiero, Reference Gomiero2013). Furthermore, modern agriculture practices have significantly reduced the diversity of crops making agriculture more vulnerable to pests, pathogens and environmental stresses (e.g. water scarcity, high and low temperatures, increased salinity, etc.) (Vurro et al., Reference Vurro, Bonciani and Vannacci2010; Dean et al., Reference Dean, van Kan, Pretorius, Hammond-Kosack, Di Pietro, Spanu, Rudd, Dickman, Kahmann, Ellis and Foster2012; Huang et al., Reference Huang, Raats, Sela, Klymiuk, Lidzbarsky, Feng, Krugman and Fahima2016; Savary et al., Reference Savary, Willocquet, Pethybridge, Esker, McRoberts and Nelson2019). For high and stable yield, crops require protection from biotic and abiotic stresses. Creating genetic diversity within crops can help restrain disease development, enhance yield, increase adaptability to abiotic factors and improve biodiversity (Schöb et al., Reference Schöb, Kerle, Karley, Morcillo, Pakeman, Newton and Brooker2015; Newton, Reference Newton2016; Savary et al., Reference Savary, Willocquet, Pethybridge, Esker, McRoberts and Nelson2019). For the sustainability of agriculture, it is important to consider the system's flexibility as a whole (holistic approach) and not adopt the reductionist strategy to improve tolerance by selecting traits (Newton, Reference Newton2016). For example, the focus should be on developing strains that are productive as well as disease tolerant and resistant to pests, pathogens and other hostile environmental conditions, such as drought, extreme temperatures, soil salinity, etc.
The most relevant results for developing sustainable, robust organisms and biomaterials for agricultural and health sectors will come from new biological research methods. The conventional strategies have been, to date, to search for well-adapted and well-performing organisms (Pickens et al., Reference Pickens, Tang and Chooi2011; King et al., Reference King, Edgar, Qiao and Stephanopoulos2016; Wohlleben et al., Reference Wohlleben, Mast, Stegmann and Ziemert2016; Zhang et al., Reference Zhang, Li and Zhu2018). We hypothesize that the simple and most redefining strategy would be to subject organisms to stress conditions that modulate evolutionary processes (recombination, mutation, epigenetic modification and gene transfer) and support the emergence of genetically diverse or new varieties of strains (Boto, Reference Boto2010; Lynch, Reference Lynch2010; Nei, Reference Nei2013; Lind and Spagopoulou, Reference Lind and Spagopoulou2018; Prasad et al., Reference Prasad, Richter, Vadakedath, Mancinelli, Krüger, Strauch, Grimm, Darriet, Chapel, Cohen and Lebert2020). Environmental stressors, including both biotic (e.g. parasites, pathogens, pests, etc.) and abiotic factors (e.g. chemicals, temperature, light, other climatic factors, etc.), are viewed as selection elements and play a significant role in shaping the acclimation, adaptation and evolution of species (Bijlsma and Loeschcke, Reference Bijlsma and Loeschcke1997; Hoffmann and Hercus, Reference Hoffmann and Hercus2000; Badyaev, Reference Badyaev2005; Parsons, Reference Parsons2005; Nevo, Reference Nevo2011). For example, the development of antibiotics, pesticide and heavy metal resistance in different organisms in response to the selection pressure exerted by these agents (Davies and Davies, Reference Davies and Davies2010; Lerminiaux and Cameron, Reference Lerminiaux and Cameron2019). The space environment consists of many unique stress factors that cause both mutagenic and/or non-mutagenic effects and can be exploited to induce evolutionary changes in cells and organisms (Horneck et al., Reference Horneck, Klaus and Mancinelli2010; Prasad et al., Reference Prasad, Richter, Vadakedath, Mancinelli, Krüger, Strauch, Grimm, Darriet, Chapel, Cohen and Lebert2020).
This review focuses on the distinctive environment of space and discusses the potential to explore it to develop space-engineered products that can find application in the agri-food and health-pharma sectors on Earth. But these same space environmental stressors can also be used to explore their potential to develop products and processes applicable to space exploration now and in the future. The development of biological processes producing more efficient pharmaceutical and health products crucial for astronauts’ health needs to be addressed. Drugs required aboard the International Space Station (ISS) are either brought along with the mission or resupplied regularly. While this may be possible on the ISS, it is impractical for a mission to Mars. The development of biological processes that produce drugs on demand is of tremendous value for realizing a human mission to Mars. We will not just go to Mars but eventually set up a permanent base on Mars, and to do that, we require to establish life-support systems that are robust with long lifetimes and needs few repairs – a technology that we currently do not have. However, we can build systems that are biological-based reactors, potentially using organisms engineered in the space environment to purify water by adapting forward osmotic systems (Flynn et al., Reference Flynn, Romero, Parodi, Mancenelli, Dougherty and Loftus2016) and air (Nelson and Bohn, Reference Nelson and Bohn2011). In fact, microbial processes that will enhance food productivity that has been developed in space are uniquely adapted to improve food productivity on Earth. Last but certainly not least, all of what we have learned and are learning about the space environment on organisms is directly applicable to planetary protection (Craven et al., Reference Craven, Winters, Smith, Lalime, Mancinelli, Shirey, Schubert, Schuerger, Burgin, Seto, Hendry, Mehta, Benardini and Ruvkun2021). The influence of space environment stress factors, mainly microgravity and radiation, that bring notable changes in cells or organismal systems are discussed in the review. Adopting the natural evolution process as a systemic, non-presumed approach for the production of sustained, robust organisms to overcome environmental obstacles is very tempting.
Space environmental factors inducing evolutionary changes
The hostile environment of space is characterized by factors that stress organisms in ways that cannot be mimicked on Earth, such as microgravity (weightlessness), intense ionizing radiation, high vacuum, pressure, space magnetic fields and extreme temperatures (Horneck et al., Reference Horneck, Klaus and Mancinelli2010; Kennedy, Reference Kennedy2014; Prasad et al., Reference Prasad, Richter, Vadakedath, Mancinelli, Krüger, Strauch, Grimm, Darriet, Chapel, Cohen and Lebert2020). These factors would not occur on Earth. Studies show that the space environment exerts distinct effects on genetics, mutation rate, gene expression, epigenetics, metabolite production and metabolic pathways and alters the shape, structure, function, physiology and growth behaviour of organisms. Thus, space can be explored as a ‘casting tray’ for the ‘guided evolution’ of species leading to the emergence of strains with novel properties (e.g. high yield, rapid growth, improved pathogen resistance, enhanced drought, disease tolerance, etc.) (Fig. 1) (Horneck et al., Reference Horneck, Klaus and Mancinelli2010; Milojevic and Weckwerth, Reference Milojevic and Weckwerth2020; Prasad et al., Reference Prasad, Richter, Vadakedath, Mancinelli, Krüger, Strauch, Grimm, Darriet, Chapel, Cohen and Lebert2020). The two major space environmental features that are widely studied include microgravity and ionizing radiation. These space stressors, which cannot be duplicated on Earth, can be used for the evolution of strains of organisms that will be more robust and more productive than their respective wild types on Earth.
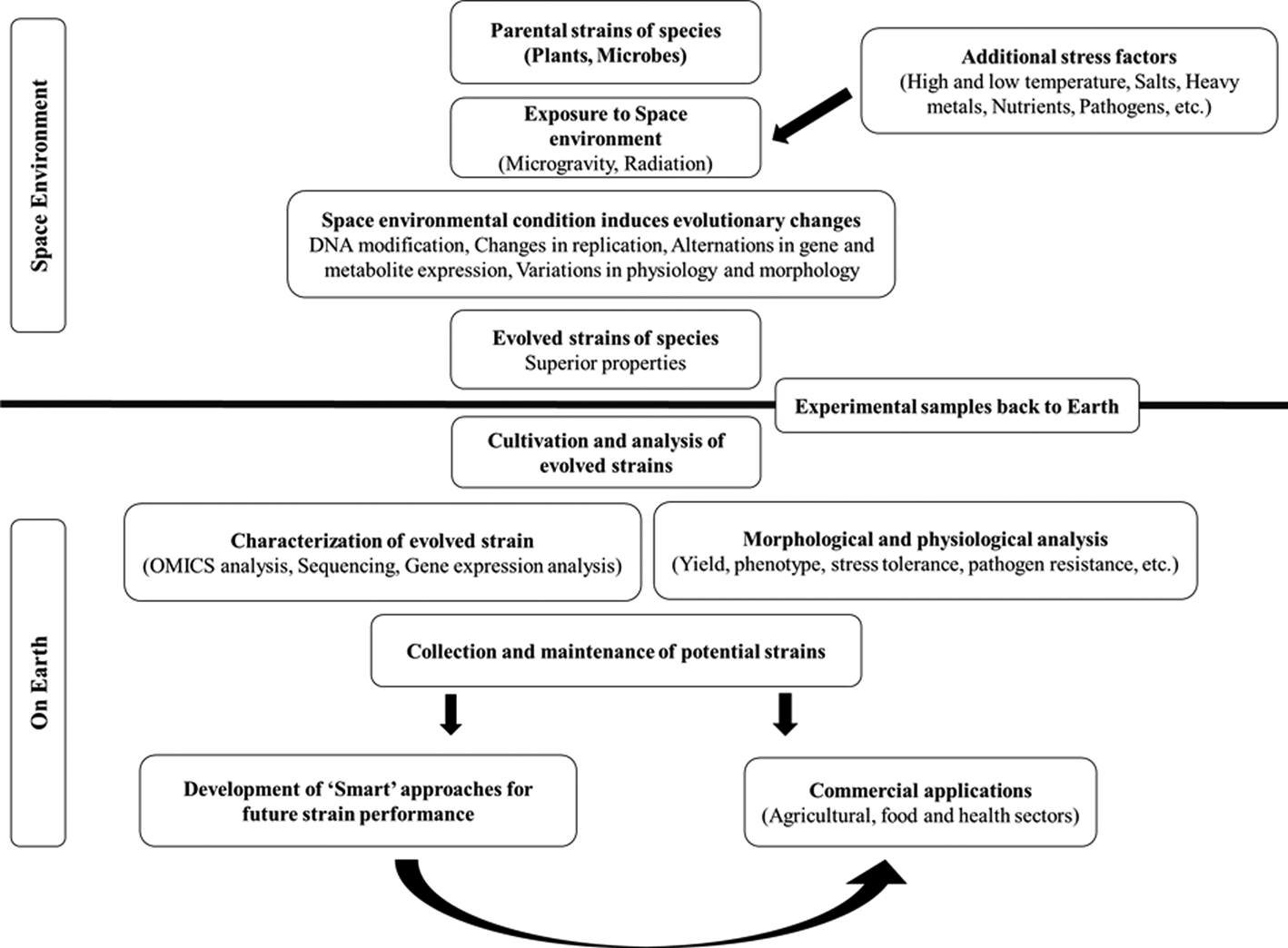
Fig. 1. Scheme of the utilization of space environmental conditions to support the emergence of species with novel characters. The unique space environment (microgravity and radiation) can be combined with abiotic and biotic stress factors to modulate novel evolutionary changes in species that cannot be achieved on Earth under the influence of gravity. These strains can find potential applications in food, agricultural and health sectors in a constantly changing and challenging environment. A systematic characterization of the naturally evolved strains on Earth will enlighten the underlying mechanisms of changes. In addition, ‘Smart’ strategies, including the engineering of gene regulatory networks by conventional breeding, mutagenesis and genetic engineering approaches and alteration of biological systems’ function by external or internal means such as environmental stimuli, can be implemented to enhance strain performance further. The knowledge can be applied to develop other novel strains as well.
Microgravity
Microgravity is, in essence, the absence of gravity, which is one of the four basic forces in physics that leads to the acceleration of the mass. The physical environment is entirely different in microgravity than under 1 × g (Todd, Reference Todd1989; Reference Todd1992; Horneck et al., Reference Horneck, Klaus and Mancinelli2010). Various physical phenomena are based on gravity's acceleration force, such as sedimentation, convection, hydrostatic pressure, etc. Under space microgravity conditions, sedimentation/stratification and convection are negligibly small since no acceleration force acts on solids, liquids and gases to separate them based on their density differences. This means that no buoyancy-driven convection occurs in liquids or gases under microgravity, whereas the constant exchange of volume occurs under 1 × g. Due to the lack of convection in microgravity, metabolites’ transport is reduced to the range (and rate) of diffusion. There is also no hydrostatic pressure in a water column in microgravity because hydrostatic pressure occurs due to acceleration forces. Also, shear forces are strongly reduced in microgravity because moving or rotating objects no longer work against acceleration vectors.
Microgravity can produce drastic effects on cell systems. For example, the fluidity of cell membranes is increased in microgravity (Sieber et al., Reference Sieber, Hanke and Kohn2014) that affects membrane proteins’ functionality, such as membrane channel conductance (Sieber et al., Reference Sieber, Kaltenbach, Hanke and Kohn2016; Kohn et al., Reference Kohn, Hauslage and Hanke2017). Due to the increase in membrane fluidity, drugs’ pharmacokinetic (incorporation of amphipathic drugs into membranes) are changed in microgravity (Kohn and Hauslage, Reference Kohn and Hauslage2019). It is also observed that the bacterial virulence, pathogenicity and resistance to antibiotics increase in space microgravity conditions (Wilson et al., Reference Wilson, Ott, Quick, Davis, zu Bentrup, Crabbé, Richter, Sarker, Barrila, Porwollik, Cheng, McClelland, Tsaprailis, Radabaugh, Hunt, Shah, Nelman-Gonzalez, Hing, Parra, Dumars, Norwood, Bober, Devich, Ruggles, CdeBaca, Narayan, Benjamin, Goulart, Rupert, Catella, Schurr, Buchanan, Morici, McCracken, Porter, Pierson, Smith, Mergeay, Leys, Stefanyshyn-Piper, Gorie, Nickerson and Ausubel2008). Thus, space's unique microgravity environment can be used to understand microbes’ physiology and pathogenesis and develop novel treatment strategies (Amselem, Reference Amselem2019).
