Introduction
Coronavirus disease 2019 (COVID-19) is causing worldwide morbidity and mortality. The aetiology and progression of the – initially – respiratory disease in those severely affected is complex and affects many organ systems. Disease severity varies widely between affected individuals, from an asymptomatic course or mild symptoms to an overwhelming multisystem illness with high mortality. Those who are older, obese and with major pre-existing health conditions (including cardiovascular disease and diabetes) are at greatest risk(Reference Velavan and Meyer1–Reference Triggle, Bansal and Ding5). In addition to a high incidence of acute respiratory distress syndrome (ARDS), the virus poses particular risks of both arterial and venous thromboembolism and of haemorrhagic complications: pulmonary embolism and stroke are important causes of death. Endothelial damage may also contribute to the high incidence of acute kidney injury (AKI). Immune dysfunction underlies the catastrophic ‘cytokine storm’ which can be a terminal event(Reference Velavan and Meyer1,Reference Huang, Soleimani and Herasevich4,Reference Santaolalla, Beckmann, Kibaru, Josephs, Van Hemelrijck and Irshad6) . Several therapeutic interventions, particularly those that control cytokine production, have recently been shown to reduce mortality in patients with a severe disease course(Reference Huang, Soleimani and Herasevich4,Reference Group, Horby and Lim7–Reference Casadevall, Joyner and Pirofski9) . Vaccination programmes are currently rolled out globally. Until the time that a large proportion of the world population has acquired immunity, infection prevention and reduction of disease severity is paramount, in view of the severe disease process with long rehabilitation time in many patients(Reference Quesada-Gomez, Entrenas-Castillo and Bouillon10).
Vitamin D has received increased interest since it is known to modulate immune function and the inflammatory response and vitamin D deficiency is associated with increased risk of respiratory infections(Reference Quesada-Gomez, Entrenas-Castillo and Bouillon10–15) and adverse health outcomes in severely ill patients(Reference Ney, Heyland and Amrein16,Reference Kempker, Tangpricha, Ziegler and Martin17) . It may therefore be involved in both susceptibility and progression of respiratory disease. No good-quality, population representative data on the relationship between vitamin D status and severe acute respiratory syndrome coronavirus 2 (SARS-CoV-2) infection risk and severity of COVID-19 exist. The first findings from three intervention studies in hospitalised COVID-19 patients supplemented with vitamin D were recently published(Reference Entrenas Castillo, Entrenas Costa and Vaquero Barrios18–Reference Nogues, Ovejero and Pineda-Moncusi20), and several randomised controlled trials (RCT) in diverse population or patient groups are ongoing(Reference Quesada-Gomez, Entrenas-Castillo and Bouillon10,Reference Chakhtoura, Napoli and El Hajj Fuleihan21,Reference Raisi-Estabragh, Martineau and Curtis22) .
Vitamin D status is measured as the plasma concentration of 25-hydroxy vitamin D (25(OH)D). Deficiency thresholds for population health are defined as 25(OH)D serum concentrations <25 or 30 nmol/L per UK and US guidelines. Thresholds or target values for 25(OH)D may be set higher for clinical management and specific patient groups(23–Reference Christodoulou, Aspray and Schoenmakers25). Vitamin D status has been shown to be associated with many health outcomes, but for many chronic diseases other than musculoskeletal health, intervention trials are less consistent and often do not confirm findings of observational studies(Reference Rejnmark, Bislev and Cashman26–Reference Akutsu, Kitamura, Himeiwa, Kitada, Akasu and Urashima28). This can potentially be ascribed partly to the confounding effects of vitamin D supply from sources other than the intervention given in RCTs (i.e. vitamin D supply through cutaneous sun exposure and from food and supplements), vitamin D uptake in and potential mobilisation from bodily stores and the complex nature of vitamin D metabolism. Vitamin D status is therefore influenced by factors associated with lifestyle and fitness. In addition, status is affected by pathological changes occurring during acute and chronic illness. Therefore, causality and reverse causality are difficult to distinguish. Further, the effect of interventions may depend on baseline and post-supplementation vitamin D status, but also results considering 25(OH)D before and after supplementation are conflicting(Reference Dawson-Hughes, Staten and Knowler29–Reference Chandler, Chen and Ajala32).
The role of vitamin D and the effect of supplementation has also been investigated in studies and intervention trials with respiratory disease outcomes and critically ill patients. These are likely relevant for the current COVID-19 epidemic.
Here we summarise recent findings regarding vitamin D status and metabolism in patients, with a particular focus on those severely ill and with COVID-19. The effect of vitamin D supplementation on respiratory disease and critically ill patients is described in the context of alterations of vitamin D metabolism and requirements with severe illness. We also provide an overview of guidelines to correct and maintain vitamin D status for specific categories of patients and the prevention of vitamin D deficiency in population groups.
COVID-19, vitamin D status and supplementation
The recent COVID-19 epidemic identified a high prevalence of vitamin D deficiency amongst patients(Reference Bilezikian, Bikle and Hewison33), particularly those that develop severe symptoms of the disease(Reference D’Avolio, Avataneo and Manca34–Reference Meltzer, Best, Zhang, Vokes, Arora and Solway39). Vitamin D deficiency (25(OH)D <30 nmol/L) at hospital admission (n = 185) was associated with increased hazard ratio to require invasive mechanical ventilation and death in one study(Reference Radujkovic, Hippchen, Tiwari-Heckler, Dreher, Boxberger and Merle40), but this was not found in another, slightly smaller study (n = 109)(Reference Pizzini, Aichner and Sahanic41). Patients at increased risk of requiring intensive care unit (ICU) admission and ventilation appear to be carriers of common risk factors for vitamin D deficiency, that is, being overweight or obese, having type 2 diabetes, hypertension and chronic kidney disease(Reference Docherty, Harrison and Green2,Reference Williamson, Walker and Bhaskaran3,Reference Pereira, Dantas Damascena, Galvão Azevedo, de Almeida Oliveira and da Mota Santana36,Reference Harrison42) , but vitamin D status has also been reported to be an independent predictor after adjustment for confounding factors(Reference Ye, Tang and Liao37,Reference Radujkovic, Hippchen, Tiwari-Heckler, Dreher, Boxberger and Merle40) . This raises the question whether vitamin D deficiency is a risk factor for this viral infection and/or developing severe disease symptoms is a biomarker of poorer health or that the disease process is associated with a decrease in vitamin D status as outlined below. In several countries (the United States and UK), overrepresentation of ethnic minorities testing positive and/or developing severe symptoms is reported(Reference Williamson, Walker and Bhaskaran3,Reference Meltzer, Best, Zhang, Vokes, Arora and Solway39,Reference Pan, Sze and Minhas43–Reference Kaufman, Niles, Kroll, Bi and Holick46) (https://digital.nhs.uk/coronavirus/coronavirus-data-services-updates/ethnicity-and-outcomes-of-covid-19-patients-in-england). The higher prevalence of severe illness in specific ethnic minority groups in the United States and UK, countries with temperate climates, has been suggested to be related to their generally lower vitamin D status, due to lower rate of cutaneous vitamin D production associated with a darker skin tone. Many other factors may also play a role, including higher risk of obesity, hypertension, type 2 diabetes mellitus, elevated inflammation markers and renal impairment, in these groups(Reference Chaturvedi47). Individuals of specific racial descent may potentially also have different responses to infection and treatment and may require different treatment strategies. In addition, overrepresentation of these groups in professions with high exposure risk, differences in access to and use of health care systems and other socio-economic factors may play a key role in some areas(Reference Chastain, Osae, Henao-Martínez, Franco-Paredes, Chastain and Young45,Reference Reitsma, Claypool and Vargo48) . Country differences in the prevalence of COVID-19 between ethnic groups may also reflect differences in population diversity and characteristics and health care systems. There are no prospective data available that directly allow the investigation of the protective role of vitamin D status, intake and supplementation in the prevention of infection with SARS-CoV-2 at the time of viral exposure and subsequent development of COVID-19 symptoms. Two studies with retrospective data from the population-based UK BIOBANK showed that plasma 25(OH)D measured 10–14 years before the COVID-19 outbreak was not associated with the risk of developing moderate-to-severe symptoms of the disease after correction for appropriate confounders(Reference Raisi-Estabragh, McCracken and Bethell44,Reference Hastie, Mackay and Ho49,Reference Hastie, Pell and Sattar50) . In univariate models, Hastie(Reference Hastie, Pell and Sattar50), but not Raisi- Estabragh found an increased odds ratio of COVID positivity with lower plasma concentrations of 25(OH)D, but this did not remain significant after correction for range of confounders (BMI, age, ethnicity and season) and co-morbidities. Other retrospective studies used large data sets from clinical laboratories and medical records. Kaufman et al.(Reference Kaufman, Niles, Kroll, Bi and Holick46) reported a significant negative association between the percentage of patients testing positive for SARS-CoV-2 and season-corrected plasma 25(OH)D measured within a year prior to SARS-CoV-2 infection. In subgroup analyses, Kaufman presented data by ethnic group (as based on predominance of ethnicity by postcode area), showing (a) a higher COVID positive incidence rate and (b) a lower plasma 25(OH)D concentration in postcodes with predominantly Black and Hispanic communities compared with predominantly white communities, but (c) the association with 25(OH)D was negative and significant in all three groups. Meltzer conducted two retrospective studies using large data sets from clinical laboratories and medical records. They included individuals who had a COVID-19 PCR test following potential COVID-19 symptoms or exposure and if assessed for vitamin D status within 1 year before the COVID test. In the 2020 study by Meltzer(Reference Meltzer, Best, Zhang, Vokes, Arora and Solway51), this was based on plasma 25(OH)D or 1,25(OH)2D concentrations. In the larger (n = 4638), 2021 study, this was based on 25(OH)D only(Reference Meltzer, Best, Zhang, Vokes, Arora and Solway39,Reference Meltzer, Best, Zhang, Vokes, Arora and Solway51) . An increased risk of testing positive with lower category of vitamin D status (values below versus above 50 nmol/L(Reference Meltzer, Best, Zhang, Vokes, Arora and Solway51) and below 50 nmol/L versus ≥100 nmol/L(Reference Meltzer, Best, Zhang, Vokes, Arora and Solway39)) was reported in both studies. In subgroup analyses by ethnicity and controlling for a range of confounders and co-morbidities as well as time since last 25(OH)D test, Meltzer(Reference Meltzer, Best, Zhang, Vokes, Arora and Solway39) reported an increased risk of infection (incidence rate ratio (IRR) 2·55; 95% CI, 1·26–5·15) in African Americans with a 25(OH)D below 50 nmol compared with those with a concentration over 100 nmol/L (<20 versus >40 ng/mL). The relationship between the COVID-19 IRR and 25(OH)D was non-linear and non-significant for the category 50–75 nmol/L compared with ≥100 nmol/L. This association with 25(OH)D was not found in white counterparts. The results from Kaufman and Meltzer suggest that there may be an interaction between ethnicity, vitamin D status and COVID-19 risk. However, differences between ethnic groups in the relative risk of infection and association with vitamin D status may also be determined by other factors that differ by ethnicity as outlined above. Such associations between COVID infection risk and vitamin D status may be lost in those reports that applied statistical adjustment for ethnicity(Reference Hastie, Pell and Sattar50), since in temperate climates, vitamin D status differs by ethnic group. Another study (Israel; subtropical region) reported an increased odds ratio for infection and hospitalisation with COVID-19 amongst patients who had a plasma 25(OH)D below 75 nmol/L tested before the COVID-19 outbreak(Reference Merzon, Tworowski and Gorohovski52). In this study, it was unclear how long before the outbreak and in which season this prior test of 25(OH)D was done. Both Kaufman and Merzon(Reference Kaufman, Niles, Kroll, Bi and Holick46,Reference Merzon, Tworowski and Gorohovski52) did not correct for body composition nor provide data whether treatment of vitamin D deficiency was initiated after testing, a factor considered in the two studies by Meltzer(Reference Meltzer, Best, Zhang, Vokes, Arora and Solway39,Reference Meltzer, Best, Zhang, Vokes, Arora and Solway51) .
There are several limitations to these prospective population-based studies and the use of medical records. Changes in recommendations for vitamin D intake and testing for and correction of deficiency may have resulted in secular trends in population vitamin D status. In the UK, however, population vitamin D status did not substantially change after the 2016 revised guidance (https://www.gov.uk/government/collections/national-diet-and-nutrition-survey). Although vitamin D status is known to track within a person, it is strongly influenced by season. In temperate climates, like the UK, population vitamin D status typically cycles with the seasons, with a nadir in March and a peak in September(Reference Schoenmakers, Gousias, Jones and Prentice53). The nadir thus coincides with the peak of many respiratory viral infections, but also other factors play a role, including meteorological factors (temperature, UV index, humidity) influencing virus survival and time spent indoors(Reference Li, Wang and Nair54,Reference Ianevski, Zusinaite and Shtaida55) . It may therefore be reasonable to assume that vitamin D status at the time of viral exposure may be the more relevant measure than a year average or season-adjusted value. Another study, relating the severity of the COVID outbreak with latitude and season also suggested a role of vitamin D status(Reference Rhodes, Subramanian, Laird, Griffin and Kenny56,Reference Rhodes, Subramanian, Laird and Kenny57) . However, the subsequent spread of the virus across all climate zones and the resurge of COVID prevalence in September 2020 and summer 2021 at northern temperate latitudes does not appear to strongly support this hypothesis. Data from population studies, such as the Biobank studies(Reference Raisi-Estabragh, McCracken and Bethell44,Reference Hastie, Mackay and Ho49,Reference Hastie, Pell and Sattar50) , are further limited by the fact that those typically at increased risk of being exposed or developing severe COVID-19 symptoms (i.e. ethnic minority groups and older frail people) are often underrepresented in population surveys(Reference Chastain, Osae, Henao-Martínez, Franco-Paredes, Chastain and Young45,Reference Reitsma, Claypool and Vargo48) , while in data sets based on medical records, individuals with underlying health conditions may be overrepresented. In addition, in clinical data sets that are restricted to patients with a prior biochemical test of 25(OH)D, individuals with an increased risk of deficiency and receiving treatment are expected to be overrepresented. Changes in vitamin D status following testing and treatment also need to be considered. Notwithstanding, generating ‘optimal’ data to assess the protective role of vitamin D status would require near-impossible intense surveillance and sampling of large cohorts of people potentially acquiring the infection.
Findings of the first small, open-label vitamin D intervention trial (n = 50 intervention; n = 26 control group) with hospitalised COVID-19 patients in Spain (diagnosed by positive PCR test and radiographic evidence of viral pneumonia) was recently published(Reference Entrenas Castillo, Entrenas Costa and Vaquero Barrios18). In this study, which was a sub-study COVIDIOL cohort, an intermediate high oral dose of calcifediol (the pharmaceutical form of 25(OH)D) at hospitalisation and at regular intervals thereafter was provided. The control group received standard medical care without vitamin D. In the vitamin D group, the risk of ICU admission was significantly reduced (OR 0·02; 95% CI 0·002–0·17), but this was based on one patient in the intervention group and thirteen in the control group requiring ICU treatment. In this study, a range of prognostic indices and risk factors for the development of severe symptoms were investigated. Compared with the intervention group, there was a higher prevalence of potential risk factors for the development of severe COVID in the control group (hypertension: 24 % versus 58 %, P = 0·002; diabetes mellitus 6 % versus 19 %; P = 0·08). After correction for these potential confounding factors, differences in the OR remained significant (OR 0·03; 95% CI 0·003–0·25). Baseline and post-supplementation vitamin D status was not assessed, and potential confounding by body composition was not considered. Differences in deaths were also investigated and were not significant. However, interpretation of this outcome was hindered by the design the study, by which supplementation was stopped on admission to ICU. Nogues(Reference Nogues, Ovejero and Pineda-Moncusi20) reported a second sub-study from the COVIDIOL cohort including a larger data set (n = 447 intervention; n = 391 control group). Interventions were identical, but in this sub-study, supplementation was continued after ICU admission. Baseline 25(OH)D was 32·5 (IQR 20–55) nmol/L. Co-morbidities were well balanced, except for a slightly lower baseline 25(OH)D concentration in the control group. This study also showed a reduced risk of ICU admission with and without adjustments for confounders, which included baseline 25(OH)D (confounder adjusted OR 0·13; 95% CI 0·07–0·23). Mortality was significantly reduced in the calcifediol-supplemented group (confounder-adjusted OR 0·21; 95% CI 0·10–0·43). A randomised placebo-controlled vitamin D intervention trial in Brazil (n = 120 intervention; n = 120 placebo) with hospitalised COVID-19 patients (diagnosed by positive PCR test) administered a single dose of 200,000 IU D3 1·4 d after admission and 10·3 d after onset of symptoms. Mean baseline 25(OH)D was 52 ± 9·1 nmol/L. This trial did not result in a reduction in length of hospitalisation, in-hospital mortality, risk of ICU admission or requiring mechanical ventilation(Reference Murai, Fernandes and Sales19). Rastogi(Reference Rastogi, Bhansali and Khare58) investigated the 21-d SARS-CoV-2 clearance rate in mildly symptomatic or asymptomatic individuals with a 25(OH)D < 50 nmol/L (median baseline concentration <25 nmol/L). Supplementation was open label and individually tailored to achieve a 25(OH) concentration over 50 nmol/L. They reported a higher proportion of patients who were SARS-CoV-2 negative after 21 d in the supplemented group (n = 10 of 16; 62·5 %) versus the placebo group (n = 5 of 24; 20·8 %), although the mean duration to being SARS-CoV-2 negative was not different between groups. No data were presented on the severity of symptoms or length of hospitalisation, and no data on risk factors.