Gravity cannot be eliminated on Earth to perform experiments without its influence. There are several methods of achieving simulated microgravity conditions for scientific experiments that differ in duration, frequency and level of gravity, as well as on the type of research (Sabbatini, Reference Sabbatini2014; Amselem, Reference Amselem2019; Prasad et al., Reference Prasad, Richter, Vadakedath, Mancinelli, Krüger, Strauch, Grimm, Darriet, Chapel, Cohen and Lebert2020). Several devices providing simulated microgravity conditions have been developed with an advantage in cost, easy accessibility and reproducibility. Examples include 2D- or 3D-clinostat, random positioning machines, rotating wall vessels, rotating-wall bioreactor, rotary cell culture system, etc. (Horneck et al., Reference Horneck, Klaus and Mancinelli2010; Prasad et al., Reference Prasad, Richter, Vadakedath, Mancinelli, Krüger, Strauch, Grimm, Darriet, Chapel, Cohen and Lebert2020). However, none of these methods provide real microgravity conditions similar to space since gravity cannot be shielded or reduced. Real microgravity can only be achieved under free-fall conditions. Platforms, such as drop towers, sounding rockets, aircraft flying parabolic manoeuvres, spacecraft (research satellites) and the ISS are utilized for microgravity research (Pletser, Reference Pletser2004; Ruyters and Friedrich, Reference Ruyters and Friedrich2006; Baglioni et al., Reference Baglioni, Sabbatini, Horneck, Horneck and Rettberg2007; Horneck et al., Reference Horneck, Klaus and Mancinelli2010; Böhmer and Schleiff, Reference Böhmer and Schleiff2019). The duration of sample preparation, exposure and recovery significantly differs among the various platforms used to achieve real microgravity conditions. Depending upon the type of platform used, microgravity values between 10−3 and 10−6g can be achieved (Horneck et al., Reference Horneck, Klaus and Mancinelli2010; Böhmer and Schleiff, Reference Böhmer and Schleiff2019). Also, depending upon the method, the degree of automatization varies. During spaceflights, test samples encounter a complex environment consisting of acceleration, microgravity, vibration, radiation and electromagnetism, among other environmental stresses, which are impossible to simulate completely on the ground (Horneck et al., Reference Horneck, Klaus and Mancinelli2010; Taylor, Reference Taylor2015).
Space ionizing radiation
Among space stress factors, ionizing radiation is probably the primary constraint for organisms’ growth and human-crewed space missions (Kennedy, Reference Kennedy2014; Furukawa et al., Reference Furukawa, Nagamatsu, Nenoi, Fujimori, Kakinuma, Katsube, Wang, Tsuruoka, Shirai, Nakamura, Sakaue-Sawano, Miyawaki, Harada, Kobayashi, Kobayashi, Kunieda, Funayama, Suzuki, Miyamoto, Hidema, Yoshida and Takahashi2020). Space radiation is composed of galactic cosmic radiation, solar cosmic radiation and radiation trapped by Earth's magnetosphere (van Allen belts) (Ohnishi and Ohnishi, Reference Ohnishi and Ohnishi2004). Galactic cosmic radiation is produced by the Sun and supernova remnants. It consists of 87% high-energy protons, 12% α-particles and 1% heavier nuclei (Horneck et al., Reference Horneck, Klaus and Mancinelli2010; Kennedy, Reference Kennedy2014; Li et al., Reference Li, Jella, Jaafar, Li, Park, Story, Wang, Wang and Dynan2018). The heavier nuclei comprise high-charge and high-energy particles called HZE particles (High charge Z and high Energy). The solar cosmic radiation consists of low solar wind particles to highly energetic charged particles (up to several GeV) originating from solar energy (Horneck et al., Reference Horneck, Klaus and Mancinelli2010; Kennedy, Reference Kennedy2014; Li et al., Reference Li, Jella, Jaafar, Li, Park, Story, Wang, Wang and Dynan2018). The solar energy particle events arise from the sun's magnetically disturbed regions and generally last for a few hours. About 92% protons, 6% helium ions and 6% HZE ions make up these highly energetic particles. The Earth's magnetic field provides latitude-dependent protection against solar particle events. The galactic and solar cosmic radiation trapped within the Earth's magnetic field and atmosphere form the van Allen belt, consisting of electrons, protons and some heavier particles (Horneck et al., Reference Horneck, Klaus and Mancinelli2010). The inner belt particles emerge from the decay of neutrons produced in cosmic particle interactions with the atmosphere, while the outer belt mainly consists of trapped solar particles. The average dose of radiation aboard the ISS is about 180 μGy day−1 (Goossens et al., Reference Goossens, Vanhavere, Leys, de Boever, O'Sullivan, Zhou, Spurny, Yukihara, Gaza and McKeever2006; Vanhavere et al., Reference Vanhavere, Genicot, O'Sullivan, Zhou, Spurný, Jadrníčková, Sawakuchi and Yukihara2008), which is up to 80-fold higher than the natural radiation levels on Earth.
The impact of space radiation on organisms is an essential aspect of study for space missions. The biological effects of radiation depend upon the quality and quantity of radiation, exposure duration, the quality of the inflicted damage and characteristics and developmental stage of the cell/tissue/organism (Horneck et al., Reference Horneck, Klaus and Mancinelli2010; de Micco et al., Reference de Micco, Arena, Pignalosa and Durante2011; Matallana-Surget and Wattiez, Reference Matallana-Surget and Wattiez2013). The significant effect of space ionizing radiation (γ-ray, X-ray, β-ray, α-radiation and neutron radiation) is DNA damage (single- or double-strand breaks), which activates cellular responses leading to cell cycle arrest and appropriate DNA repair mechanisms (base-excision repair, homologous recombination or non-homologous end-joining repair) depending on the nature of the damage (Zhou and Elledge, Reference Zhou and Elledge2000). The HZE particles have high linear energy transfer (LET) values (> 10 keV μm−1) and are considered as the main component of radiation-induced cellular damages (complex, difficult-to repair DNA damages) and health risks to astronauts (Cucinotta et al., Reference Cucinotta, Manuel, Jones, Iszard, Murrey, Djojonegro and Wear2001; Nikjoo et al., Reference Nikjoo, O'Neill, Wilson and Goodhead2001; Cucinotta and Durante, Reference Cucinotta and Durante2006; Friedland et al., Reference Friedland, Dingfelder, Kundrát and Jacob2011; Arena et al., Reference Arena, de Micco, Macaeva and Quintens2014). The LET values of electromagnetic ionizing radiations (X-rays and γ-rays) are relatively lower than high LET radiations (α particles, neutrons and protons) but have a high penetration power into matter (Reisz et al., Reference Reisz, Bansal, Qian, Zhao and Furdui2014). The biological damages produced by the X-rays and γ-rays are lower than those induced by the same dose of high-LET radiations. The significant cellular effects produced by X- and γ-rays is due to the generation of reactive oxygen species (ROS) (H2O2, O2•− and •OH) and other reductants (H• and eaq−) (Reisz et al., Reference Reisz, Bansal, Qian, Zhao and Furdui2014; Desiderio et al., Reference Desiderio, Salzano, Scaloni, Massa, Pimpinella, de Coste, Pioli, Nardi, Benvenuto and Villani2019). These highly reactive agents cause DNA breaks, protein fragmentation, protein structure deterioration and cell membrane function and permeability changes.
Several experiments have been performed in space aboard space stations and different satellites as well as on Earth to study the effects of cosmic radiation on cells/tissue/organisms from the perspective of manned space missions (Horneck et al., Reference Horneck, Klaus and Mancinelli2010; Arena et al., Reference Arena, de Micco, Macaeva and Quintens2014; Furukawa et al., Reference Furukawa, Nagamatsu, Nenoi, Fujimori, Kakinuma, Katsube, Wang, Tsuruoka, Shirai, Nakamura, Sakaue-Sawano, Miyawaki, Harada, Kobayashi, Kobayashi, Kunieda, Funayama, Suzuki, Miyamoto, Hidema, Yoshida and Takahashi2020). Different facilities simulating space-like radiation are available to evaluate ionizing radiation's biological effects (Furukawa et al., Reference Furukawa, Nagamatsu, Nenoi, Fujimori, Kakinuma, Katsube, Wang, Tsuruoka, Shirai, Nakamura, Sakaue-Sawano, Miyawaki, Harada, Kobayashi, Kobayashi, Kunieda, Funayama, Suzuki, Miyamoto, Hidema, Yoshida and Takahashi2020). However, space ionizing radiation cannot be simulated to its full complexity on Earth (Moissl-Eichinger et al., Reference Moissl-Eichinger, Cockell and Rettberg2016). In space, radiation may act independently or synergistically with other stress factors, such as microgravity, space vacuum and low shear forces, among others, to produce significant effects on cells/tissue/organisms.
Experiments in space and ground-based space simulation facilities
The first space experiments, mostly done by Russian scientists in the 1960s, revealed that microgravity does not produce any considerable effects on living organisms (Zhukov-Verezhnikov et al., Reference Zhukov-Verezhnikov, Maiskii, Yazdovskii, Pekhov, Gyurdzhian, Nefed'eva, Kapichnikov, Podoplelov, Rybakov, Klemparskaya and Klimov1962; Montgomery et al., Reference Montgomery, Cook, Reynolds, Paul, Hayflick, Stock, Schulz, Kimsey, Thirolf, Rogers and Campbell1978; Tairbekov et al., Reference Tairbekov, Parfyonov, Shepelev and Sushkov1983). However, further experiments in the space environment showed that space-specific conditions change the biological properties of cells. Changes in genetics, gene expression, metabolite production and function, and morphology, growth and behaviour of cells/organisms have been observed in response to the space environment and space analogue conditions. It is proposed that microgravity primarily alters cell growth kinetics and behaviour, while radiation contributes to increased mutation rates (Taylor, Reference Taylor2015; Senatore et al., Reference Senatore, Mastroleo, Leys and Mauriello2018).
Effect of microgravity on microorganisms
Many microorganisms can survive and thrive in extreme environments, including space hostile environment (Bronikowski et al., Reference Bronikowski, Bennett and Lenski2001; Benoit et al., Reference Benoit, Li, Stodieck, Lam, Winther, Roane and Klaus2006; Horneck et al., Reference Horneck, Klaus and Mancinelli2010; Vaishampayan et al., Reference Vaishampayan, Rabbow, Horneck and Venkateswaran2012; Aunins et al., Reference Aunins, Erickson, Prasad, Levy, Jones, Shrestha, Mastracchio, Stodieck, Klaus, Zea and Chatterjee2018; Huang et al., Reference Huang, Li, Huang and Liu2018). A diverse range of microorganisms has been sent into space to study the effects of the space environment (Taylor, Reference Taylor1974; Juergensmeyer et al., Reference Juergensmeyer, Juergensmeyer and Guikema1999; Kacena et al., Reference Kacena, Manfredi and Todd1999a; Nickerson et al., Reference Nickerson, Ott, Wilson, Ramamurthy and Pierson2004; Clément and Slenzka, Reference Clément and Slenzka2006; Wilson et al., Reference Wilson, Ott, zu Bentrup, Ramamurthy, Quick, Porwollik, Cheng, McClelland, Tsaprailis, Radabaugh, Hunt, Fernandez, Richter, Shah, Kilcoyne, Joshi, Nelman-Gonzalez, Hing, Parra, Dumars, Norwood, Bober, Devich, Ruggles, Goulart, Rupert, Stodieck, Stafford, Catella, Schurr, Buchanan, Morici, McCracken, Allen, Baker-Coleman, Hammond, Vogel, Nelson, Pierson, Stefanyshyn-Piper and Nickerson2007; Reference Wilson, Ott, Quick, Davis, zu Bentrup, Crabbé, Richter, Sarker, Barrila, Porwollik, Cheng, McClelland, Tsaprailis, Radabaugh, Hunt, Shah, Nelman-Gonzalez, Hing, Parra, Dumars, Norwood, Bober, Devich, Ruggles, CdeBaca, Narayan, Benjamin, Goulart, Rupert, Catella, Schurr, Buchanan, Morici, McCracken, Porter, Pierson, Smith, Mergeay, Leys, Stefanyshyn-Piper, Gorie, Nickerson and Ausubel2008; Horneck et al., Reference Horneck, Klaus and Mancinelli2010; Crabbé et al., Reference Crabbé, Schurr, Monsieurs, Morici, Schurr, Wilson, Ott, Tsaprailis, Pierson, Stefanyshyn-Piper and Nickerson2011; Reference Crabbé, Nielsen-Preiss, Woolley, Barrila, Buchanan, McCracken, Inglis, Searles, Nelman-Gonzalez, Ott, Wilson, Pierson, Stefanyshyn-Piper, Hyman, Nickerson and Coste2013; Rosenzweig and Chopra, Reference Rosenzweig and Chopra2012; Kim et al., Reference Kim, Tengra, Shong, Marchand, Chan, Young, Pangule, Parra, Dordick, Plawsky and Collins2013a; Reference Kim, Tengra, Young, Shong, Marchand, Chan, Pangule, Parra, Dordick, Plawsky and Collins2013b; Huang et al., Reference Huang, Liu, Rong, Ruan and Huang2015). Also, several experiments have been performed in simulated microgravity conditions on Earth to study the response of different microorganisms (Nickerson et al., Reference Nickerson, Ott, Mister, Morrow, Burns-Keliher and Pierson2000; Baker et al., Reference Baker, Meyer and Leff2004; Chopra et al., Reference Chopra, Fadl, Sha, Chopra, Galindo and Chopra2006; Tucker et al., Reference Tucker, Ott, Huff, Fofanov, Pierson, Willson and Fox2007; Altenburg et al., Reference Altenburg, Nielsen-Preiss and Hyman2008; Gao et al., Reference Gao, Liu and Zhang2011; Searles et al., Reference Searles, Woolley, Petersen, Hyman and Nielsen-Preiss2011; Pacello et al., Reference Pacello, Rotilio and Battistoni2012; Arunasri et al., Reference Arunasri, Adil, Venu Charan, Suvro, Himabindu Reddy and Shivaji2013; Lawal et al., Reference Lawal, Kirtley, van Lier, Erova, Kozlova, Sha, Chopra and Rosenzweig2013; Rosenzweig et al., Reference Rosenzweig, Ahmed, Eunson and Chopra2014; Soni et al., Reference Soni, O'Sullivan, Quick, Ott, Nickerson and Wilson2014; Huang et al., Reference Huang, Liu, Rong, Ruan and Huang2015; Xu et al., Reference Xu, Li, Zheng, Si, Shi, Huang, Zhang, Cui and Cui2015; Kim et al., Reference Kim, Lee, Choi, Bahinski and Ingber2016). Both real and simulated microgravity conditions have been shown to influence microbial growth, physiology, virulence, pathogenesis, antibiotic resistance, stress tolerance and secondary metabolite production (Lapchine et al., Reference Lapchine, Moatti, Gasset, Richoilley, Templier and Tixador1986; Nickerson et al., Reference Nickerson, Ott, Mister, Morrow, Burns-Keliher and Pierson2000; Ilyin, Reference Ilyin2005; Horneck et al., Reference Horneck, Klaus and Mancinelli2010; Mermel, Reference Mermel2012; Kalpana et al., Reference Kalpana, Im and Lee2016; Senatore et al., Reference Senatore, Mastroleo, Leys and Mauriello2018; Tirumalai et al., Reference Tirumalai, Karouia, Tran, Stepanov, Bruce, Ott, Pierson and Fox2019).