Time from onset of COID symptoms was only reported in the study by Murai (10·3 ± 4·3 d); Nogues(Reference Nogues, Ovejero and Pineda-Moncusi20), Entrenas Castillo(Reference Entrenas Castillo, Entrenas Costa and Vaquero Barrios18) and Murai(Reference Murai, Fernandes and Sales19) reported time from hospitalisation to supplementation (0, 0 and 1·4 (0·9 d) d, respectively). Considering the potential multifactorial role of vitamin D (and thus status and supplementation) in different stages and aspects of the response to viral infection and subsequent development of symptoms, time since infection and development of (severe) symptoms relative to the start of supplementation as well as baseline 25(OH)D concentrations may be important factors to consider in the evaluation of the effect of interventions. This is supported by secondary outcomes reported by Nogues(Reference Nogues, Ovejero and Pineda-Moncusi20). They found that, when mortality rates were considered including both patients that initiated calcifediol treatment at hospitalisation (n = 447) and those that first received this at ICU admission (n = 53), the adjusted mortality OR (0·52; 95% CI 0·27–0·99) was higher than when only patients were considered that initiated treatment at admission. The authors speculated that supplementation is more effective if initiated before the development of ARDS.
Though not randomised, an audit of standard care in a French nursing home found that residents who had received regular 2–3 monthly vitamin D supplementation (oral 80,000 IU as a bolus) within 1 month before or 1 week after SARS-CoV-2 virus infection had a less severe disease course and lower mortality(Reference Annweiler, Hanotte, Grandin de l’Eprevier, Sabatier, Lafaie and Celarier59). Similarly, an audit of vitamin D supplementation in frail hospitalised COVID-19 patients, showed a lower mortality risk in those that who had received regular vitamin D supplementation preceding infection and in those supplemented directly after testing positive (both 80,000–100,000 IU as a bolus) compared with those who did not receive vitamin D supplementation(Reference Annweiler, Corvaisier and Gautier60).
Upper respiratory tract infections and vitamin D status and supplementation
Observational studies have shown that vitamin D deficiency may predispose to increased risk of viral acute respiratory tract infections. Findings of the effect of vitamin D supplementation on the risk of preventing acute respiratory tract infections are conflicting. Meta-analyses of RCTs and recent RCTs indicate that daily supplementation (intakes of 400–1000 IU/d) has a small protective effect(Reference Quesada-Gomez, Entrenas-Castillo and Bouillon10,Reference Martineau, Jolliffe and Hooper11,Reference Vuichard Gysin, Dao, Gysin, Lytvyn and Loeb14,15,Reference Jolliffe, Camargo and Sluyter61) . No protective effect was found with less frequent (weekly or less or after a bolus) administration. Many of the studies were conducted in populations with pre-existing respiratory diseases and in children. The most recent and largest meta-analyses found no interaction with 25(OH)D concentration at baseline(Reference Jolliffe, Camargo and Sluyter61), whereas in an earlier individual participant data meta-analyses, a stronger protective effect was found in subgroups with baseline vitamin D deficiency (<25 nmol/L)(Reference Martineau, Jolliffe and Hooper11,Reference Martineau, Jolliffe and Greenberg62) . The two UK Scientific Advisory Committee on Nutrition (SACN) rapid reviews on vitamin D and acute respiratory tract infections concluded that there is evidence of a small beneficial effect of vitamin D supplementation with intakes of 400–1000 IU/d, but that sufficient evidence is only available for children 1–16 years of age (https://www.gov.uk/government/publications/sacn-rapid-review-vitamin-d-and-acute-respiratory-tract-infections). A recent study in long-term care residents, with approximately one-third of participants with a baseline 25(OH)D < 50 nmol/L, showed a significant protective effect (odds ratio 0·67) with high-dose monthly supplementation (100,000 IU per month). However, in this study, consistent with other reports with high-dose bolus vitamin D supplementation, an increased risk of falling was observed(Reference Ginde, Blatchford and Breese13).
Evidence from a large multi-centre observational study in infants hospitalised for bronchiolitis suggests that vitamin D status is associated with severity of respiratory disease. This study showed that the percentage of hospitalised children that proceeded to need intensive care treatment was almost twice as high in those with a 25(OH)D < 50 nmol/L compared with those with a concentration >75 nmol/L (22 % versus 12 %)(Reference Vo, Koppel and Espinola63). Vitamin D intervention studies in children with pneumonia have, however, not shown a reduction of length of recovery and other disease outcomes(Reference Vo, Koppel and Espinola63).
Mechanistic studies support a role of vitamin D and its metabolites and vitamin D binding protein (DBP) in immune function and pulmonary health, which is summarised below. The contrasting findings between observational, mechanistic and intervention studies suggest that many other factors play a role; the beneficial effects of vitamin D may be limited to specific subgroups, the alterations in vitamin D metabolism with disease processes may influence its function and/or other drivers of the immune and inflammatory response may be predominant, particularly in severe illness.
Summary of interactions of vitamin D metabolites and vitamin D binding protein with respiratory tract infections and immune function
The effect of vitamin D and DBP on immune function and pulmonary health has been described in in vitro, ex vivo and animal models and in human health(Reference Hewison64). It is considered to be multi-factorial and involves both the innate and adaptive immune function (see reviews(Reference Quesada-Gomez, Entrenas-Castillo and Bouillon10,Reference Hewison64,Reference Bilezikian, Bikle and Hewison65) ). Laboratory studies indicate that the active metabolite 1,25-dihydroxy vitamin D (1,25(OH)2D) modulates the expression of angiotensin-converting enzyme 2 (ACE2), the receptor for SARS-CoV, including SARS-CoV-2, and may thus modulate viral entry and infection(Reference Quesada-Gomez, Entrenas-Castillo and Bouillon10,Reference Rhodes, Subramanian, Laird, Griffin and Kenny56) . To date, it is unclear what the importance of this mechanism is. Vitamin D metabolites and DBP appear to play an important role in the immune and inflammatory response and, hence, disease severity and the development of immunity. It may also be hypothesised that vitamin D status modulates the response to vaccination. Many of the cells of the immune system and those with a barrier function, express vitamin D receptors (VDRs) and CYP27B1, the enzyme that hydroxylates 25(OH)D into 1,25(OH)2D. This locally produced 1,25(OH)2D activates VDRs for autocrine and paracrine functions. It stimulates the expression of the antimicrobial function of cathelicidin, which modulates the chemokine and cytokine response and stimulates the chemotaxis of neutrophils, monocytes and T cells, thus promoting the clearance of respiratory pathogens(Reference Quesada-Gomez, Entrenas-Castillo and Bouillon10,Reference Hewison64–Reference Kumar, Rathi, Haq, Wimalawansa and Sharma66) . Further, 25(OH)D and 1,25(OH)2D stimulate autophagic encapsulation of viral particles, promoting lysosomal degradation and antigen presentation(Reference Bilezikian, Bikle and Hewison65). The role of vitamin D deficiency and supplementation in lymphocyte differentiation and apoptosis remains unclear(Reference Zheng, Pan, Li, Xiang and Jin67,Reference de Carvalho, Schneider, Cuppari, Grabulosa, Cendoroglo and Dalboni68) , but considering that severe illness with COVID-19 is characterised by inadequate T-cell responses(Reference Velavan and Meyer1,Reference Sette and Crotty69) , it may be expected that the effects of vitamin D metabolites on immune function with severe COVID are altered. It may therefore be speculated that vitamin D status is of particular importance in earlier stages and the progression of the disease, preventing the development of a cytokine storm, found in patients with a severe disease course of COVID-19(Reference Quesada-Gomez, Entrenas-Castillo and Bouillon10,Reference Hewison64) . Vitamin D deficiency has also have been linked to increased risk of the development of endothelial dysfunction and cardiovascular complications, frequently observed in patients hospitalised with COVID-19. Although no direct casual evidence is available, mechanistic studies suggest that deficiency or reduced VDR activation leads to overexpression of the renin– angiotensin aldosterone system (RAAS), hypertension and increased thrombogenicity(Reference Bilezikian, Bikle and Hewison65). This may influence the effects SARS-CoV-2 infection and its interaction with the ACE2 receptor, suggested to lead to dysregulation of RAAS(Reference Bilezikian, Bikle and Hewison65,Reference Garvin, Alvarez and Miller70) .
Summary of vitamin D metabolism
Vitamin D metabolism
Vitamin D is synthesised in the skin after sunshine exposure and taken up into the lymph system. In temperate climates this only occurs during spring and summer months, whereas at latitudes <30° north and south this can take place throughout the year. Oral vitamin D is rapidly and efficiently absorbed from the intestine incorporated in chylomicrons. Vitamin D from both supply routes is transported to the liver, but part of it may be taken up by fat tissue. In the liver, vitamin D is converted to 25(OH)D (Fig. 1). Despite the efficient conversion (˜80 % at first passage with normal liver function), it takes several days to weeks to correct vitamin D deficiency unless high (bolus) dosages are given, particularly in severely deficient patients with a high BMI. This is thought to be due to the uptake of vitamin D by fat cells. Oral supplementation with 25(OH)D (i.e. calcifediol), at present only available on prescription, leads to a faster (with an increase already detectable within a few hours after administration) and proportionally higher increase in plasma 25(OH)D(Reference Schoenmakers, Jones, Feldman, Pike, Goltzman, Giovannucc, Hewison and Bouillon71).