A significant number of studies have investigated the growth response of different microorganisms, including bacteria, fungi, archaea and microalgae, to real and simulated microgravity conditions. Studies with Escherichia coli (Bouloc and D'Ari, Reference Bouloc and D'Ari1991; Thévenet et al., Reference Thévenet, D'ari and Bouloc1996; Kacena et al., Reference Kacena, Leonard, Todd and Luttges1997; Reference Kacena, Merrell, Manfredi, Smith, Klaus and Todd1999b; Brown et al., Reference Brown, Klaus and Todd2002; Benoit and Klaus, Reference Benoit and Klaus2005; Tucker et al., Reference Tucker, Ott, Huff, Fofanov, Pierson, Willson and Fox2007; Arunasri et al., Reference Arunasri, Adil, Venu Charan, Suvro, Himabindu Reddy and Shivaji2013; Tirumalai et al., Reference Tirumalai, Karouia, Tran, Stepanov, Bruce, Ott, Pierson and Fox2017), Bacillus subtilis (Kacena et al., Reference Kacena, Leonard, Todd and Luttges1997; Reference Kacena, Merrell, Manfredi, Smith, Klaus and Todd1999b), Salmonella typhimurium (Wilson et al., Reference Wilson, Ott, Ramamurthy, Porwollik, McClelland, Pierson and Nickerson2002a), Pseudomonas aeruginosa (England et al., Reference England, Gorzelak and Trevors2003; Kim et al., Reference Kim, Tengra, Shong, Marchand, Chan, Young, Pangule, Parra, Dordick, Plawsky and Collins2013a; Reference Kim, Tengra, Young, Shong, Marchand, Chan, Pangule, Parra, Dordick, Plawsky and Collins2013b), Staphylococcus (Rosado et al., Reference Rosado, Doyle, Hinds and Taylor2010), Streptococcus (Allen et al., Reference Allen, Galindo, Pandya, Watson, Chopra and Niesel2007; Orsini et al., Reference Orsini, Lewis and Rice2017), Streptomyces (Fang et al., Reference Fang, Pierson, Mishra, Koenig and Demain1997c; Reference Fang, Pierson, Mishra and Demain2000), Saccharomyces cerevisiae (Purevdorj-Gage et al., Reference Purevdorj-Gage, Sheehan and Hyman2006; van Mulders et al., Reference van Mulders, Stassen, Daenen, Devreese, Siewers, van Eijsden, Nielsen, Delvaux and Willaert2011), Candida albicans (Altenburg et al., Reference Altenburg, Nielsen-Preiss and Hyman2008; Crabbé et al., Reference Crabbé, Nielsen-Preiss, Woolley, Barrila, Buchanan, McCracken, Inglis, Searles, Nelman-Gonzalez, Ott, Wilson, Pierson, Stefanyshyn-Piper, Hyman, Nickerson and Coste2013; Jiang et al., Reference Jiang, Xu, Yi, Huang, Li, Jiang, Zhou, Zhang and Cui2014), Deinococcus radiodurans (Ott et al., Reference Ott, Fuchs, Moeller, Hemmersbach, Kawaguchi, Yamagishi, Weckwerth and Milojevic2019), Haloarchaea (Dornmayr-Pfaffenhuemer et al., Reference Dornmayr-Pfaffenhuemer, Legat, Schwimbersky, Fendrihan and Stan-Lotter2011; Thombre et al., Reference Thombre, Shinde, Dixit, Jagtap and Vidyasagar2017), Euglena (Häder et al., Reference Häder, Richter, Ntefidou and Lebert2005; Nasir et al., Reference Nasir, Strauch, Becker, Sperling, Schuster, Richter, Weißkopf, Ntefidou, Daiker, An, Li, Liu, Lebert and Legué2014; Krüger et al., Reference Krüger, Richter, Stoltze, Strauch, Krüger, Daiker, Prasad, Sonnewald, Reid and Lebert2019), Chlamydomonas (Yoshimura et al., Reference Yoshimura, Matsuo and Kamiya2003; Häder et al., Reference Häder, Richter, Ntefidou and Lebert2005), etc., have been conducted. Most studies have reported reduced lag phase and increased cell population across several bacterial species in the space environment (Benoit and Klaus, Reference Benoit and Klaus2007; Horneck et al., Reference Horneck, Klaus and Mancinelli2010; Huang et al., Reference Huang, Li, Huang and Liu2018). The differences in growth rate are attributed to the type of cell and species used, the property of cell motility, culture conditions (suspension and solid-state agar culture), concentrations of medium nutrient (high and low), the extracellular microenvironment, the type of bioreactors used inside the spacecraft and microgravity conditions (Klaus et al., Reference Klaus, Benoit, Nelson and Hammond2004; Horneck et al., Reference Horneck, Klaus and Mancinelli2010; Taylor, Reference Taylor2015; Huang et al., Reference Huang, Li, Huang and Liu2018). Also, it is proposed that the indirect physical effects of microgravity (viz. fluid dynamics, extracellular transport) have a significant influence on bacterial cell growth rather than the direct impact during spaceflight (Horneck et al., Reference Horneck, Klaus and Mancinelli2010; Huang et al., Reference Huang, Li, Huang and Liu2018). In microgravity, decreased mass diffusion, low shear forces and changes in fluid dynamics alter a cell's microenvironment influencing growth and physiology. The direct effect of microgravity on cell growth is mainly observed in higher organisms that possess unique molecules or organelles to sense gravity (Limbach et al., Reference Limbach, Hauslage, Schäfer and Braun2005; Horneck et al., Reference Horneck, Klaus and Mancinelli2010; Huang et al., Reference Huang, Li, Huang and Liu2018; Nasir et al., Reference Nasir, Le Bail, Daiker, Klima, Richter and Lebert2018; Prasad et al., Reference Prasad, Richter, Vadakedath, Mancinelli, Krüger, Strauch, Grimm, Darriet, Chapel, Cohen and Lebert2020).
A significant knowledge gap remains regarding the specific mechanisms involved in altering virulence, pathogenesis and resistance to antibiotics of pathogens in the microgravity environment. Based on studies conducted to date, it is speculated that global regulatory signals may control these change (Wilson et al., Reference Wilson, Ramamurthy, Porwollik, McClelland, Hammond, Allen, Ott, Pierson and Nickerson2002b, Reference Wilson, Ott, zu Bentrup, Ramamurthy, Quick, Porwollik, Cheng, McClelland, Tsaprailis, Radabaugh, Hunt, Fernandez, Richter, Shah, Kilcoyne, Joshi, Nelman-Gonzalez, Hing, Parra, Dumars, Norwood, Bober, Devich, Ruggles, Goulart, Rupert, Stodieck, Stafford, Catella, Schurr, Buchanan, Morici, McCracken, Allen, Baker-Coleman, Hammond, Vogel, Nelson, Pierson, Stefanyshyn-Piper and Nickerson2007; Nickerson et al., Reference Nickerson, Ott, Wilson, Ramamurthy, LeBlanc, Höner zu Bentrup, Hammond and Pierson2003; Crabbé et al., Reference Crabbé, Schurr, Monsieurs, Morici, Schurr, Wilson, Ott, Tsaprailis, Pierson, Stefanyshyn-Piper and Nickerson2011; Aunins et al., Reference Aunins, Erickson, Prasad, Levy, Jones, Shrestha, Mastracchio, Stodieck, Klaus, Zea and Chatterjee2018; Ott et al., Reference Ott, Crabbé, Wilson, Barrila, Castro-Wallace, Nickerson and Choukèr2020). Studies have shown the involvement of the global transcriptional regulator Hfq, a RNA-binding protein, in the induction of virulence-related genes in pathogenic bacteria (e.g. P. aeruginosa, S. typhimurium) in spaceflight or spaceflight analogue conditions (Wilson et al., Reference Wilson, Ott, zu Bentrup, Ramamurthy, Quick, Porwollik, Cheng, McClelland, Tsaprailis, Radabaugh, Hunt, Fernandez, Richter, Shah, Kilcoyne, Joshi, Nelman-Gonzalez, Hing, Parra, Dumars, Norwood, Bober, Devich, Ruggles, Goulart, Rupert, Stodieck, Stafford, Catella, Schurr, Buchanan, Morici, McCracken, Allen, Baker-Coleman, Hammond, Vogel, Nelson, Pierson, Stefanyshyn-Piper and Nickerson2007; Crabbé et al., Reference Crabbé, Schurr, Monsieurs, Morici, Schurr, Wilson, Ott, Tsaprailis, Pierson, Stefanyshyn-Piper and Nickerson2011; Ott et al., Reference Ott, Crabbé, Wilson, Barrila, Castro-Wallace, Nickerson and Choukèr2020). In a low-shear modelled microgravity (LSMMG) environment experiment, Wilson et al. (Reference Wilson, Ramamurthy, Porwollik, McClelland, Hammond, Allen, Ott, Pierson and Nickerson2002b) observed the differential expression of 163 genes representing functionally diverse groups (transcriptional regulators, virulence factors, lipopolysaccharide biosynthetic enzymes, iron-utilization enzymes and proteins of unknown function) and discovered the role of ferric uptake regulator (Fur) in the regulation of virulence, stress resistance and protein expression in Salmonella enterica in microgravity. Nickerson et al. (Reference Nickerson, Ott, Mister, Morrow, Burns-Keliher and Pierson2000) found S. enterica serovar Typhimurium cells grown in simulated microgravity more virulent and resistant to acid stress and macrophage killing than normal-gravity-grown cells. Higher cell counts in murine spleen and liver and significant differences in protein synthesis were also found in simulated microgravity (Nickerson et al., Reference Nickerson, Ott, Mister, Morrow, Burns-Keliher and Pierson2000). They indicated that the microgravity environment regulates the expression of genes and proteins, contributing to these effects in S. enterica. Alteration of gene and protein expression was also found in bacteria that showed higher virulence and better adherence to human cells under simulated microgravity conditions than in normal 1 × g (Poudrier, Reference Poudrier2003). Morphological alterations can contribute to microbial pathogenicity. Induction of cell aggregation, clumping and extracellular matrix formation have been observed in virulent strains of S. typhimurium cultivated in spaceflight (Wilson et al., Reference Wilson, Ott, zu Bentrup, Ramamurthy, Quick, Porwollik, Cheng, McClelland, Tsaprailis, Radabaugh, Hunt, Fernandez, Richter, Shah, Kilcoyne, Joshi, Nelman-Gonzalez, Hing, Parra, Dumars, Norwood, Bober, Devich, Ruggles, Goulart, Rupert, Stodieck, Stafford, Catella, Schurr, Buchanan, Morici, McCracken, Allen, Baker-Coleman, Hammond, Vogel, Nelson, Pierson, Stefanyshyn-Piper and Nickerson2007). Also, the increased frequency of filamentous forms contributing to virulence has been noted in C. albicans under microgravity conditions (Altenburg et al., Reference Altenburg, Nielsen-Preiss and Hyman2008).
Very recently, Gilbert et al. (Reference Gilbert, Torres, Clemens, Hateley, Hosamani, Wade and Bhattacharya2020) found that Serratia marcescens was more lethal to Drosophila melanogaster on the ISS than in ground-based controls and suggested that spaceflight induces a physiological change leading to increased virulence in the pathogen. In contrast, the virulence of clinical pathogens (Listeria monocytogenes, methicillin-resistant S. aureus (MRSA), Enterococcus faecalis and C. albicans) against larvae and adult Caenorhabditis elegans reduced in the space environment (Hammond et al., Reference Hammond, Stodieck, Birdsall, Becker, Koenig, Hammond, Gunter and Allen2013). No effect on the virulence of MRSA and Listeria against both adult and larvae was noted in simulated microgravity conditions (clinorotation), while Candida and Enterococcus were less virulent for larvae, but not adult worms of C. elegans. These data suggest that real and simulated microgravity environments alter the interactions between host and virulent bacteria.