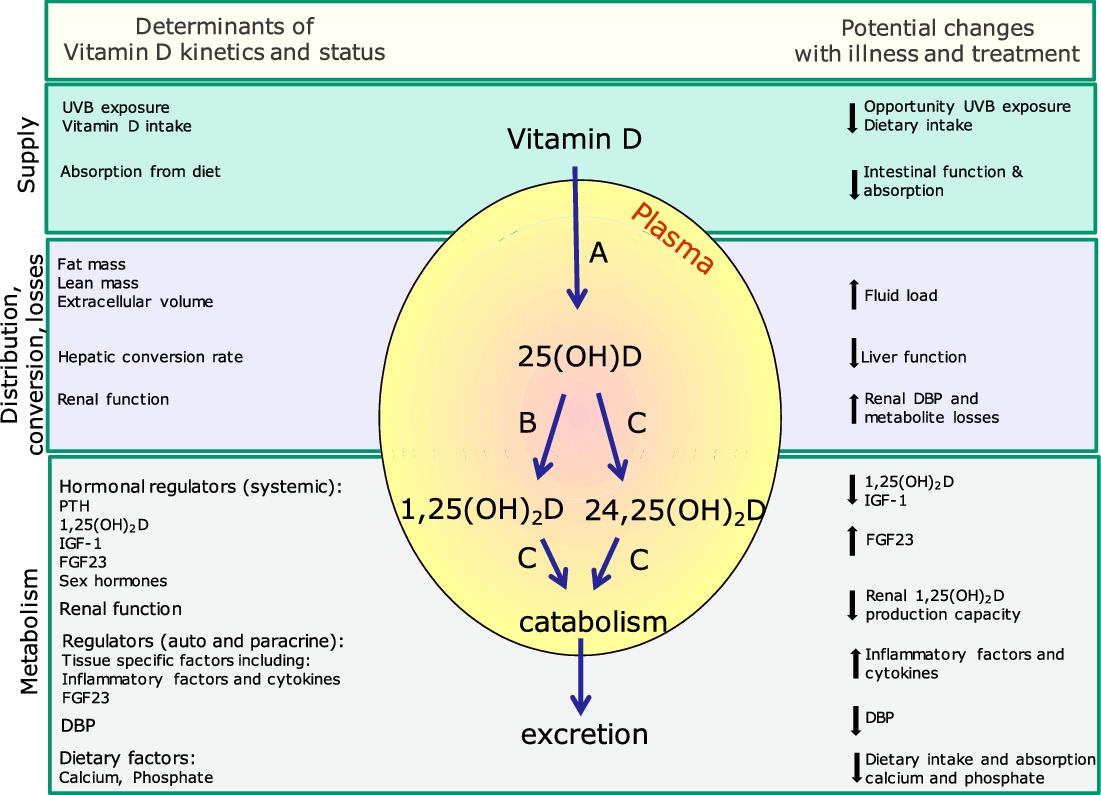
Fig. 1. Determinants of the kinetics of vitamin D and potential changes with severe illness and treatment. Blue arrows indicate metabolic pathways of vitamin D and vitamin D metabolites. Letters indicate hydroxylation enzymes: A, CYP2R1; B, CYP27B1; C, CYP24A1. Changes associated with severe illness lead to pronounced changes in supply, distribution, hepatic conversion, renal losses and metabolism due to changes in hydroxylation enzyme activity. Determinants of these changes are summarised. Conversion (A) of vitamin D into 25(OH)D may be impaired, activation (B) of 25(OH)D into 1,25(OH)2D may be decreased, and catabolism (C) of 25(OH) D into 24,25(OH)2D and 1,25(OH)2D into1,24,25(OH)3D and further downstream products may be increased. Metabolism may further be influenced by medication use. For further explanation, see text. Vitamin D metabolism is further influenced by physiological factors and life stage, including growth, pregnancy and ageing
A further conversion into the active metabolite 1,25(OH)2D is required, and this occurs in the kidney for systemic effects. Local conversion takes place in many other tissues for autocrine and paracrine effects, including the lung and cells of the immune system. The regulation of 1,25(OH)2D production in the kidney is predominantly under control of parathyroid hormone (PTH), 1,25(OH)2D itself, fibroblast growth factor 23 (FGF23) and several other factors, including sex hormones (reviewed in Ref.(Reference Schoenmakers, Jones, Feldman, Pike, Goltzman, Giovannucc, Hewison and Bouillon71)). The extra-renal production is not under the control of these factors, except for FGF23. Instead, its regulation is thought to be tissue specific and is only partly understood. In immune cells, the regulation of 1,25(OH)2D production is partly influenced by inflammatory factors and cytokines (reviewed in Refs.(Reference Quesada-Gomez, Entrenas-Castillo and Bouillon10,Reference Hewison64,Reference Kew72) ) (Fig. 1).
Generally, there is no or a poor correlation between plasma concentrations of 25(OH)D and 1,25(OH)2D. The concentration below which 25(OH)D becomes rate limiting for 1,25(OH)2D production is not well established and also depends on other factors. It is generally assumed that when 25(OH)D falls below 15 nmol/L, plasma 1,25(OH)2D is reduced(Reference Schoenmakers, Jones, Feldman, Pike, Goltzman, Giovannucc, Hewison and Bouillon71). A meta-analysis of supplementation studies, however, showed that post-supplementation 1,25(OH)2D concentrations also increased when baseline 25(OH)D concentrations were above 15–20 nmol/L(Reference Zittermann, Ernst, Birschmann and Dittrich73). It is unclear whether at lower 25(OH)D concentrations the balance between renal and extra-renal 25(OH)D availability and tissue uptake is altered. In the kidney, internalisation of 25(OH)D, bound to its binding protein is through an active megalin–cubilin receptor mediated mechanism. It may therefore be assumed that renal uptake takes place against the concentration gradient, partly overcoming the effects of low plasma 25(OH)D availability. In most other tissues, including those pertaining to the immune system, a receptor-mediated internalisation mechanism has not been demonstrated. According to the free hormone theory, cellular uptake in these extra-renal tissues is thought to be concentration dependent and thus is reduced when 25(OH)D is low (see further below).
The role of vitamin D binding protein in vitamin D metabolism
The majority of vitamin D and its metabolites circulate in plasma bound to DBP (˜85–90 %) and to a lesser extent and with a lower binding affinity to albumin. Less than 1 % circulates in its free form(Reference Schoenmakers, Jones, Feldman, Pike, Goltzman, Giovannucc, Hewison and Bouillon71). In healthy people, the free 25(OH)D concentration is highly correlated to total 25(OH)D and accordingly, the free: total 25(OH)D ratio is stable throughout the physiological ranges of 25(OH)D(Reference Schwartz, Gallagher and Jorde74). In some physiological and pathological conditions, the free 25(OH)D fraction is altered, and this is thought to be predominantly a result of changes in the concentrations of DBP(Reference Schwartz, Gallagher and Jorde74). DBP binding of vitamin D metabolites has several functions: to prolong half-life and to facilitate cellular internalisation of vitamin D metabolites through the megalin–cubilin receptor pathway. The megalin–cubilin receptor is expressed in a number of other tissues and is not specific to the DBP–vitamin D complex. Internalisation of vitamin D metabolites through this pathway has to date only been shown in renal and muscle tissue and potentially in the mammary gland. Other tissues, including those pertaining to the immune system are thought to be mostly dependent on the internalisation of the free fraction. However, other internalisation mechanisms for 25(OH)D and 1,25(OH)2D have been proposed, and DBP also plays an important role in the delivery and distribution of compounds to all tissues(Reference Chun, Peercy, Orwoll, Nielson, Adams and Hewison75).
The megalin–cubilin mediated internalisation of the DBP-25(OH)D complex is thought to be the predominant internalisation route in the kidney and is also essential for renal reabsorption of the DBP complex and thus prevents urinary losses of both the protein and the associated vitamin D metabolites. A decrease in the expression of these receptors with renal impairment is associated with a decline in systemic 1,25(OH)2D concentrations. It may be speculated that also a reduction of plasma DBP may lead to a reduction in renal bioavailability of vitamin D metabolites, reducing the substrate for production of systemic 1,25(OH)2D.
DBP binding protects against catabolism of vitamin D metabolites. As a consequence, in conditions with a decreased DBP concentration, although this has never been measured directly, it is expected that the bodily stores of 25(OH)D are more rapidly depleted and that the half-life of 25(OH)D and the active metabolite 1,25(OH)2D decreases. This potentially explains the decrease in vitamin D status during acute illness and the frequently observed poor vitamin D status in ICU patients, even in those who do not have the traditional risk factors and may have adequate vitamin D intake. These mechanisms may also partly explain the low and decline in 1,25(OH)2D concentrations observed in many ICU patients as described in the next sections.
DBP further fulfils several other functions in the immune system and in response to inflammation and tissue damage as summarised below(Reference Schoenmakers, Jones, Feldman, Pike, Goltzman, Giovannucc, Hewison and Bouillon71,Reference Chun, Peercy, Orwoll, Nielson, Adams and Hewison75,Reference Bouillon, Schuit, Antonio and Rastinejad76) .