An increase in resistance to antibiotics and various stresses, such as acid, thermal and osmotic, is also observed in a real and simulated space environment (Leys et al., Reference Leys, Hendrickx, de Boever, Baatout and Mergeay2004; Klaus and Howard, Reference Klaus and Howard2006; Horneck et al., Reference Horneck, Klaus and Mancinelli2010; Taylor, Reference Taylor2015; Thombre et al., Reference Thombre, Shinde, Dixit, Jagtap and Vidyasagar2017; Aunins et al., Reference Aunins, Erickson, Prasad, Levy, Jones, Shrestha, Mastracchio, Stodieck, Klaus, Zea and Chatterjee2018). Higher concentrations of antibiotics are required to inhibit bacterial growth in microgravity. This is probably due to the lower transport rate (mass transport is limited to diffusion) and changes in cell proliferation, membrane permeability, rate and morphology (Klaus et al., Reference Klaus, Simske, Todd and Stodieck1997, Reference Klaus, Benoit, Nelson and Hammond2004; Kacena and Todd, Reference Kacena and Todd1999; Sieber et al., Reference Sieber, Hanke and Kohn2014; Zea et al., Reference Zea, Prasad, Levy, Stodieck, Jones, Shrestha and Klaus2016, Reference Zea, Larsen, Estante, Qvortrup, Moeller, Dias de Oliveira, Stodieck and Klaus2017; Kohn et al., Reference Kohn, Hauslage and Hanke2017; Aunins et al., Reference Aunins, Erickson, Prasad, Levy, Jones, Shrestha, Mastracchio, Stodieck, Klaus, Zea and Chatterjee2018; Kohn and Hauslage, Reference Kohn and Hauslage2019). In a study with the halophilic archaeon, Haloarcula argentinensis under simulated microgravity condition (clinostat), an increase in the activity of antibiotic efflux pumps resulting in the development of multi-drug resistance to antibiotics, such as augmentin, norfloxacin, tobramycin and cefoperazone was observed (Thombre et al., Reference Thombre, Shinde, Dixit, Jagtap and Vidyasagar2017). Studies have also indicated the involvement of global regulators (hfq and rpoS) in cross-resistance to antibiotics in Salmonella and E. coli under microgravity conditions (Wilson et al., Reference Wilson, Ott, Ramamurthy, Porwollik, McClelland, Pierson and Nickerson2002a; Lynch et al., Reference Lynch, Brodie and Matin2004; Horneck et al., Reference Horneck, Klaus and Mancinelli2010).
Increases in antibiotic resistance have also been observed in some microorganisms and have been associated with mutations of genes in the space environment (Fajardo-Cavazos and Nicholson, Reference Fajardo-Cavazos and Nicholson2016; Fajardo-Cavazos et al., Reference Fajardo-Cavazos, Leehan and Nicholson2018; Tirumalai et al., Reference Tirumalai, Karouia, Tran, Stepanov, Bruce, Ott, Pierson and Fox2019). Dramatic differences in the spectrum of mutations in the regulator gene rpoB leading to rifampicin-resistance (RifR) have been observed in B. subtilis and Staphylococcus epidermidis cultivated in the ISS compared to ground controls, indicating the mutagenic potential of spaceflight environment (Fajardo-Cavazos and Nicholson, Reference Fajardo-Cavazos and Nicholson2016; Fajardo-Cavazos et al., Reference Fajardo-Cavazos, Leehan and Nicholson2018). The frequency of mutation to RifR in S. epidermidis cells flown aboard the ISS was observed to be significantly higher (24-fold) than ground controls (Fajardo-Cavazos and Nicholson, Reference Fajardo-Cavazos and Nicholson2016). In a recent study, Tirumalai et al. (Reference Tirumalai, Karouia, Tran, Stepanov, Bruce, Ott, Pierson and Fox2019) noted mutations in multiple genes associated with antibiotic resistance (ompF, acrB, marR and mdfA) in E. coli cells grown under LSMMG for over 1000 generation leading to resistance against chloramphenicol, cefalotin, cefuroxime, cefuroxime axetil, cefoxitin and tetracycline. The resistance was stable in successive generations grown under normal gravity conditions (Tirumalai et al., Reference Tirumalai, Karouia, Tran, Stepanov, Bruce, Ott, Pierson and Fox2019). Authors have linked the spread and increase of virulence and antibiotic resistance in spaceflight conditions to gene transfer events as well (Timmery et al., Reference Timmery, Hu and Mahillon2011; Senatore et al., Reference Senatore, Mastroleo, Leys and Mauriello2018; Tirumalai et al., Reference Tirumalai, Karouia, Tran, Stepanov, Bruce, Ott, Pierson and Fox2019). Efficient gene transfer events have been noticed in the Gram-positive bacteria Bacillus sp. in spaceflight compared to ground controls, whereas no differences have been observed in the Gram-negative species E. coli and Cupriavidus metallidurans (de Boever et al., Reference de Boever, Mergeay, Ilyin, Forget-Hanus, Van der Auwera and Mahillon2007). Changes in conjugal transfer rates and phage induction have also been noted under space microgravity conditions (Mattoni, Reference Mattoni1968; Ciferri et al., Reference Ciferri, Tiboni, Di Pasquale, Orlandoni and Marchesi1986; Alpatov et al., Reference Alpatov, I'lin, Antipov and Tairbekov1989). Recently, Aunins et al. (Reference Aunins, Erickson, Prasad, Levy, Jones, Shrestha, Mastracchio, Stodieck, Klaus, Zea and Chatterjee2018) suggested that microgravity-induced stress response genes also contribute to the increased resistance of bacteria to antibiotics in space conditions.
Overexpression of stress response genes and proteins have been observed in bacteria and fungi under simulated and space microgravity conditions (Leys et al., Reference Leys, Baatout, Rosier, Dams, s'Heeren, Wattiez and Mergeay2009; Mastroleo et al., Reference Mastroleo, van Houdt, Leroy, Benotmane, Janssen, Mergeay, Vanhavere, Hendrickx, Wattiez and Leys2009, Reference Mastroleo, van Houdt, Atkinson, Mergeay, Hendrickx, Wattiez and Leys2013; van Mulders et al., Reference van Mulders, Stassen, Daenen, Devreese, Siewers, van Eijsden, Nielsen, Delvaux and Willaert2011; Nicholson et al., Reference Nicholson, Moeller and Horneck2012; Vukanti and Leff, Reference Vukanti and Leff2012). In a study, Vukanti and Leff (Reference Vukanti and Leff2012) observed the over-expression of stress response genes (viz. csiD, cstA, katE, otsA and treA) in E.coli cells grown in simulated microgravity (clinorotation) compared to 1 × g controls. Proteins involved in response to carbon limitation and oxidative stress were differentially expressed in C. metallidurans grown aboard the ISS (Leys et al., Reference Leys, Baatout, Rosier, Dams, s'Heeren, Wattiez and Mergeay2009). Orsini et al. (Reference Orsini, Lewis and Rice2017) reported up- and down-regulation (both ≥2-fold) of 153 and 94 genes, respectively, in Streptococcus mutans grown under simulated microgravity conditions. Among others, genes of extrachromosomal elements, carbohydrate metabolism, translation and stress responses were affected (Orsini et al., Reference Orsini, Lewis and Rice2017). Investigations have shown the induction of various ROS scavengers (e.g. superoxide dismutase (SOD)) and redox-active proteins (e.g. thioredoxin, catalase, peroxiredoxin, thiol peroxidase, sulfoxide reductase MsrA) in space-exposed Bacillus spores, E. coli, Rhodospirillum rubrum and S. typhimurium, indicating the up-regulation of antioxidant defence mechanisms to cope with the space-induced oxidative damage occurring during long-term spaceflight (Wilson et al., Reference Wilson, Ott, Quick, Davis, zu Bentrup, Crabbé, Richter, Sarker, Barrila, Porwollik, Cheng, McClelland, Tsaprailis, Radabaugh, Hunt, Shah, Nelman-Gonzalez, Hing, Parra, Dumars, Norwood, Bober, Devich, Ruggles, CdeBaca, Narayan, Benjamin, Goulart, Rupert, Catella, Schurr, Buchanan, Morici, McCracken, Porter, Pierson, Smith, Mergeay, Leys, Stefanyshyn-Piper, Gorie, Nickerson and Ausubel2008; Mastroleo et al., Reference Mastroleo, van Houdt, Leroy, Benotmane, Janssen, Mergeay, Vanhavere, Hendrickx, Wattiez and Leys2009; Nicholson et al., Reference Nicholson, Moeller and Horneck2012; Vaishampayan et al., Reference Vaishampayan, Rabbow, Horneck and Venkateswaran2012). Increased synthesis of protective carotenoids has been reported in simulated-microgravity-exposed H. argentinensis against the oxidative damage caused by the harmful effects of stress (Thombre et al., Reference Thombre, Shinde, Dixit, Jagtap and Vidyasagar2017). Studies also show that cells activate pathways similar to those induced during osmotic stress and produce osmoprotective compounds (e.g. compatible solutes) to increase osmotolerance and survive in the space environment (Li et al., Reference Li, Chang, Xu, Chen, Su, Guo, Chen, Wang, Wang, Wang, Fang and Liu2015; Zhang et al., Reference Zhang, Fang and Liu2015; Milojevic and Weckwerth, Reference Milojevic and Weckwerth2020).
Noticeable effects on the growth kinetics, spores, mycelia formation and morphogenic switches have been noted in the fungal pathogen C. albicans under modelled microgravity conditions (rotary cell culture system) as well (Jiang et al., Reference Jiang, Xu, Yi, Huang, Li, Jiang, Zhou, Zhang and Cui2014). Differential regulation of genes involved in transcriptional regulation, ABC transport, cell aggregation and resistance against antifungals and oxidative stress, among others, have been observed in spaceflight-subjected C. albicans compared to ground controls (Crabbé et al., Reference Crabbé, Nielsen-Preiss, Woolley, Barrila, Buchanan, McCracken, Inglis, Searles, Nelman-Gonzalez, Ott, Wilson, Pierson, Stefanyshyn-Piper, Hyman, Nickerson and Coste2013). Genes were found to be differentially expressed. Also, increased random budding was noted in spaceflight-cultured cells compared to bipolar budding in 1 × g samples (Crabbé et al., Reference Crabbé, Nielsen-Preiss, Woolley, Barrila, Buchanan, McCracken, Inglis, Searles, Nelman-Gonzalez, Ott, Wilson, Pierson, Stefanyshyn-Piper, Hyman, Nickerson and Coste2013). Random budding and induction of stress-responsive proteins involved in stress/protein folding and oxidative stress have also been detected in S. cerevisiae in a space mission (Soyuz TMA-9) (van Mulders et al., Reference van Mulders, Stassen, Daenen, Devreese, Siewers, van Eijsden, Nielsen, Delvaux and Willaert2011). In contrast to bacteria, the SOD and peroxiredoxin were found to be down-regulated in S. cerevisiae, indicating a shift of the culture towards anaerobiosis in microgravity (van Mulders et al., Reference van Mulders, Stassen, Daenen, Devreese, Siewers, van Eijsden, Nielsen, Delvaux and Willaert2011). The up-regulation of genes involved in anaerobic metabolism has also been observed in P. aeruginosa during spaceflight (Crabbé et al., Reference Crabbé, Schurr, Monsieurs, Morici, Schurr, Wilson, Ott, Tsaprailis, Pierson, Stefanyshyn-Piper and Nickerson2011).
Microbial biofilm formation is also reported to be altered in microgravity. Increased number of viable cells, thick and higher biomass biofilms, differences in biofilm-forming extracellular polymeric substances, cell aggregation and cell clumping, and differential expression of genes involved in cell aggregation and biofilm formation are observed in different bacterial biofilms in microgravity (Wilson et al., Reference Wilson, Ott, zu Bentrup, Ramamurthy, Quick, Porwollik, Cheng, McClelland, Tsaprailis, Radabaugh, Hunt, Fernandez, Richter, Shah, Kilcoyne, Joshi, Nelman-Gonzalez, Hing, Parra, Dumars, Norwood, Bober, Devich, Ruggles, Goulart, Rupert, Stodieck, Stafford, Catella, Schurr, Buchanan, Morici, McCracken, Allen, Baker-Coleman, Hammond, Vogel, Nelson, Pierson, Stefanyshyn-Piper and Nickerson2007; Crabbé et al., Reference Crabbé, Schurr, Monsieurs, Morici, Schurr, Wilson, Ott, Tsaprailis, Pierson, Stefanyshyn-Piper and Nickerson2011, Reference Crabbé, Nielsen-Preiss, Woolley, Barrila, Buchanan, McCracken, Inglis, Searles, Nelman-Gonzalez, Ott, Wilson, Pierson, Stefanyshyn-Piper, Hyman, Nickerson and Coste2013; Kim et al., Reference Kim, Tengra, Young, Shong, Marchand, Chan, Pangule, Parra, Dordick, Plawsky and Collins2013b). Transcriptome analysis showed that spaceflight conditions caused up-regulation of biofilm-related genes in B. subtilis (Morrison et al., Reference Morrison, Fajardo-Cavazos and Nicholson2019). Space-exposed cells of the human pathogen Klebsiella pneumoniae exhibited increased biofilm formation, which is an essential characteristic of virulence (Li et al., Reference Li, Liu, Wang, Ge, Woo, Yan, Zhao, Gao, Liu and Liu2014). Increased tolerance to salt and ethanol treatments and antibiotic resistance has also been reported in microbes of biofilms under microgravity conditions (Lynch et al., Reference Lynch, Mukundakrishnan, Benoit, Ayyaswamy and Matin2006; Tucker et al., Reference Tucker, Ott, Huff, Fofanov, Pierson, Willson and Fox2007).
The extracellular environmental signals and stress factors, like heat, nutrients, chemicals, osmotic stress and shear stress, greatly influence the production of secondary metabolites in microbes (Gao et al., Reference Gao, Fang, Pierson, Mishra and Demain2001, Reference Gao, Liu and Zhang2011; Viollier et al., Reference Viollier, Kelemen, Dale, Nguyen, Buttner and Thompson2003; Bibb, Reference Bibb2005). Considering the immense impact of space environmental conditions on microbial growth and physiology, several research groups investigated the production of secondary metabolites of commercial interest under stressful microgravity conditions (Table 1) (Horneck et al., Reference Horneck, Klaus and Mancinelli2010; Huang et al., Reference Huang, Li, Huang and Liu2018; Prasad et al., Reference Prasad, Richter, Vadakedath, Mancinelli, Krüger, Strauch, Grimm, Darriet, Chapel, Cohen and Lebert2020). Very interesting and diverse results of secondary metabolites yields (e.g. antibiotics, bioactive compounds, anti-tumour agents, vaccines, immunosuppressant, etc.) have been reported (Lam et al., Reference Lam, Mamber, Pack, Forenza, Fernandes and Klaus1998, Reference Lam, Gustavson, Pirnik, Pack, Bulanhagui, Mamber, Forenza, Stodieck and Klaus2002; Demain and Fang, Reference Demain and Fang2001; Horneck et al., Reference Horneck, Klaus and Mancinelli2010; Gao et al., Reference Gao, Liu and Zhang2011; Huang et al., Reference Huang, Li, Huang and Liu2018). An increase in the antibiotic monorden's specific productivity by Humicola fuscoatra WC5157 has been found in solid-state fermentation carried on T8 and PG agar media aboard the Space Shuttle mission STS-77 (Lam et al., Reference Lam, Mamber, Pack, Forenza, Fernandes and Klaus1998). The production of monorden in the T8 and PG agar medium increased up to 30 and 190%, respectively, in spaceflight conditions compared to ground control (Lam et al., Reference Lam, Mamber, Pack, Forenza, Fernandes and Klaus1998). The group has also reported an increased production of the anticancer agent actinomycin D by Streptomyces plicatus WC56452 grown in defined and complex media under dark, anaerobic and thermally controlled (20 °C) conditions onboard the Space Shuttle mission STS-80 (Lam et al., Reference Lam, Gustavson, Pirnik, Pack, Bulanhagui, Mamber, Forenza, Stodieck and Klaus2002). In the study by Benoit et al. (Reference Benoit, Li, Stodieck, Lam, Winther, Roane and Klaus2006), increased production of actinomycin D by S. plicatus was noted only on days 8 and 12 during the space mission, while decreased production was noted in all subsequent sample days (4 days interval for 72 days) compared to the ground controls. In a study, Huang et al. (Reference Huang, Liu, Rong, Ruan and Huang2015) investigated the growth, morphological and secondary metabolic responses of S. coelicolor exposed to simulated (2D-clinostat) and real (Shenzhou-8 space mission) microgravity conditions. They observed morphological and secondary metabolite production changes and increased bacteriostatic activity of the culture against B. subtilis in microgravity environments, indicating that S. coelicolor A3(2) produces more bioactive substances under microgravity conditions. In the study of Luo et al. (Reference Luo, Gao, Song, Tan and Liu1998), the antifungal agent nikkomycin production by space-exposed strains of Streptomyces ansochromogenus (15 days of spaceflight aboard a satellite) was found to be increased by 13–18%. Enhanced production of the toxin microcystin by the cyanobacteria Microcystis aeruginosa under simulated microgravity conditions has also been demonstrated (Xiao et al., Reference Xiao, Liu, Wang, Hao and An2010).
Table 1. Examples of observed changes or products of commercial interest derived by exposing microbes and plants to the real space environment
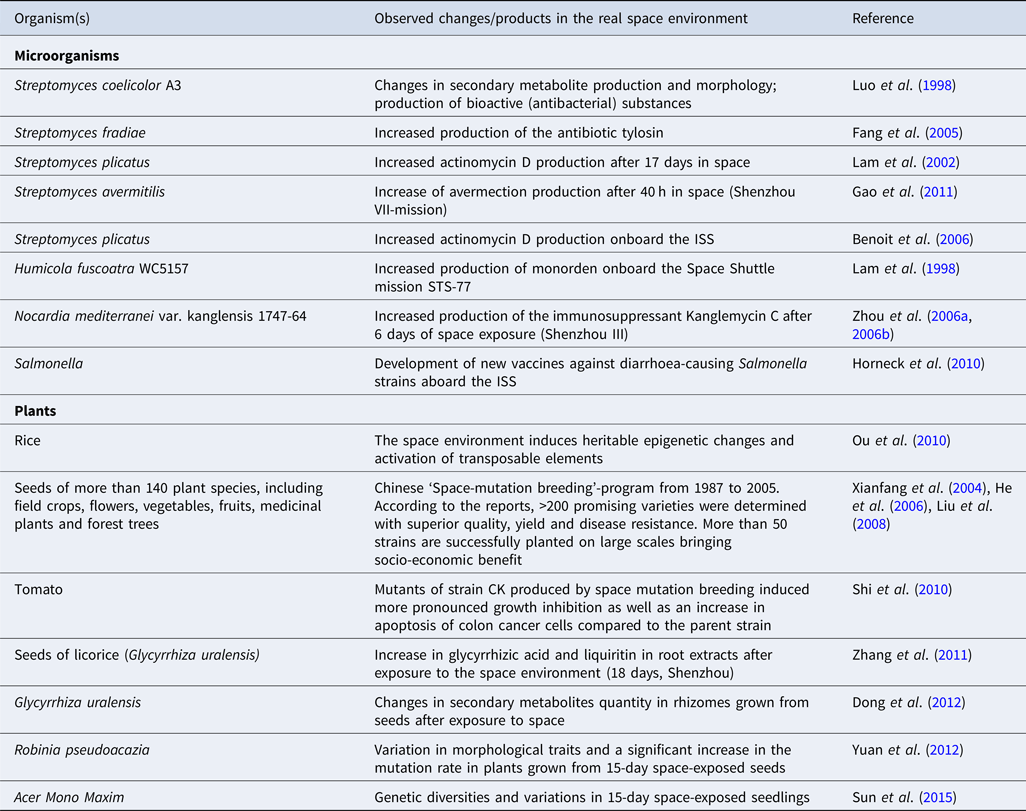
Investigations under LSMMG conditions have shown decreased production of metabolites, such as the β-lactam antibiotic (cephalosporin C) and polyketide macrolide (rapamycin) by Streptomyces sp. (Fang et al., Reference Fang, Pierson, Mishra, Koenig and Demain1997c, Reference Fang, Pierson, Mishra and Demain2000). In another study, Fang et al. (Reference Fang, Pierson, Koenig, Mishra and Demain1997a) demonstrated a decreased production of the peptide antibiotic microcin B17 by E. coli ZK650 under LSMMG condition (high aspect rotating vessel (HARV)). Also, cultivation in the HARV led to extracellular production of microcin compared to the intracellular production in control shake flasks. de Gelder et al. (Reference de Gelder, Vandenabeele, de Boever, Mergeay, Moens and de Vos2009) demonstrated increased production of poly-β-hydroxybutyrate by C. metallidurans LMG 1195 after 24 h of cultivation under LSSMG conditions but reduction after 48 h of culture compared to 1 × g controls, indicating fluctuation of metabolite production in microgravity. No simulated-microgravity-related changes in the production of secondary metabolites (e.g. gramicidin S) have also been reported (Fang et al., Reference Fang, Pierson, Mishra, Koenig and Demain1997b).
The variation in microbial secondary metabolite production (increased, decreased, fluctuate over time or uninfluenced) under microgravity conditions is mainly attributed to differences in cell strains, medium, fermentation processes and conditions and extracellular microenvironment, as well as microgravity-related physical effects on fluid dynamics, secretion and transport of metabolites (Benoit et al., Reference Benoit, Li, Stodieck, Lam, Winther, Roane and Klaus2006; Horneck et al., Reference Horneck, Klaus and Mancinelli2010; Huang et al., Reference Huang, Li, Huang and Liu2018).
Collectively, studies have provided substantial evidence on the effect of microgravity on microbial growth, behaviour, virulence, pathogenicity, resistance and metabolite production. Future experiments in space and space analogue conditions employing comparative omics techniques (genomics, transcriptomics, proteomics and metabolomics) and bioinformatics tools will further enhance our understanding of the molecular mechanisms of microgravity-induced alterations in microorganisms. In-depth knowledge of space-induced changes can be utilized to engineer microbes with new properties and develop new approaches to produce antibiotics and other metabolites of commercial interest in terrestrial facilities more efficiently, as well as establish novel treatment strategies for preventing and controlling infectious diseases on Earth (Benoit et al., Reference Benoit, Li, Stodieck, Lam, Winther, Roane and Klaus2006; Horneck et al., Reference Horneck, Klaus and Mancinelli2010; Aunins et al., Reference Aunins, Erickson, Prasad, Levy, Jones, Shrestha, Mastracchio, Stodieck, Klaus, Zea and Chatterjee2018; Milojevic and Weckwerth, Reference Milojevic and Weckwerth2020).
Effect of microgravity on plants
Whole plants and their parts (organs, tissues, cells and protoplast cultures) have been studied in several space experiments onboard satellites, space shuttles and orbital stations, as well as under simulated microgravity conditions on Earth to elucidate gravity-related changes. These experiments demonstrate that changes in gravity (microgravity and hypergravity) affects the growth, development and phenotype of plants and enhance our understanding of cell-division processes, gravity perception, signal transduction and gravity-induced distribution of organelles in plants (Kiss, Reference Kiss2000; Ferl et al., Reference Ferl, Wheeler, Levine and Paul2002; Hemmersbach and Braun, Reference Hemmersbach and Braun2006; Stutte et al., Reference Stutte, Monje, Hatfield, Paul, Ferl and Simone2006; Paul et al., Reference Paul, Amalfitano and Ferl2012a, Reference Paul, Zupanska, Ostrow, Zhang, Sun, Li, Shanker, Farmerie, Amalfitano and Ferl2012b; Correll et al., Reference Correll, Pyle, Millar, Sun, Yao, Edelmann and Kiss2013; Kordyum and Chapman, Reference Kordyum and Chapman2017).
Morphological, anatomical and histological changes have been observed in plants grown in the space environment. Arabidopsis grown on the ISS was found to be smaller with abnormal root growth than 1 × g controls (Millar et al., Reference Millar, Johnson, Edelmann and Kiss2011; Paul et al., Reference Paul, Amalfitano and Ferl2012a). Arabidopsis seedlings germinated and grown under real microgravity in space were reported to show a significant decrease in ribosome production (Matía et al., Reference Matía, González-Camacho, Herranz, Kiss, GASSET, van Loon, MARCO and Javier Medina2010). Compared to 1 × g-samples, cell proliferation rate increased, but cell growth decreased. The authors suggest that microgravity affects cell cycle regulation and leads to a shortened G2 period (Matía et al., Reference Matía, González-Camacho, Herranz, Kiss, GASSET, van Loon, MARCO and Javier Medina2010). Leaf ultrastructure and chloroplast shape were also found to be different in dwarf wheat grown under microgravity (Stutte et al., Reference Stutte, Monje, Hatfield, Paul, Ferl and Simone2006). Changes in the actin cytoskeleton organization involved in transporting cell wall components to the cell periphery have also been noted under microgravity conditions (Correll et al., Reference Correll, Pyle, Millar, Sun, Yao, Edelmann and Kiss2013). In a study by Kamal et al. (Reference Kamal, Herranz, van Loon and Medina2018), alteration in the cell cycle (lower fraction of cells in G1-phase), levels of proteins involved in cell-cycle regulation and nucleolus and fibrillar structures were observed under simulated microgravity or Martian gravity.