Regulation of the concentration and production of vitamin D binding protein
Vitamin D binding protein is mainly produced in the liver, although the gene and protein are also expressed in very low concentrations in other tissues, including monocytes(Reference Chishimba, Thickett, Stockley and Wood77). It has three domains. The ‘A’ domain binds vitamin D metabolites and the B and C domains actin, and this can occur simultaneously(Reference Bouillon, Schuit, Antonio and Rastinejad76). The estimated daily production of DBP is about 700–900 mg/d for an adult (10 mg/kg/d). The plasma concentration of DBP in healthy people is in the micromolar range (˜6 μmol/L or 300 mg/L) and is remarkably stable throughout the life course. After acute depletion, for example after trauma or plasma exchange, DBP returns to baseline values after several days(Reference Hiemstra, Casian, Boraks, Jayne and Schoenmakers78). Reported mean plasma concentrations in healthy populations vary between 200 and 600 mg/L. These differences are probably mostly related to assay differences and lack of standardisation. Some (monoclonal) assays report lower values for specific population groups due to their incomplete quantitation of specific genotypes of DBP(Reference Nielson, Jones and Bouillon79).
Similar to albumin, 40 % of DBP is intravascular and 60 % is distributed in the interstitial space of various organs (primarily muscle, adipose tissue, connective tissue and skin). It is also present in bronchoalveolar, amniotic, cerebral and seminal fluid, breast milk and saliva and bound to the cell surface of many cell types, including neutrophils(Reference Bouillon, Schuit, Antonio and Rastinejad76,Reference Chishimba, Thickett, Stockley and Wood77,Reference Gomme and Bertolini80) .
In humans, exposure to oestrogens increases serum DBP, but androgens have no effects. Vitamin D status (including deficiency and excess) and supplementation have no effect on serum DBP concentration. In vitro models showed that dexamethasone, interleukine-6 (IL-6) and tumour necrosis factor alpha (TNFα) stimulate, while transforming growth factor beta (TGFβ) inhibits its production(Reference Guha, Osawa, Werner, Galbraith and Paddock81). Accordingly, DBP hepatic production was reported to increase in trauma patients(Reference Dahl, Schiødt, Rudolph, Ott, Kiaer and Heslet82). Although acute inflammation decreases plasma DBP concentrations, in states of chronic low-grade inflammation such as in obesity and rheumatoid arthritis, comparable or slightly higher DBP concentrations are reported, suggesting that increased hepatic production compensates for the potential higher degradation rate of DBP.
Decreased serum DBP concentrations are found in patients with liver cirrhosis, with protein-energy restriction and malnutrition, peritoneal dialysis, nephrotic syndrome and a variety of other kidney diseases with proteinuria and renal loss of DBP(Reference Schwartz, Gallagher and Jorde74,Reference Bouillon, Schuit, Antonio and Rastinejad76,Reference Speeckaert, Speeckaert, van Geel and Delanghe83,Reference Leaf, Waikar, Wolf, Cremers, Bhan and Stern84) . Plasma DBP is also decreased in conditions associated with cell lysis and the release of intracellular actin into the bloodstream such as during infection or trauma(Reference Silva and Furlanetto85–Reference Waldron, Ashby and Cornes87). This decline in DBP reflects its role as actin scavenger protein(Reference Bouillon, Schuit, Antonio and Rastinejad76,Reference Speeckaert, Speeckaert, van Geel and Delanghe83,Reference Meier, Gressner, Lammert and Gressner88) .
The role of vitamin D binding protein and vitamin D in actin scavenging and coagulation
Actin is the most abundant intracellular protein in any organism. During tissue injury, actin is released into extracellular fluids. Intra-cellular actin exists in two states: monomeric globular actin (G-actin) or G-actin polymerised into filaments (F-actin)(Reference Kew72). The process of polymerisation and depolymerisation is dynamic and highly regulated by actin binding proteins. When released into the extracellular space, actin escapes normal intracellular regulatory mechanisms and the protein will form F-actin filaments. In animal models, extracellular F-actin filaments have been shown to alter the coagulation and fibrinolytic systems, leading to occlusion and damage of the microcirculation, particularly in the lung(Reference Kew72). Actin scavenging by DBP, together with gelsolin, plays an important role in the clearance of actin from the circulation and the prevention of formation of actin filaments. In addition, DBP, particularly when bound to actin, has a regulatory function in neutrophil chemotaxis and, after conversion to macrophage activating factor, in macrophage activation(Reference Quesada-Gomez, Entrenas-Castillo and Bouillon10,Reference Kew72) . The function of DBP as a neutrophil chemotactic cofactor is modulated by 1,25(OH)2D-DBP binding(Reference Kew72). Much of the mechanisms are still poorly understood(Reference Quesada-Gomez, Entrenas-Castillo and Bouillon10,Reference Kew72,Reference Chishimba, Thickett, Stockley and Wood77,Reference Hewison89) .
Upon DBP binding of actin, the complex is rapidly catabolised, decreasing DBP plasma half-life from 1·7–3 d to 30 min(Reference Bouillon, Schuit, Antonio and Rastinejad76,Reference Speeckaert, Speeckaert, van Geel and Delanghe83,Reference Meier, Gressner, Lammert and Gressner88) . Bound vitamin D metabolites are released, and although they may rapidly re-associate with another DBP molecule, the free fraction of vitamin D metabolites increases. This may be a beneficial response to infection, increasing the availability of vitamin D metabolites for passive cellular internalisation, which may particularly increase the uptake into extra-renal tissues.
Strategies to improve DBP plasma concentrations in patients through stimulation of endogenous production or intra-venous administration have not been tested in humans. Administration of (human) DBP to healthy animals was not associated with toxic effects, except the formation of antibodies against the human form of DBP(Reference Pihl, Jørgensen and Santoni-Rugiu90). In vitro models have, however, shown that exposure to the actin–DBP complex causes inflammation and cell death(Reference Ge, Trujillo, Miller and Kew91). The safety profile may therefore depend on the presence of actin in the extracellular space. Therefore, caution is warranted with strategies to rapidly and extensively increase the DBP concentration in the presence of extracellular actin as this may increase the inflammatory response(Reference Kew72).
Vitamin D status and metabolism in acute and critically ill patients
Vitamin D deficiency is commonly found in critically ill patients of all ages(Reference Vo, Koppel and Espinola63,Reference Silva and Furlanetto85,Reference Waldron, Ashby and Cornes87,Reference Amrein, Oudemans-van Straaten and Berger92–Reference Quraishi and Camargo96) and is associated with increased mortality and morbidity(Reference Ney, Heyland and Amrein16,Reference Kempker, Tangpricha, Ziegler and Martin17) . It is unclear whether vitamin D deficiency in these patients is a marker of (pre-existing) poor health or low supply prior to hospitalisation (due to limited opportunities for spending time outdoors and/or low dietary intake), or develops as a result of altered metabolism, or whether low tissue availability plays a role in the disease process. In many but not all studies, a decrease in 25(OH)D is reported over the course of hospitalisation(Reference Ney, Heyland and Amrein16,Reference Silva and Furlanetto85,Reference Waldron, Ashby and Cornes87,Reference Zajic, Heschl and Schorghuber97–Reference Lohia, Kapur, Patel and Seyoum101) . Also, a lower plasma 1,25(OH)2 D concentration compared with matched controls and a decrease in 1,25(OH)2 D is reported in critically ill patients and in patients undergoing major surgery(Reference Ney, Heyland and Amrein16,Reference Quraishi, Bittner, Blum, McCarthy, Bhan and Camargo95,Reference Zajic, Heschl and Schorghuber97,Reference Li, Tang and Wasnik102–Reference Ingels, Vanhorebeek and Van Cromphaut105) .
In a longitudinal observational study, Ney(Reference Ney, Heyland and Amrein16) showed that pre-operative 1,25(OH)2 D concentrations in patients undergoing major cardiac surgery was a negative predictor of post-operative organ failure, sepsis, mortality and prolonged hospital stay, whereas pre-operative 25(OH)D was not predictive. Although the relationship with post-operative 1,25(OH)2D with health outcomes was not reported, pre- and post-operative concentrations seemed to be correlated, and those with low pre-operative values had a further decline in 1,25(OH)2D. This parallels findings of a study in ICU patients treated with a high bolus dose of vitamin D. Post-hoc analyses showed that the 1,25(OH)2 D concentration, not 25(OH)D, measured in plasma 3 d after vitamin D administration was predictive of 28-d survival(Reference Martucci, McNally and Parekh99). This indicates that renal activation of 25(OH)D into (systemic) 1,25(OH)2D and maintenance of plasma 1,25(OH)2D concentrations play a key role.
Several mechanisms provide potential explanations (for a summary, see Fig. 1). Renal impairment and AKI are highly prevalent in ICU patients. This is also found in patients with severe COVID-19 symptoms. Renal damage reduces the capacity to convert 25(OH)D into 1,25(OH)2D(Reference Chand, Kapoor and Orsi106,Reference Robbins-Juarez, Qian and King107) and leads to renal losses of vitamin D metabolites bound to DBP and albumin(Reference Waldron, Ashby and Cornes87,Reference Li, Tang and Wasnik102) . Further, animal models of sepsis show that renal 1,25(OH)2D production may have decreased as a result of decline in insulin growth factor 1 (IGF-1; known to stimulate 1,25(OH)2D production), despite an increase in PTH, commonly observed in sepsis. Catabolism may be expected to be increased due to an increase in FGF23 (known to decrease the half-life of 1,25(OH)2D). Finally, the decline in DBP observed with acute and critical illness (see further below) is expected to be associated with an increase in the free fraction and, therefore, catabolism of vitamin D metabolites(Reference Schoenmakers, Jones, Feldman, Pike, Goltzman, Giovannucc, Hewison and Bouillon71).