Significant changes in the transcriptome and proteome of Arabidopsis under microgravity conditions are recorded (Martzivanou and Hampp, Reference Martzivanou and Hampp2003; Martzivanou et al., Reference Martzivanou, Babbick, Cogoli-Greuter and Hampp2006; Paul et al., Reference Paul, Zupanska, Ostrow, Zhang, Sun, Li, Shanker, Farmerie, Amalfitano and Ferl2012b, Reference Paul, Zupanska, Schultz and Ferl2013; Correll et al., Reference Correll, Pyle, Millar, Sun, Yao, Edelmann and Kiss2013; Hausmann et al., Reference Hausmann, Fengler, Hennig, Franz-Wachtel, Hampp, Neef and Legué2014; Fengler et al., Reference Fengler, Spirer, Neef, Ecke, Nieselt and Hampp2015, Reference Fengler, Spirer, Neef, Ecke, Hauslage and Hampp2016; Schüler et al., Reference Schüler, Hemmersbach and Böhmer2015). Among others, genes involved in reactive oxygen pathways, stress response, cell wall remodelling, diverse metabolic pathways and calcium signalling were found to be differentially expressed. Studies with Arabidopsis callus cells also show that acceleration or microgravity induces changes in gene expression, protein translation and modification and metabolites production (Martzivanou and Hampp, Reference Martzivanou and Hampp2003; Babbick et al., Reference Babbick, Dijkstra, Larkin, Anthony, Davey, Power, Lowe, Cogoli-Greuter and Hampp2007; Barjaktarović et al., Reference Barjaktarović, Schütz, Madlung, Fladerer, Nordheim and Hampp2009a, Reference Barjaktarović, Babbick, Nordheim, Lamkemeyer, Magel and Hampp2009b; Hausmann et al., Reference Hausmann, Fengler, Hennig, Franz-Wachtel, Hampp, Neef and Legué2014; Neef et al., Reference Neef, Ecke and Hampp2015). Mainly, changes in proteins involved in primary metabolism, carbohydrate metabolism (e.g. glyceraldehyde-3-phosphate dehydrogenase, triose-phosphate-isomerase), signalling, radical scavenging, detoxification of ROS and chaperone functions (HSP70) were found (Barjaktarović et al., Reference Barjaktarović, Babbick, Nordheim, Lamkemeyer, Magel and Hampp2009b). Both short- and long-term microgravity exposure influences the expression of genes of ROS-related enzymes, such as ascorbate and glutathione peroxidases, calcium-dependent signalling, stress response and detoxification (Salmi and Roux, Reference Salmi and Roux2008; Barjaktarović et al., Reference Barjaktarović, Schütz, Madlung, Fladerer, Nordheim and Hampp2009a, Reference Barjaktarović, Babbick, Nordheim, Lamkemeyer, Magel and Hampp2009b; Salmi et al., Reference Salmi, Ul Haque, Bushart, Stout, Roux and Porterfield2011; Paul et al., Reference Paul, Zupanska, Ostrow, Zhang, Sun, Li, Shanker, Farmerie, Amalfitano and Ferl2012b, Reference Paul, Zupanska, Schultz and Ferl2013; Hausmann et al., Reference Hausmann, Fengler, Hennig, Franz-Wachtel, Hampp, Neef and Legué2014).
Alterations in expression of genes involved in detoxification, biotic-induced stress, water stress, oxidative stress, salt stress and other stress responses have also been observed in Arabidopsis thaliana seedlings (Paul et al., Reference Paul, Zupanska, Ostrow, Zhang, Sun, Li, Shanker, Farmerie, Amalfitano and Ferl2012b, Reference Paul, Zupanska, Schultz and Ferl2013; Correll et al., Reference Correll, Pyle, Millar, Sun, Yao, Edelmann and Kiss2013). Changes in genes coding for proteins involved in pathogen response, touch-wounding response, stress response (cold and drought stress), cell wall remodelling, growth, auxin-mediated root development, signal transduction, etc., were found in seedlings exposed to the space environment. Several experiments have shown the effects of acceleration changes on protein synthesis and gene expression in other plants as well (Ceratopteris richardii, Solanum lycopersicum, dwarf wheat and Oryza sativa) (Stutte et al., Reference Stutte, Monje, Hatfield, Paul, Ferl and Simone2006; Salmi and Roux, Reference Salmi and Roux2008; Bushart et al., Reference Bushart, Cannon, Ul Haque, San Miguel, Mostajeran, Clark, Porterfield and Roux2013; Jin et al., Reference Jin, Chen and Cai2015). These studies observed alteration in genes involved in the cell wall structure, primary metabolism, transcription, calcium regulation and physiological and metabolic processes (protein kinases, oxidoreductase, transferases, hydrolases, etc.), among others.
Studies have shown that altered gravity produces significant epigenetic changes in plants (Kamal et al., Reference Kamal, Herranz, van Loon and Medina2018; Xu et al., Reference Xu, Chen, Jin and Cai2018; Zhou et al., Reference Zhou, Sng, LeFrois, Paul and Ferl2019). In a recent study, Zhou et al. (Reference Zhou, Sng, LeFrois, Paul and Ferl2019) found that 20% of the protein-coding genes to be differentially methylated in leaves of Arabidopsis subjected to real microgravity conditions aboard the ISS. Determination of DNA methylation in Arabidopsis’ cell culture showed that artificial microgravity and Martian gravity increased DNA methylation levels (Kamal et al., Reference Kamal, Herranz, van Loon and Medina2018). In the study by Ou et al. (Reference Ou, Long, Wu, Yu, Lin, Qi and Liu2010), both genetic changes and hypo- and hyper-methylation (CG and CNG) were observed in rice plants grown from space-flown seeds. The frequency of genetic changes ranged from 0.7 to 6.7% across investigated plants, while hypomethylation frequency was found to be 0.76% (CG) and 0.80% (CNG). The hypermethylation was 1.95% (CG) and 1.44% (CNG) (Ou et al., Reference Ou, Long, Wu, Yu, Lin, Qi and Liu2010). Analysis of Arabidopsis seedlings flown onboard of an SJ-10 recoverable satellite for 60 h revealed that among others, genes involved in metabolic pathways, biosynthesis of secondary metabolites and starch and sucrose metabolism were methylated in microgravity (Xu et al., Reference Xu, Chen, Jin and Cai2018), suggesting epigenetic modifications in plants under space environment.
Available literature data strongly indicate that the space environment can also induce heritable and stable DNA changes in plants (both positive and negative mutations), resulting in genetically diverse strains (Table 1) (Cowles et al., Reference Cowles, LeMay and Jahns1994; Dutcher et al., Reference Dutcher, Hess and Halstead1994; Nechitailo et al., Reference Nechitailo, Jinying, Huai, Yi, Chongqin and Min2005; He et al., Reference He, Liu, Lu, Xue, Pan and Teixeira da Silva2006; Liu et al., Reference Liu, Guo, Zhao, Gu and Zhao2008; Gao et al., Reference Gao, Li, Yan, Gao and Hu2009; Wu et al., Reference Wu, Huang, Zhang and Sun2010; Chengzhi, Reference Chengzhi2011; Dong et al., Reference Dong, Gao, Zhang, Zuo and Huang2012; Yuan et al., Reference Yuan, Li, Sun, Sun, Zhang, Yang, Zhang, Li and Wang2012). In the course of a ‘Space-mutation breeding’ program, Chinese scientists sent seeds of crops, floriculture, vegetable, fruits, medicinal plants and forest trees to space (Xianfang et al., Reference Xianfang, Long, Weixu and Chunhua2004; He et al., Reference He, Liu, Lu, Xue, Pan and Teixeira da Silva2006; Liu et al., Reference Liu, Guo, Zhao, Gu and Zhao2008). According to He et al. (Reference He, Liu, Lu, Xue, Pan and Teixeira da Silva2006) report, more than 200 varieties of plants exhibiting promising properties, such as high yield, resistance to pathogens and good quality, were obtained from the space-exposed seeds. A remarkable increase in potency and germination rate was found in space-mutated seeds of wheat, maize, barley, triticale, soybean, sunflower, cucumber, tomato and cotton, while no significant difference was noted in rice, pea, millet, lettuce, sweet pepper and tobacco. The seed germination rate was also found to be reduced in seeds of sorghum, watermelon, eggplant, radish and towel gourd (Dutcher et al., Reference Dutcher, Hess and Halstead1994; Liu et al., Reference Liu, Guo, Zhao, Gu and Zhao2008). These indicate that the effect of spaceflight differs between different species or strains of plants. Flowering plants (purple shamrock, scarlet sage, garden petunia, orchids) with brighter flowers, unpredictable flower colour pattern and more extended flowering periods and more tolerant to insects and pathogens as well as vegetables (chili pepper, cucumber, eggplant, tomato and lotus) with higher yields and contents of vitamins and elements have been developed through the space-induced mutation breading strategy (Li et al., Reference Li, Wang, Zhang and Jiang1999; Yan et al., Reference Yan, Li and Lei2002; Huai et al., Reference Huai, Min, Jinying, Yi and Chunhua2005; He et al., Reference He, Liu, Lu, Xue, Pan and Teixeira da Silva2006; Liu et al., Reference Liu, Guo, Zhao, Gu and Zhao2008). China has officially approved several new varieties of crops, including rice, wheat, pepper, tomato, alfalfa, sesame and cotton, developed by space breeding techniques and successfully planted on large scales (He et al., Reference He, Liu, Lu, Xue, Pan and Teixeira da Silva2006; Liu et al., Reference Liu, Guo, Zhao, Gu and Zhao2008).
Yuan et al. (Reference Yuan, Li, Sun, Sun, Zhang, Yang, Zhang, Li and Wang2012) observed higher levels of genetic diversity as determined by simple sequence repeat and sequence-related amplified polymorphism molecular marker analyses and also apparent variation in morphological traits (height, basal diameter, number of branches, leaflet vertex angle and tippy leaf vertex angle, among others) were observed in the space-mutagenized black locust plants (Robinia pseudoacacia, a forest legume) from seeds that endured 15-day spaceflight compared to plants from parallel ground-based control seeds (Yuan et al., Reference Yuan, Li, Sun, Sun, Zhang, Yang, Zhang, Li and Wang2012). Genetic diversities and variations have also been observed in 15-day space-exposed Acer Mono Maxim seedlings than ground control seedlings (Sun et al., Reference Sun, Zhang, Yuan, Yang, Long, Li and Yang2015). Inheritable changes in proteins and phenotypes have been observed in rice mutants grown from seeds subjected to short-term (15 days) spaceflight conditions (Lu et al., Reference Lu, Wang, Zheng, Guan, Xin and Y2008). In a study on the effects of long-term exposure to spaceflight physical factors on tomato plants grown from dormant seeds flown for 6 years aboard the space station MIR, Nechitailo et al. (Reference Nechitailo, Jinying, Huai, Yi, Chongqin and Min2005) found that upon return to the Earth, the seeds germinated and grew to maturity. Significant differences in growth, development, yield and molecular changes were observed between plants (first and second generation) from space-exposed seeds and ground-based controls (Nechitailo et al., Reference Nechitailo, Jinying, Huai, Yi, Chongqin and Min2005). Of the 12 cauliflowers plants grown from space-exposed seeds, two first-generation plants showed significant phenotypical changes in plant size and flower head weight (Wu et al., Reference Wu, Huang, Zhang and Sun2010). Also, the space-flown cauliflowers plants were found to be resistant to the black rot, suggesting that space-induced mutagenesis has the potential to result in disease-resistant strains as well. Similar mutations were observed in plants grown from seeds obtained from first-generation plants (Wu et al., Reference Wu, Huang, Zhang and Sun2010). However, in the case of sprout broccoli, the rate of emergence from the flown seeds was 30% lower than the control (Wu et al., Reference Wu, Huang, Zhang and Sun2010). Spaceflight-induced heritable mutations have been observed in different rice varieties grown from 6-day spaceflight-subjected seeds (Shenzhou-3) (Yu et al., Reference Yu, Wu, Wei, Cheng, Xin, Huang, Zhang and Sun2007). Changes in the height of plants, colour, shape and angle of leaves, panicle length and type and rice shape and colour, among others, were observed in the first and successive generations of plants (Yu et al., Reference Yu, Wu, Wei, Cheng, Xin, Huang, Zhang and Sun2007). These studies collectively show that DNA changes have occurred in the space-flown seeds, and some of the changes are inherited from the first to successive generations.
Alterations in the flower colour (golden, yellow, light yellow or yellowish-green), ligulate petals of the unisexual floret (broadened or thin), short tubular petals of bisexual floret (elongated or turned into semi-ligulate or ligulate petals) and shape and thickness of leaves were observed in the plants grown from space-subjected seeds(Yang et al., Reference Yang, Shen, Zhang, Chen, Liu, Ma, Chen and Peng2012). Also, sequence analysis showed changes in a DNA sequence by deletion, insertion and replacement, indicating the high mutagenic efficacy of space flight (Yang et al., Reference Yang, Shen, Zhang, Chen, Liu, Ma, Chen and Peng2012). Significant changes in the rate of DNA variation (6.34%) as well as various morphological (increase in the plant height, number of leaves and fresh weight) and anatomical modification were observed in space-flown rose seedlings compared to ground controls (Huai et al., Reference Huai, Min, Jinying, Yi and Chunhua2005). Ultrastructural alterations included twist, contraction, deformation of the cell wall, curvature and loose arrangement of lamellae of some chloroplasts and an increase in the number of starch grains per chloroplast (Huai et al., Reference Huai, Min, Jinying, Yi and Chunhua2005).
Taken together, a growing body of evidence shows the significant impact of the space environment on the genetic integrity, epigenetics, gene expression, physiology, metabolite production and morphology of plants. Studies demonstrate that the microgravity environment acts as a stressor and induce heritable mutagenic changes in plants. The space-induced mutation technique can be used as a novel method to support the emergence of new varieties of plants and develop and enhance characters of crops, such as yield, quality, short-growing period, resistance to pathogens, etc.
Effects of ionizing radiation on microorganisms
The effect of space ionizing radiation on microorganisms’ response is not as widely studied as the effects of microgravity. An in-depth understanding of the impact of space ionizing radiation on different microbes is essential from the perspective of life-support systems for space exploration as well as to create novel microbial strains for agricultural and biotechnological applications. Studies show that ionizing radiation produces negative or positive effects that mainly depend on radiation (type, quality, dose and duration) and the microbe itself (species, shape, structure, growth stage, metabolism and genome). On exposure to ionizing radiation, alterations in genetics, metabolite expression, physiology and phenotype have been observed in microbes (Fukuda et al., Reference Fukuda, Fukuda, Takahashi, Ohnishi, Nakano, Sato and Gunge2000; Yatagai et al., Reference Yatagai, Saito, Takahashi, Fujie, Nagaoka, Sato and Ohnishi2000; Kimura et al., Reference Kimura, Ishidou, Kurita, Suzuki, Shibato, Rakwal and Iwahashi2006; Novikova et al., Reference Novikova, de Boever, Poddubko, Deshevaya, Polikarpov, Rakova, Coninx and Mergeay2006; Mastroleo et al., Reference Mastroleo, van Houdt, Leroy, Benotmane, Janssen, Mergeay, Vanhavere, Hendrickx, Wattiez and Leys2009; Senatore et al., Reference Senatore, Mastroleo, Leys and Mauriello2018).
The whole-genome oligonucleotide microarray analysis and high throughput gel-free proteomics of the α-proteobacterium Rhodospirillum rubrum S1H sent twice to the ISS revealed that a low dose (2 mGy) of simulated ISS-ionizing radiation (a combination of single beams of low-LET γ rays and high-LET neutron rays) caused significant changes at the transcriptomic level but only a few considerable variations in the expression of proteins, and no alteration in cell viability (Mastroleo et al., Reference Mastroleo, van Houdt, Leroy, Benotmane, Janssen, Mergeay, Vanhavere, Hendrickx, Wattiez and Leys2009).
Spores are highly resistant to a wide range of stressors, including extreme temperatures, radiation, pressure, vacuum, oxidizing agents and chemicals. Nicholson et al. (Reference Nicholson, Fajardo-Cavazos, Rebeil, Slieman, Riesenman, Law and Xue2002) used B. subtilis strains defective in various DNA repair systems and spore structural components to investigate the types of DNA damage induced by sunlight UV-B and UV-A components. The strains were also used to determine the role of spore DNA repair systems and spore structural components in resisting the mutagenic and lethal effects of solar UV radiation. They concluded that B. subtilis spores employ a wide range of protective and repair mechanisms that enable them to survive the stresses of solar UV radiation exposure. Long-term exposure (nearly 6 years) of B. subtilis spores in monolayers or multilayers to the space environment showed that solar UV radiation reduced spores’ survival, but up to 104 viable spores were still recovered (Horneck et al., Reference Horneck, Bücker and Reitz1994). The study also reported the survival of 70% of the multilayered spores exposed to space vacuum and protected against solar UV radiation. B. pumilus spores were found to be highly resistant to the open space environment as well as simulated Martian atmospheric conditions aboard the ISS (Vaishampayan et al., Reference Vaishampayan, Rabbow, Horneck and Venkateswaran2012). The survival rate of spores was found to be between 10 and 40% in open space and between 85 and 100% under simulated Martian conditions. The proteomic analysis also showed an increase of proteins inducing stress tolerance (e.g. SOD) in space-exposed samples compared to ground controls (Vaishampayan et al., Reference Vaishampayan, Rabbow, Horneck and Venkateswaran2012). Also, the first-generation cells and spores derived from space-exposed samples exhibited high resistance to UV-C than ground control samples (Vaishampayan et al., Reference Vaishampayan, Rabbow, Horneck and Venkateswaran2012).
Many fungal species also exhibit high resistance to radiation. In a study, Kimura et al. (Reference Kimura, Ishidou, Kurita, Suzuki, Shibato, Rakwal and Iwahashi2006) analysed the effect of ionizing radiation (X- and γ-rays) on the transcriptional response of S. cerevisiae strain S288C by cDNA microarray analysis and revealed a time-course transcriptional profile of changed gene expressions. They noted the up-regulation of genes mainly related to cell cycle and DNA processing, cell rescue defence and virulence, protein and cell fate and metabolism in irradiated cells (50 Gy dose of ionizing radiation). The down-regulated genes primarily included transcription and protein synthesis, cell cycle and DNA processing, control of the cellular organization, cell fate and C-compound and carbohydrate metabolism categories (Kimura et al., Reference Kimura, Ishidou, Kurita, Suzuki, Shibato, Rakwal and Iwahashi2006). Space-related conditions of UV-C irradiation and vacuum induced significant stearic acid production, a biofilm-associated compound, in the radioresistant bacterium D. radiodurans, which may have led to cell survivability and maintenance of cell membrane integrity under stress conditions (Ott et al., Reference Ott, Kawaguchi, Kölbl, Chaturvedi, Nakagawa, Yamagishi, Weckwerth and Milojevic2017). This compound is generally produced in lower quantities under non-stressed conditions (Melin et al., Reference Melin, Peuchant, Perromat and Clerc1998). The integrated proteomic and metabolomic analysis also revealed molecular alterations in several metabolic and stress response pathways, including the tricarboxylic acid cycle, the DNA damage response systems, ROS scavenging systems and transcriptional regulators to cope with the space-related stressors (Ott et al., Reference Ott, Kawaguchi, Kölbl, Chaturvedi, Nakagawa, Yamagishi, Weckwerth and Milojevic2017).
A survey of environmental biocontamination aboard the ISS showed the presence of several fungal and bacterial species (opportunistic) pathogens, and strains degrading structural materials (Novikova et al., Reference Novikova, de Boever, Poddubko, Deshevaya, Polikarpov, Rakova, Coninx and Mergeay2006). The study suggested that the survivability of the microorganisms in the extreme environment of space is due to the observed physiological and morphological changes (Novikova et al., Reference Novikova, de Boever, Poddubko, Deshevaya, Polikarpov, Rakova, Coninx and Mergeay2006). A long-term spaceflight experiment performed aboard the Russian space station Mir exposed to space radiation containing high-LET of 10–21 mGy (0.25–0.51 mGy day−1) revealed that space-subjected samples had higher mutation frequency than ground control samples of B. Subtilis and S. cerevisiae (Fukuda et al., Reference Fukuda, Fukuda, Takahashi, Ohnishi, Nakano, Sato and Gunge2000; Yatagai et al., Reference Yatagai, Saito, Takahashi, Fujie, Nagaoka, Sato and Ohnishi2000). No significant difference in the frequency of mutations in the ribosomal protein L (rpsL) gene leading to spectinomycin resistance (SpcR), but distinct differences in the spectrum of mutations leading to SpcR in B. subtilis spores were noted between spaceflight and ground control samples (Yatagai et al., Reference Yatagai, Saito, Takahashi, Fujie, Nagaoka, Sato and Ohnishi2000). In another study by Fukuda et al. (Reference Fukuda, Fukuda, Takahashi, Ohnishi, Nakano, Sato and Gunge2000), no significant difference in point mutation rates but total or large deletion in the bacterial ribosomal protein L (rpsL) gene were observed between space and ground samples, proposing that space radiation comprising high-LET caused deletion-type mutations. However, in the study by Takahashi et al. (Reference Takahashi, Ohnishi, Yokota, Kumagai, Nakano and Ohnishi2002), the mutation frequencies of space samples were not different from the ground control samples in plasmid DNA containing the rpsL gene as well as in both the repair-deficient and wild-type cells of E. coli strains, suggesting no effect of space radiation on the mutation frequency.
In the study of Zhou et al. (Reference Zhou, Sun, Wang, Gao, Bai, Zheng, You and Li2006a), the production of the immunosuppressant Kanglemycin C (K-C) by one of the mutant strain (F-16) derived from Nocardia mediterranei var. kanglensis strain U-7 spores exposed to space radiation during a spaceflight (Shenzhou III) was found to be increased up to 200% compared to the ground control strain U-7. In another mutant strain, F-210 derived from spaceflight-subjected spores of strain M-13, morphological and physiological changes and reduced K-C productivity were observed (Zhou et al., Reference Zhou, Sun, Wang, Gao, Bai, Zheng, You and Li2006a).
Studies as a whole indicate that space ionizing radiation exerts mutagenic effects (e.g. increase in mutation rates, variations in the spectrum of mutation) on microbial cells bringing alteration in genome organization, physiology and morphology. The observed effects or changes depend on the type of investigated species, the quality and quantity of ionizing radiation and the presence of other stress factors, e.g. microgravity, in the case of real space experiments.
Effect of ionizing radiation on plants
Several experiments have been performed in space and on Earth to analyse the effect of ionizing radiation on plants and elucidate the physiological mechanisms of tolerance to radiation. Ionizing radiation can alter genetics, growth, development and physiology of whole plants, seeds, meristems and germ cells (de Micco et al., Reference de Micco, Arena, Pignalosa and Durante2011; Arena et al., Reference Arena, de Micco, Macaeva and Quintens2014). The severity of the effect is dependent on several factors, including radiation type and dose and plant species, cultivar, age, physiology, morphology and genome organization (Holst and Nagel, Reference Holst, Nagel and Wang1997; Kim et al., Reference Kim, Kim, Goh, Kim, Kim, Seo, Jang and Kang2011; de Micco et al., Reference de Micco, Arena, Pignalosa and Durante2011; Arena et al., Reference Arena, de Micco, Macaeva and Quintens2014). Low doses of ionizing radiation can cause stimulatory effects, while intermediate to high doses can be harmful or detrimental (de Micco et al., Reference de Micco, Arena, Pignalosa and Durante2011; Arena et al., Reference Arena, de Micco, Macaeva and Quintens2014). The visible changes in plants upon exposure to ionizing radiation include alterations in the morphology (abnormal development, dwarf or tall phenotypes, increased pubescence, etc.), the anatomy of organs and reproductive capabilities (Wi et al., Reference Wi, Chung, Kim, Kim, Baek, Lee and Kim2007; de Micco et al., Reference de Micco, Arena, Pignalosa and Durante2011; Reference de Micco, Paradiso, Aronne, de Pascale, Quarto and Arena2014b; Arena et al., Reference Arena, de Micco, Macaeva and Quintens2014). The physiological and cytological modification include changes in photosynthetic machinery and efficiency of photosynthetic apparatus oxygen evolution, dilation of thylakoid membranes, accumulation of anthocyanin, phenolics and antioxidant compounds and variation of the antioxidative system (Kovács and Keresztes, Reference Kovács and Keresztes2002; Kim et al., Reference Kim, Chung, Kim and Wi2005; Reference Kim, Kim, Goh, Kim, Kim, Seo, Jang and Kang2011; Arena et al., Reference Arena, de Micco, Aronne, Pugliese, Virzo De Santo and de Maio2013). The photosynthetic machinery components that are mainly influenced by ionizing radiation include electron transport carriers, light-harvesting complexes and carbon reduction cycle enzymes (de Micco et al., Reference de Micco, Arena, Pignalosa and Durante2011; Fan et al., Reference Fan, Shi, Huang, Xu, Wang and Guo2014). The detrimental effects of deleterious mutations due to ionizing radiation include the death of embryonic cells, loss of reproduction, abnormal phenotypes, shortened lifespan, lower yield and increased vulnerability to diseases (de Micco et al., Reference de Micco, Arena, Pignalosa and Durante2011; Arena et al., Reference Arena, de Micco, Macaeva and Quintens2014).
Plants are more resistant to space ionizing radiation than animals and humans (Kumagai et al., Reference Kumagai, Katoh, Kumada, Tanaka, Tano and Miyazaki2000; Real et al., Reference Real, Sundell-Bergman, Knowles, Woodhead and Zinger2004; Arena et al., Reference Arena, de Micco, Macaeva and Quintens2014). This is most likely due to the structural properties (e.g. thick cell wall), presence of absorbing materials in the cuticle (e.g. phenolic substances and aliphatic organic molecules), genetic organization (e.g. polyploidy), higher efficiency in repairing DNA damages and/or higher rates of DNA methylation (Kranz et al., Reference Kranz, Gartenbach and Zimmermann1994; Shikazono et al., Reference Shikazono, Tanaka, Kitayama, Watanabe and Tano2002; Yokota et al., Reference Yokota, Shikazono, Tanaka, Hase, Funayama, Wada and Inoue2005; Esnault et al., Reference Esnault, Legue and Chenal2010; Pecinka and Mittelsten Scheid, Reference Pecinka and Mittelsten Scheid2012; Arena et al., Reference Arena, de Micco, Macaeva and Quintens2014; de Micco et al., Reference de Micco, Arena and Aronne2014a; Beysens and van Loon, Reference Beysens and van Loon2015). Indeed plant seeds exhibit stronger resistance against radiation compared to organs at different stages of life because of their metabolism and intrinsic characters, such as water content and structure, that influence the rate of ion penetration and production of free radicals (Kumagai et al., Reference Kumagai, Katoh, Kumada, Tanaka, Tano and Miyazaki2000; Wu and Yu, Reference Wu and Yu2001; Qin et al., Reference Qin, Wang, Xue, Miao, Ma, Mei, Zhang, Guo, Wang and Gu2007; Esnault et al., Reference Esnault, Legue and Chenal2010; de Micco et al., Reference de Micco, Arena, Pignalosa and Durante2011).