An alternative explanation for the decrease in 1,25(OH)2D concentrations is a functional down-regulation of the total concentration to maintain free 1,25(OH)2 D concentrations constant. This is observed in several studies conducted after surgery, where the free fractions of 25(OH)D and 1,25(OH)2D remained constant in the presence of a decrease in DBP(Reference Briggs, Kuan and Greiller108,Reference Reid, Toole and Knox109) . A longitudinal study in ICU patients, however, showed that the decline in 1,25(OH)2D and 25(OH)D far exceeded the decline in DBP, rendering the free fractions of these metabolites significantly lower than in healthy controls(Reference Ingels, Vanhorebeek and Van Cromphaut105).
Severe trauma (e.g. hip fracture), major surgical procedures and severe illness (including septic shock, ARDS) lead to pronounced changes in DBP concentrations(Reference Silva and Furlanetto85–Reference Meier, Gressner, Lammert and Gressner88). Although not detailed in most reports, this should be assumed to reflect the actin-free DBP concentration, which is measured in most types of assays. Where total DBP is measured, the concentration has been reported to increase(Reference Meier, Gressner, Lammert and Gressner88). In two longitudinal studies in ICU or patients with severe trauma, the total concentration or actin free DBP was shown to initially decrease, while the complexed DBP concentration increased. Subsequently, measured over the course of 7–10 d, actin-free and actin-bound DBP concentrations increased(Reference Dahl, Schiødt, Rudolph, Ott, Kiaer and Heslet82,Reference Ingels, Vanhorebeek and Van Cromphaut105) . Plasma concentrations of DBP (either measured as total, actin-free or actin-bound) are reported to be a sensitive predictor of in-hospital complications, including sepsis and respiratory distress and failure, and of mortality(Reference Kew72,Reference Gomme and Bertolini80,Reference Dahl, Schiødt, Rudolph, Ott, Kiaer and Heslet82,Reference Meier, Gressner, Lammert and Gressner88,Reference Suberviola, Lavin, Jimenez, Perez-San Martin, Garcia-Unzueta and Santibañez110–Reference Yoo, Jung and Ju114) . These data indicate that DBP actin binding capacity and hepatic DBP production in severely ill patients play an important role in survival. It has been hypothesised that, when actin release exceeds DBP scavenging capacity, the formation of actin filaments leads to increased viscosity and increased risk of coagulation(Reference Gomme and Bertolini80,Reference Kim, Ji, Song, Moon, Hur and Yun86,Reference Ingels, Vanhorebeek and Van Cromphaut105) . Considering that DBP is also present in alveolar fluids, its involvement in the prevention and development of airway obstructions may be expected(Reference Chishimba, Thickett, Stockley and Wood77,Reference Gomme and Bertolini80) , although the role of DBP and change in DBP concentrations with cell lysis and inflammation in extra-vascular fluids are mostly unknown.
Venous and arterial thromboembolic events are common in ICU patients and those with severe COVID-19, affecting approximately 30 % of cases admitted to intensive care and occurring despite thromboprophylaxis(Reference Rhodes, Subramanian, Laird, Griffin and Kenny56). In pre-clinical studies, vitamin D has been shown to have anti-thrombotic effects, and there has been considerable interest in the effects of vitamin D on coagulation, but large intervention studies have failed to show an impact of vitamin D status or supplementation on the risks for thromboembolism or cardiovascular disease(Reference Quesada-Gomez, Entrenas-Castillo and Bouillon10). This is possibly related to the earlier described reduced capacity to convert 25(OH)D into 1,25(OH)2D in patients typically at increased risk and depletion of actin scavenging capacity of DBP and gelsolin and the concomitant decrease in plasma albumin concentration, which are not influenced by vitamin D supplementation(Reference Chand, Kapoor and Orsi106,Reference Robbins-Juarez, Qian and King107) .
Other aspects of altered metabolism, tissue distribution and extracellular volume also need to be considered in the interpretation of plasma concentrations of vitamin D metabolites, DBP and the potential of receptor activation. Treatment protocols of ICU and surgical patients (e.g. fluid load) may lead to significant haemodilution; the distribution of compounds between the vascular and extra-vascular compartments may be changed; and alterations of tissue uptake and receptor expression may modify sensitivity and changes of circadian rhythms (well known to play an important role in vitamin D metabolism and calcium and phosphate homeostasis(Reference Jones, Redmond and Fulford115)), all of which may lead to pronounced changes in plasma concentrations of any biochemical marker(Reference Hassan-Smith and Cooper116–Reference Quraishi and Camargo118). All these factors and mechanisms may have implications for the interpretation of findings in ICU patients and potential treatment strategies.
Randomised controlled trials with vitamin D in ICU and acutely ill patients
There is a limited number of published RCTs with administration of native vitamin D(Reference Amrein, Schnedl and Holl104,Reference Amrein, Sourij and Wagner119–Reference Miri, Kouchek, Rahat Dahmardeh and Sistanizad125) , the pharmaceutical form of 25(OH)D (calcifediol) or 25(OH)D(Reference Ingels, Vanhorebeek and Van Cromphaut105) or 1,25(OH)2D(Reference Leaf, Raed, Donnino, Ginde and Waikar126) in critically ill patients. In the majority of studies, vitamin D or 25(OH)D was given as either a high bolus or a loading dosage followed by lower maintenance doses. This strategy is chosen when time is critical and has been shown to lead to a rapid correction of vitamin D deficiency(Reference Schoenmakers, Jones, Feldman, Pike, Goltzman, Giovannucc, Hewison and Bouillon71,Reference Ingels, Vanhorebeek and Van Cromphaut105) . These trials were designed (and powered) for different primary and secondary outcomes, and the majority were of small size (n < 100) and differed in study population. These trials reported conflicting results. Several meta-analyses were conducted and focused on hard clinical end points. Jointly, meta-analyses of these trials showed no effect on length of hospital or ICU stay and/or requirement for mechanical ventilation(Reference Putzu, Belletti and Cassina127–Reference Langlois, Szwec, D’Aragon, Heyland and Manzanares130). No effect on mortality (total 28-d or split by mortality in hospital or ICU; mortality after 7 d, 28–30 d, 84 d and 6 months) was reported in three of the meta-analyses(Reference Lan, Lai, Chang, Lu, Hung and Lin128–Reference Langlois, Szwec, D’Aragon, Heyland and Manzanares130), while Putzu(Reference Putzu, Belletti and Cassina127) reported a lower odds ratio (0·70 CI: 0·50–0·98) for total mortality (as recorded at the last day of data collection of an individual study) with vitamin D supplementation. The largest, recent multi-centre RCT (n = 1360)(Reference Ginde and Talmor123) was only included in the meta-analyses conducted by Lan(Reference Lan, Lai, Chang, Lu, Hung and Lin128). This RCT recruited vitamin-D-deficient (defined as 25(OH)D < 50 nmol/L) patients at high risk of death or lung injury, and a bolus of vitamin D (540,000 IU D3) was given within 12 h after ICU admission. This trial found no effect on 28- or 90-d mortality, days on ventilation and length of stay in hospital or other health care facilities, and none of the wide range of other health outcomes and measurements (which included the percentage of patients that developed ARDS) reached statistical significance. The trial, originally designed to recruit 3000 patients, was stopped on the basis of interim analyses predicting no effect of treatment.
Trials varied in form, dosages, frequency and length of administration of vitamin D or its metabolite. The route of administration was oral, intra-venous or intramuscular. Considering the differences in pharmacokinetic profiles and bio-availability as influenced by the form of vitamin D (native or its metabolite(s)), dosage, frequency and length of administration and route of administration (reviewed in Ref.(Reference Schoenmakers, Jones, Feldman, Pike, Goltzman, Giovannucc, Hewison and Bouillon71)), this may be expected to have influenced the results of individual trials. The majority of studies administered a bolus or provided vitamin D for a short length of time. Two meta-analyses analysed the influence of factors affecting the pharmacokinetic profiles. In these, no significant interaction was found between outcomes and route, length and dose of administration(Reference Lan, Lai, Chang, Lu, Hung and Lin128,Reference Langlois, Szwec, D’Aragon, Heyland and Manzanares130) . However, the validity of outcomes reported after more than five times the half-life of either 25(OH)D or 1,25 (OH)2D is questionable (25(OH)D: ˜ 2–3 weeks; 1,25(OH)2D: ˜ 5 h), unless these were reported as cumulative data. In addition, administration of bolus dosages, although leading to a rapid increase in 25(OH)D, has a disadvantageous pharmacokinetic profile as set out below in the section ‘Symptoms and correction of vitamin D deficiency’. Studies designed to initially rapidly correct vitamin D deficiency, followed by a maintenance dose schedule, may be more suitable to evaluate the effect of vitamin D administration in ICU patients.