Plant cells with a specialized thick secondary wall coated with phenolic compounds (lignins and suberin) provide high resistance to ionizing radiation. The cell wall's role in radioprotection is not yet entirely proved since its structural components, like cellulose and pectin, show increased sensitivity to ionizing radiation (Kovács and Keresztes, Reference Kovács and Keresztes2002). The phenolic compounds occurring in the cuticle, trichomes and cells of sub-epidermal layers act as screens and provide a photoprotective function. Some specific phenolic compounds also act as antioxidant agents (Agati et al., Reference Agati, Stefano, Biricolti and Tattini2009). Due to an effective anti-oxidative system triggered by radiation-induced oxidative stress, plants protect themselves against higher radiation (Zaka et al., Reference Zaka, Vandecasteele and Misset2002; Arena et al., Reference Arena, de Micco, Aronne, Pugliese, Virzo De Santo and de Maio2013). Also, polyploid species show a higher resistance to radiation than species with low chromosome numbers within the same genus. Due to the increased regeneration capability, plants are more tolerant against ionizing radiation or other environmental stresses (de Micco and Aronne, Reference de Micco, Aronne and Aroca2012). Enhanced tolerance of plants to high doses of ionizing radiation is attributed to the activation of the scavenging enzymatic machinery and the enzymes regulating stress response pathways (like the nuclear enzymes poly-ADP-ribose polymerases) that recognizes and repairs damaged DNA (Arena et al., Reference Arena, de Micco, Macaeva and Quintens2014). It has also been reported that higher doses of X- and γ-rays (50 and 100 Gy) increase scavenger enzymes activity and overproduce pigments (anthocyanins, carotenoids and flavonoids) as well as ascorbic acid, glutathione and phenolic compounds that potentially remove free radicals and counteract cell oxidative damage (Arena et al., Reference Arena, de Micco, Aronne, Pugliese, Virzo De Santo and de Maio2013; Fan et al., Reference Fan, Shi, Huang, Xu, Wang and Guo2014; de Micco et al., Reference de Micco, Arena and Aronne2014a). The scavenger enzymes include SOD, catalase, ascorbate peroxidase, glutathione-S-transferase and glutathione reductase (Gill and Tuteja, Reference Gill and Tuteja2010; Foyer and Shigeoka, Reference Foyer and Shigeoka2011; Hasanuzzaman et al., Reference Hasanuzzaman, Bhuyan, Anee, Parvin, Nahar, Mahmud and Fujita2019). These enzymes work in harmony to protect plant cells from radiation-induced oxidative damage by scavenging ROS, which affects the expression of several genes and numerous processes, like growth, development, cell cycle, cell death, signalling, abiotic stress responses and pathogen defence.
A study by Kim et al. (Reference Kim, Baek, Chung, Wi and Kim2004) demonstrated that low doses of γ radiation altered photosynthesis and antioxidant capacity of red pepper (Capsicum annuum L.) grown from γ-irradiated seeds. Enzyme activity assays and pigment analyses of two cultivars showed that irradiation modified the activities of antioxidant enzymes (SOD and glutathione reductase) and the composition of photosynthetic pigments (chlorophylls and carotenoids) (Kim et al., Reference Kim, Baek, Chung, Wi and Kim2004). In a study, Marcu et al. (Reference Marcu, Cristea and Daraban2013) showed that an irradiation dose of ionizing radiation between 2 and 30 Gy enhanced the growth factors (final germination percentage, germination index and root and hypocotyl length) and the photosynthetic pigments (chlorophyll a, chlorophyll b and carotenoids) content of lettuce plants compared to untreated ones. A higher dose (70 Gy) significantly decreased plant growth as well as assimilatory pigments. Similarly, Arena et al. (Reference Arena, de Micco, Macaeva and Quintens2014) showed that 50 and 100 Gy of X-rays reduced the ratios of Chl a/b and Chl (a + b)/Car(x + c) in dwarf bean (Phaseolus vulgaris) leaflets, indicating that chlorophylls and carotenoids are sensitive to higher doses of irradiation.
Nevertheless, the effects of ionizing radiation on photosynthetic pigments vary among plant species and cultivars (Kim et al., Reference Kim, Baek, Chung, Wi and Kim2004). Changes in the plant height, leaf area and mass, stem, above-ground biomass, photosynthesis and respiration rates and chlorophyll fluorescence (f v/f m ratio and yield) have been observed in irradiated flowering plants like A. thaliana (Kurimoto et al., Reference Kurimoto, Constable and Huda2010). In a study, Arena et al. (Reference Arena, Vitale, Hay Mele, Cataletto, Turano, Simoniello and de Micco2019) determined the effect of ionizing radiation (25 Gy calcium ions) on the morphology, photosynthetic efficiency, leaf anatomical functional traits and antioxidant production in leaves and fruits of a tomato plant (Solanum lycopersicum L. ‘Microtom’). Plants germinated from irradiated seeds showed various physiological and structural changes. Better photochemical efficiency, higher amounts of D1 protein and photosynthetic pigment content, leaves with smaller cells and a lower number of chloroplasts were observed in plants germinated from irradiated seeds than controls. Also, plants grown from irradiated seeds produced larger berries, richer in antioxidants (carotenoids, anthocyanins and ascorbic acid) than controls (Arena et al., Reference Arena, Vitale, Hay Mele, Cataletto, Turano, Simoniello and de Micco2019). In an experiment, Desiderio et al. (Reference Desiderio, Salzano, Scaloni, Massa, Pimpinella, de Coste, Pioli, Nardi, Benvenuto and Villani2019) investigated the effects of X- and γ-rays on tomato hairy root cultures and found variation in the proteome of cultures exposed to 5 or 10 Gy of X- or γ-rays but no morphological changes compared to the unexposed control. These studies confirm that plants trigger various molecular, physiological, and morphological changes for the acclimation or adaptation to ionizing radiation.
Space ionizing radiation (real and simulated) has been widely used in plant breeding program to create plants with desirable traits, such as high yield, shorter growth period, flower colour, temperature tolerance, pathogen-resistance, etc. (Mei et al., Reference Mei, Qiu, Sun, Huang, Yao, Zhang, Hong and Ye1998; Cyranoski, Reference Cyranoski2001; Okamura et al., Reference Okamura, Yasuno, Ohtsuka, Tanaka, Shikazono and Hase2003; Yamaguchi et al., Reference Yamaguchi, Nagatomi, Morishita, Degi, Tanaka, Shikazono and Hase2003, Reference Yamaguchi, Shimizu, Hase, Tanaka, Shikazono, Degi and Morishita2010; Wei et al., Reference Wei, Yang, Xia, Furusawa, Guan, Xin and Sun2006; Zhou et al., Reference Zhou, Li, Yu, Li, Li, Ma, Dong, Zhou and Leloup2006b; Li et al., Reference Li, Liu, Cheng and Sun2007; Chengzhi, Reference Chengzhi2011; Wong et al., Reference Wong, Law, Yao, Chen, Xu, Liu and Leung2016; Yamaguchi, Reference Yamaguchi2018). New cultivars of several ornamental plant species (e.g. chrysanthemum, carnation, rose, Lilium, Limonium, Gypsophila, Gentiana, Tulipa, Delphinium, Dahlia, Dianthus, Tricyrtis hirta, Rhododendron, Asclepias, Hydrangea, Petunia, Cyclamen, Dendrobium, Cymbidium, Osteospermum, Salvia, Prunus, Torenia, etc.) with new flower colours and shapes, fewer lateral buds and malformed flowers and the ability to flower early at a low temperature have been produced using ion beams and sold as potted plants and cut flowers (Ueno et al., Reference Ueno, Nagayoshi, Imakiire, Koriyama, Minami, Tanaka, Hase and Matsumoto2013; Yamaguchi, Reference Yamaguchi2018). Considerable DNA alternations, point-like mutations and null mutations have been observed in mutants of chrysanthemum, carnation and Arabidopsis induced by ion beams (Tanaka et al., Reference Tanaka, Shikazono and Hase2010). Appropriate doses of ion beam radiation have also been utilized to generate novel mutants of crops, such as faba bean, rye, rice and linseed (Yamaguchi et al., Reference Yamaguchi, Hase, Tanaka, Shikazono, Degi, Shimizu and Morishita2009; Khazaei et al., Reference Khazaei, Mäkelä and Stoddard2018).
In the study of Mei et al. (Reference Mei, Qiu, Sun, Huang, Yao, Zhang, Hong and Ye1998), mutations and morphological features of maize plants germinated from seeds exposed to space radiation (Chinese satellite for 15 days) were investigated. Morphological changes, such as yellow stripes on leaves, dwarf, yellow-green seedlings, etc., were observed in first-generation plants grown from irradiated seeds. The results showed that radiation-induced mutagenic changes are inherited in successive generations (Mei et al., Reference Mei, Qiu, Sun, Huang, Yao, Zhang, Hong and Ye1998). Alteration of cell growth, various chromosome aberrations (micronuclei, chromosomal bridges, fragments and laggards) were found in spaceflight-subjected as well as artificially-irradiated (heavy energy ion of) rice seeds (Wei et al., Reference Wei, Yang, Xia, Furusawa, Guan, Xin and Sun2006). The frequency of mitotic index and chromosome aberrations was higher in space-exposed seeds than ground-irradiated ones (Wei et al., Reference Wei, Yang, Xia, Furusawa, Guan, Xin and Sun2006).
Space ionizing radiation-induced changes in secondary metabolites production have been appreciated in rhizomes of a Chinese traditional medical plant Licorice (Glycyrrhiza uralensis) grown from seeds exposed in space to a radiation dose of 0.102 mGy day−1 for 18 days (Gao et al., Reference Gao, Li, Yan, Gao and Hu2009; Dong et al., Reference Dong, Gao, Zhang, Zuo and Huang2012). In another study, an increase in glycyrrhizic acid and liquiritin was measured along with significant changes in other secondary metabolites production (e.g. flavonoids, triterpene saponins, coumarin) in the root extracts of Licorice after space exposure (Zhang et al., Reference Zhang, Gao, Gao, Liu and Huang2011). Spaceflight-induced inheritable genetic mutations in the glycyrrhizic-acid-related gene have also been noted in G. uralensis plants grown from seeds receiving a radiation dose of 0.102 mGy day−1 and suggested that space conditions can be used for accelerating the progress in plant breeding (Yan et al., Reference Yan, Gao, Lu and Zhao2009).
Taken together, the experiments conducted with ionizing radiation on Earth and in the space environment show that the ionizing radiation produces useful structural modifications as well as stable genetic alterations (wide spectrum and high rate of mutations), suggesting the application of space ionizing radiation for the development of new varieties of plant species that can thrive and produce under stress conditions.
Conclusion and future perspectives in space research
Space environmental conditions, in particular microgravity and radiation, exert significant pressure on organisms resulting in changes in genes, physiology, metabolites production and morphology that are difficult to achieve on Earth. Recent studies using advanced molecular tools and analytics expand our understanding of these changes in cells and organisms that lead to adaptation and evolution in the space environment. Due to the unique environmental factors offered by space, scientists emphasize the commercial exploration of space factors for the production of novel strains, drugs and products for the health and agricultural sectors. Several infrastructure and space operation capacities are currently available or will be available in the near future as standard carriers (e.g. Shepard spacecraft by Blue Origin, Cygnus spacecraft by Orbital ATK or Dragon spacecraft by SpaceX) to execute various biological experiments in space. Satellite missions allow long-term experiments (days to decades) in microgravity at far lower costs than experiments on the ISS (fewer safety regulations). However, all experiments in a satellite need to be controlled fully automatically, as no operator is available to perform necessary tasks. Sounding rockets, parabolic flights and drop-tower campaigns offer short microgravity periods. Nevertheless, these facilities play an integral role as testbeds for hardware development and initial experiments with organisms because of the lower costs than a satellite or an ISS mission and relatively easy accessibility.
National space agencies are currently encouraging private entrepreneurs to participate actively and conduct various space research in different fields of life and material sciences. Recently, private stakeholders and companies are supporting space research programs. For example, the biotech company Space Biology Unlimited (SBU), France (https://space-cu.com/space-biology-unlimited/), a subsidiary of Space Cargo Unlimited, Luxembourg, aims to bring life science research in space with innovative technologies. In collaboration with academia, research institutes and industries, SBU is performing six experiments of an ambitious project ‘WISE’ (Vitus Vinum in Spatium Experientia) on the ISS to obtain fruitful outcomes for the future of food, agriculture and health on Earth. Very recently, SBU sent 12 wine bottles to the ISS for 12 months of storage. The objective of this mission is to investigate how space radiation and real microgravity conditions affect the wine components during the ageing process in space since wine is a multi-component system consisting of key elements, such as yeast, bacteria, but also crystals, colloids or polyphenols (Zanchi et al., Reference Zanchi, Poulain, Konarev, Tribet and Svergun2008; Markoski et al., Reference Markoski, Garavaglia, Oliveira, Olivaes and Marcadenti2016).
As space experiments are expensive, one must narrow down the research to a limited number of model organisms. For example, grapevines (Vitis vinifera) can be studied as a representative of agricultural plants because of their substantial scientific promise and relevance in food quality, food production, nutrition and health (e.g. resveratrol and oligomeric procyanidins). Very recently, in an experiment named ‘CANES’, which is a part of the WISE project, SBU sent grapevine canes to the ISS (https://space-cu.com/space-cargo-unlimited-debuts-the-third-space-experiment-of-mission-wise-in-partnership-with-cnes-and-esa/). The aim is to investigate the genetic, epigenetic and metabolic changes in grapevine canes due to the long-term storage in real microgravity conditions and create diverse novel varieties of grapevine cuttings. Microbes, such as yeast (e.g. Saccharomyces) and bacteria (e.g. Lactobacillus spp. and Oenococcus oeni), are interesting model organisms. S. cerevisiae has been used in winemaking, baking and fermented food production since ancient times. It is one of the most intensively studied eukaryotic model organisms in molecular and cell biology. The Gram-positive bacterium O. oeni does not contain a DNA repair system and plays an important role in the fermentation process and winemaking.
Collectively, the space environment can be used as a unique stress selection factor to develop novel and rare variants of microbes and plants that will have the immense potential to occupy a niche in the modern biotechnological market and agriculture on Earth and beyond.
Acknowledgments
Space Biology Unlimited SAS, France, and Space Cargo Unlimited, Luxembourg support this work, which is highly appreciated by the authors. The authors are also grateful to the German Aerospace Center DLR (Grant #50WB1923). SMS was supported by the Coordenação de Aperfeiçoamento de Pessoal de Nível Superior – Brasil (CAPES). RLM was funded through NASA Cooperative agreement NNX12AD05A and Grant #80NSSC18K0751 with the Bay Area Environmental Research Institute.
Conflicts of interest
The authors declare no conflict of interest.