Most of the intervention studies in ICU patients also showed no effect on plasma concentrations of markers of immune function and inflammatory or pro-inflammatory markers such as cathelicidin, interleukin 6 (IL-6), C-reactive protein and procalcitonin, measured 6 h to 14 d after (the start of) vitamin D administration(Reference Ingels, Vanhorebeek and Van Cromphaut105,Reference Amrein, Sourij and Wagner119,Reference Han, Jones and Tangpricha121,Reference Ginde and Talmor123,Reference Han, Alvarez and Jones131) . However, Leaf(Reference Leaf, Raed, Donnino, Ginde and Waikar126) demonstrated that 1,25(OH)2D administration increased the leucocyte mRNA expression of cathelicidin and interleukin-10 in sepsis patients. Quraishi(Reference Quraishi, De Pascale and Needleman120), also in a study with sepsis patients given enteral vitamin D (400,000 IU as a bolus), found an increase in plasma cathelicidin and a decrease in IL-6 after 5 d. This study also reported a range of other inflammation markers (e.g. C-reactive protein, TNF-α and IFN-γ), which were unchanged. This may potentially reflect that, during severe illness, other drivers of inflammation have a predominant effect.
The dose–response to vitamin D administration in ICU patients is poorly characterised and not formally subjected to meta-analyses. Studies have shown that the response to vitamin D and 25(OH)D given both orally and intravenously to ICU patients is blunted compared with healthy people(Reference Ingels, Vanhorebeek and Van Cromphaut105). This is likely a result of impaired hepatic conversion, increased renal losses and a more rapid catabolism. In generally healthy older people, vitamin D supplementation at high bolus dosages appears not to have beneficial effects compared with lower daily or weekly dosages, but has a disadvantageous pharmacokinetic profile(Reference Schoenmakers, Jones, Feldman, Pike, Goltzman, Giovannucc, Hewison and Bouillon71), and it may increase the propensity of falls(Reference Sanders, Stuart and Williamson132,Reference Griffin, Hewison and Hopkin133) (https://cks.nice.org.uk/topics/vitamin-d-deficiency-in-adults-treatment-prevention and www.endocrinology.org/media/3593/nos_vitamin_d_and_bone_-health_in_adults_web.pdf). In addition, it may increase the risk of hypercalcaemia and hypercalciuria(15). This appears to be a limited issue in ICU patients. Meta-analyses of the occurrence of hypercalcaemia in RCTs in ICU patients showed no increased risk after supplementation with high dosages of vitamin D(Reference Putzu, Belletti and Cassina127). Plasma 1,25(OH)2D concentrations increased with supplementation and were well over the normal reference range in some patients(Reference Martucci, Tuzzolino and Arcadipane98,Reference Amrein, Schnedl and Holl104,Reference Amrein, Sourij and Wagner119,Reference Leaf, Raed, Donnino, Ginde and Waikar126) , but were not associated with hypercalcaemia. This is in line with the observation that hypercalcaemia is relatively uncommon and hypocalcaemia is frequently observed in ICU patients(Reference Sun, Zhang and Zou35,Reference Jacobi134) . A decrease in total calcium is thought to be partly an adaptive response to maintain the biologically active fraction, ionised calcium, constant in the presence of a decrease in plasma binding proteins, the concentrations of which decrease with acute and critical illness. However, also the ionised calcium concentration is often below normal ranges with sepsis and other acute inflammatory illnesses. Although mortality rates are higher in patients with sepsis with hypocalcaemia, replacement therapy is not associated with improved outcomes(Reference Jacobi134).
Current research investigates the effects of administration of the pharmaceutical form of 25(OH)D, calcifediol and active vitamin D or analogues in critically ill patients(Reference Ingels, Vanhorebeek and Van Cromphaut105,Reference Leaf and Christov135,Reference Amrein, Papinutti, Mathew, Vila and Parekh136) . With these forms, the consequences of impaired absorption and activation of native vitamin D may be reduced. Patients with intestinal malfunction, fat malabsorption and poor liver function may benefit more from supplementation with 25(OH)D. The absorption of this form does not depend on fat absorption or the formation of chylomicrons, does not require hepatic hydroxylation and leads to a faster and proportionally higher increment in plasma 25(OH)D per unit given compared with vitamin D(Reference Schoenmakers, Jones, Feldman, Pike, Goltzman, Giovannucc, Hewison and Bouillon71,Reference Vaes, Tieland, de Regt, Wittwer, van Loon and de Groot137–Reference Perez-Castrillon, Duenas-Laita and Brandi140) . In addition, limited evidence in CKD stage 2–4 patients has shown that administration of the slow-release formulation of 25(OH)D is not associated with an increase in FGF23(Reference Sprague, Silva and Al-Saghir141,Reference Sprague, Crawford and Melnick142) , observed with higher dosages of oral vitamin D(Reference Zittermann, Berthold and Pilz143). This may be of particular importance for severely ill patients since, in many, production of renal and possibly extra-renal 1,25(OH)2D is impeded due to renal impairment and increased catabolism, as described in the previous section. To date, the availability of this form is, however, limited. In patients with renal impairment and AKI, administration of active vitamin D or analogues may be considered, similar to those for patients with CKD. A combination with native vitamin D to ensure tissue availability of 25(OH)D for extra-renal hydroxylation may be beneficial in this group(Reference Leaf and Christov135,144) .
Together, the contrasting findings between observational, mechanistic and intervention studies suggest that many factors other than vitamin D status and intake play a role. The extensive alterations of vitamin D metabolism with disease processes may influence its function. With acute and severe illness, other drivers and regulatory factors of the immune and inflammatory response may be predominant and may be determined by alterations in organ function and tissue damage.
Implications for practice
Prevention of vitamin D deficiency and population guidelines
Thresholds for vitamin D deficiency for the general population differ between advisory bodies, but most define plasma 25(OH)D values below 25–30 nmol/L as deficient (reviewed in Refs.(23–Reference Christodoulou, Aspray and Schoenmakers25,Reference Bouillon145) ). There is considerable variation between health authorities in the definition and thresholds or ranges of 25(OH)D concentration that encompasses vitamin D sufficiency or target ranges of 25(OH)D on which to base dietary recommendations(Reference Christodoulou, Aspray and Schoenmakers25,Reference Bouillon145) . Recommended 25(OH)D target concentrations and thresholds for deficiency for specific patient groups and clinical management may be higher than for generally healthy people (reviewed in Refs.(Reference Christodoulou, Aspray and Schoenmakers25,Reference Bouillon145) ).
The threshold for deficiency is the concentration of 25(OH)D below which the risk of disease increases. This is predominantly based on health outcomes related to calcium metabolism and musculoskeletal health, but in some guidelines also other health outcomes are considered. Although vitamin D deficiency is associated with many other health outcomes, discordant findings between observational studies and RCTs of vitamin D treatment are reported. An overview is beyond the scope of this manuscript and reviewed elsewhere(Reference Rejnmark, Bislev and Cashman26–Reference Akutsu, Kitamura, Himeiwa, Kitada, Akasu and Urashima28).
Population guidelines for dietary vitamin D intakes are partly based on the required intakes to prevent vitamin D deficiency or to achieve pre-defined target ranges of 25(OH)D or sufficiency. Also evidence from RCTs and other research designs linking vitamin D intake and status to health outcomes are considered. Dietary reference values or equivalents, defined by different public health institutes, vary as a consequence of differences in the defined threshold for deficiency, sufficiency or target values for 25(OH)D (reviewed in Refs.(Reference Christodoulou, Aspray and Schoenmakers25,Reference Bouillon145) ). Guidelines for dietary intakes and supplementation also depend on age group, physiological state, risk factors (as per below) and, in some countries, season. An overview of population guidelines from health authorities across the world was recently published by Bouillon(Reference Bouillon145). In most guidelines, it is acknowledged that the majority of people do not consume sufficient vitamin D through diet alone and, thus, supplementation is needed to meet requirements. In most guidelines, a contribution of cutaneous synthesised vitamin D is taken into account in the formulation of recommendations of dietary requirements. Advice on sunshine exposure to maintain vitamin D status is generally not provided in view of the variability in the response to cutaneous exposure and the risk of skin damage.
In temperate climates, supplementation is recommended during winter months. Supplementation throughout the year is recommended for pregnant women and people at increased risk of deficiency. At risk are population groups with a dark skin tone, obesity and limited skin exposure to the sun (i.e. with a habitual dress style that covers most of the body, frequent sunscreen use, limited access to the outdoors, e.g. when mobility is limited or living in residential care and during illness). It seems appropriate to also apply this recommendation to those that are in prolonged self-isolation and therefore mostly indoors. Screening of vitamin D status is not recommended for the general population. This is limited to individuals at increased risk of deficiency (as described above) or who are symptomatic(15,23,Reference Bouillon145) .
Symptoms and correction of vitamin D deficiency
The main manifestation of severe vitamin D deficiency is osteomalacia in adults and rickets in children. It is associated with secondary hyperparathyroidism, bone loss, muscle weakness, falls and fragility fractures and, in children, also cardiomyopathy and hypocalcaemia. Clinical symptoms of vitamin D deficiency are non-specific and include generalised muscle, joint and bone pain and hyperalgesia, fatigue and muscle weakness, especially of the extremities and pelvic region, manifesting in difficulties in rising from a sitting or squatting position or a waddling gait(Reference Kennel, Drake and Hurley146) (https://www.endocrinology.org/media/3593/nos_vitamin_d_and_bone_-health_in_adults_web.pdf).
When vitamin D deficiency is present (ideally assessed on basis of plasma 25(OH)D), or strongly suspected on the basis of clinical symptoms in combination with primary risk factors (see previous sections), higher intakes than daily population requirements are required. Recommendations for dosage regimens depend on local health authorities and specific patient characteristics and target ranges for 25(OH)D (see section below). In the UK, an intake of 800 IU/d is advised when vitamin D deficiency is mild and correction is not urgently required. This approach does not generally require clinical testing and management. When rapid correction of deficiency is needed, such as in patients with severe deficiency or with symptomatic disease, the recommended treatment regimen is based on fixed loading doses followed by maintenance therapy. Loading regimens aim to provide a total of approximately 300,000 IU given over a period of 6–10 weeks as weekly or daily split doses. Similarly, the US Endocrine Society recommends a loading dose of 300,000–400,000 IU given over 8 weeks(Reference Bouillon145,Reference Holick, Binkley and Bischoff-Ferrari147) . Maintenance regimens recommend intakes of 800–2000 IU daily (up to a maximum of 4000 IU daily), given either daily or intermittently at a higher equivalent dose. Treatment with these higher dosage schedules should be monitored with appropriate clinical testing and management. Large loading dosages exceeding 60,000 IU given at once should be avoided (https://cks.nice.org.uk/topics/vitamin-d-deficiency-in-adults-treatment-prevention and www.endocrinology.org/media/3593/nos_vitamin_d_and_bone_-health_in_adults_web.pdf)(Reference Bouillon145,Reference Holick, Binkley and Bischoff-Ferrari147) . High bolus dosages are associated with an increased risk of falling in adults. In addition, higher dosages, including when given as a bolus, have a different and potentially disadvantageous pharmacokinetic profile(Reference Schoenmakers, Jones, Feldman, Pike, Goltzman, Giovannucc, Hewison and Bouillon71,Reference Ketha, Thacher, Oberhelman, Fischer, Singh and Kumar148) ; with a high bolus dose, the plasma concentration of 25(OH)D rapidly increases, and there may also be a small increase in 1,25(OH)2D(Reference Prié and Friedlander149). This is followed by an increase in catabolism through increased 24-hydroxylation. This is partly mediated through an increase in FGF23(Reference Griffin, Hewison and Hopkin133,Reference Zittermann, Berthold and Pilz143,Reference Charoenngam, Rujirachun, Holick and Ungprasert150) and 1,25(OH)2D itself(Reference Prié and Friedlander149,Reference Turner, Dalton, Inaoui, Fogelman, Fraser and Hampson151) . This results in increased conversion into 24,25(OH)2D and potentially other catabolic products from 25(OH)D and possibly vitamin D itself. Also, the rate of catabolism of 1,25(OH)2D is expected to increase(Reference Griffin, Hewison and Hopkin133,Reference Prié and Friedlander149,Reference Turner, Dalton, Inaoui, Fogelman, Fraser and Hampson151) .
Guidelines for prevention and correction of vitamin D deficiency in specific patient groups
Recommended intakes, 25(OH)D target concentrations and thresholds for deficiency for specific patient groups and clinical management may be higher than for generally healthy people(Reference Bouillon145). They consider altered supply or bio-availability, losses, increased metabolism and/or requirements or a combination of these factors. Little is known about the vitamin D requirements during acute or chronic illness. Even for chronic conditions that clearly affect vitamin D metabolism (such as renal(144) and hepatic disease(152–Reference Hirschfield, Dyson and Alexander154)) or bioavailability (conditions affecting intestinal fat and/or vitamin D absorption such as with coeliac and inflammatory bowel disease(Reference Ludvigsson, Bai and Biagi155,Reference Lamb, Kennedy and Raine156) ), direct evidence on requirements is scarce. Also certain types of medication are associated with altered vitamin D metabolism, such as corticosteroids, anti-epileptic drugs and drugs inhibiting fat absorption(Reference Bouillon145,Reference Holick, Binkley and Bischoff-Ferrari147) (https://cks.nice.org.uk/topics/vitamin-d-deficiency-in-adults-treatment-prevention), or increased dietary calcium requirements, such as medications reducing bone loss. With these conditions, specific patient management guidelines should be followed, where formulated (renal(144,Reference Christodoulou, Aspray and Schoenmakers25) ; hepatic(152–Reference Hirschfield, Dyson and Alexander154); coeliac(Reference Ludvigsson, Bai and Biagi155); inflammatory bowel disease(Reference Lamb, Kennedy and Raine156); osteoporosis (theros.org.uk)). Many guidelines recommend regular testing of vitamin D status and correction of deficiency if and as required with monitoring of side effects and until a target plasma concentration or range of 25(OH)D is reached. Dependent on the aspect of metabolism affected and severity or disease, guidelines may recommend prescribing native vitamin D, 25(OH)D or active vitamin D or their analogues(144,152–Reference Hirschfield, Dyson and Alexander154,Reference Ketteler, Block and Evenepoel157–Reference Moe and Dreke159) .
Management of vitamin D status in people with acute and critical illness
Little is known about the vitamin D requirements during acute and critical illness or with inflammation(23,Reference Amrein, Papinutti, Mathew, Vila and Parekh136) .
For patients with mild and short-term acute illnesses, such as during a mild disease course following infection with SARS-CoV-2 and/or during self-isolation, supplementation with vitamin D to prevent vitamin D deficiency according to population guidelines for groups with no or limited sun exposure is appropriate(23,Reference Bouillon145) . In the presence of risk factors or symptoms of deficiency, testing of vitamin D status and correction of vitamin D deficiency is warranted, as described above.
There is no specific guidance to prevent vitamin D deficiency in severely ill patients(Reference Amrein, Papinutti, Mathew, Vila and Parekh136). The standard formulations for enteral feeding contain 200–400 IU vitamin D per day, and parenteral multivitamin preparations typically contain only 200 or 220 IU per day. This is below the population recommendations in the majority of countries. As outlined in previous sections, patients with acute and critical illness are often vitamin D deficient at admission and may have a rapid decline in vitamin D status, suggesting that vitamin D requirements are increased. This may be the result of altered bioavailability or metabolism, increased utilisation and losses of 1,25(OH)2D and 25(OH)D and medication use increasing catabolism, such as corticosteroids. The most recent update for critically ill patients recommends screening of patients for vitamin D deficiency and those with plasma 25(OH)D concentrations below 30 nmol/L to be supplemented with a single high dose of vitamin D3 (500,000 IU) within a week after admission(Reference Barazzoni, Bischoff and Breda160,Reference Singer, Blaser and Berger161) . No guidance is available regarding monitoring, frequency of testing and maintenance therapy. Considering the likelihood of increased requirements, it seems reasonable to provide regular (daily or weekly) vitamin D supplementation similar to the maintenance schedules recommended for patients after correction of vitamin D deficiency, as described above with regular testing of plasma 25(OH)D.
In consideration of underlying conditions and acute alterations in organ function with critical illness, the use of activated or partly activated vitamin D metabolites may be considered. Current research investigates the effects of administration of 25(OH)D and active vitamin D in critically ill patients, but this research is as yet not incorporated in guidelines.
Conclusions
No data are yet available demonstrating a causative link between low vitamin D status and susceptibility for COVID-19 or its disease course. Data from observation studies investigating the association between vitamin D status and risk of infection and/or developing severe symptoms are conflicting. Vitamin D deficiency is highly prevalent in patients hospitalised with COVID-19 and with other conditions, particularly those that are severely ill. This may be related to the presence of risk factors for vitamin D deficiency, associated with their general poor health status or increased catabolism and losses of 25(OH)D during disease. There are extensive alterations in vitamin D metabolism with severe illness and inflammatory processes which may increase requirements of vitamin D. This needs consideration in the assessment of associations with disease risk and severity and in patient management. Although mechanistic studies show that vitamin D metabolites and DBP have multi-factorial roles in pulmonary health, immune function and inflammatory response to viral infection and tissue damage, supplementation of ICU patients has so far, however, shown little effect. The contrasting findings between observational, mechanistic and intervention studies suggest that many other factors play a role. Alterations in vitamin D metabolism with disease processes may influence its function, and/or other drivers of the immune and inflammatory response may be predominant. The effect may depend on when supplementation is initiated relative to the development of disease symptoms and progression to severe illness and the frequency of administration. Such data are not yet available. The beneficial effects of vitamin D may also be limited to specific subgroups.
There is some evidence that vitamin D supplementation decreases the risk of respiratory tract infections, particularly in children, although the risk reduction is moderate. No such evidence is yet available for the prevention or treatment of COVID-19. In view of the high prevalence of vitamin D deficiency, pro-actively applying current population and patient management guidelines to prevent and correct vitamin D deficiency with screening and monitoring of vitamin D status in individuals who are at increased risk is appropriate.
Acknowledgements
This work was supported by The Academy of Medical Sciences [SBF002\1097] and the University of East Anglia Health and Social Care Partners, UK.
Author contributions
I.S.: conceptualisation; I.S., W.D.F. and A.F.: all authors have contributed to the interpretation, drafting and approval of the final version of this manuscript.
The authors declare no conflicts of interest.