INTRODUCTION
Autism is a complex developmental disorder marked by impairments in reciprocal social interaction and communication, and the presence of restricted, repetitive behaviors and interests. Behavioral, psychophysiological, and neuroimaging studies in autism consistently demonstrate deficits in the building blocks for social interaction, such as impaired recognition and memory for facial and vocal expressions (Golan et al., 2006; Humphreys et al., 2007; Paul et al., 2005); reduced use and recognition of emotionally salient aspects of imitation and gesture (Colgan et al., 2006; Hobson & Meyer, 2005); and limited consideration of others' mental states in joint attention or theory of mind tasks (Baron-Cohen et al., 1999; Rutherford et al., 2006).
Research regarding the neurobiological origins of autism has focused on identification of brain mechanisms that are essential for the perception and integration of information that is rich in social meaning (Schultz, 2005). Findings from anatomical, behavioral, and functional neuroimaging studies in autism have suggested abnormal structure and function of the amygdala (Baron-Cohen et al., 2000; Munson et al., 2006), although there remain many inconsistencies in results and in interpretation. Bauman and Kemper (1985, 1988) published seminal findings of normal volume but smaller neuron size and increased cell density in the amygdala as well as several other brain regions of autopsied brains of people with autism. Recent neuroimaging studies of amygdala volume in autism have been mixed, with some reporting increased volume relative to controls (Howard et al., 2000; Sparks et al., 2002), others showing reduced volume (Abell et al., 1999; Pierce et al., 2001), and others finding no difference (Bigler et al., 2003; Haznedar et al., 2000). A cross-sectional study of age effects by Schumann et al. (2004) reported that amygdala volume in an autism sample 7.5 to 12.5 years of age was larger than the comparison group, but there was no difference in the adolescent groups. Bigler et al. (2003) have pointed out numerous methodological limitations in previous reports of volumetric measurements and further conclude that brain abnormalities in autism are likely more related to functional organization than to gross volumetric differences.
At least five research groups have reported hypoactivation of the amygdala during social perception tasks (Ashwin et al., 2006; Baron-Cohen et al., 1999; Grelotti et al., 2005; Schultz et al., 2005; Wang et al., 2004). Munson et al. (2006) found that larger right amygdala volume is positively correlated with atypical social development in young children with autism. However, other neuroimaging studies of social perception in autism have suggested that such findings may be related to task-specific characteristics such as the familiarity of the face stimuli (Pierce et al., 2004; see also Hadjikhani et al., 2004). Eye gaze appears to rely in part on amygdala function (Adolphs et al., 2005). Studies of eye gaze in autism have reported discrepancies for dynamic social stimuli (Klin et al., 2002; Speer et al., 2007), although eye gaze for static photos is more intact (Pelphrey et al., 2002; van der Geest et al., 2002).
The amygdala is a complex set of separate, but closely connected nuclei at the base of the temporal lobes that are critically involved in early emotion perception and response (Davis & Whalen, 2001). It is critically involved in the detection of, and learning about, emotionally relevant stimuli, including events that have high social salience such as recognizing the meaning of facial expressions (Pasley et al., 2004; Vuilleumier & Pourtois, 2007). The “amygdala theory” of autism (Baron-Cohen et al., 2000; see also Bachevalier, 1996; Howard et al., 2000; Schultz et al., 2003) proposes that atypical social development in autism may arise from primary dysfunction in the amygdala and related “social brain” structures (Brothers, 1996; Skuse et al., 2003).
Social Information Processing in Autism
Waterhouse et al. (1996) and Schultz (2005) have proposed that there may be a general amygdala deficit in autism that disrupts the assignment or association of reinforcement value to incoming stimuli. Schultz (2005) speculated how such a deficit may specifically impair social development in autism: during face perception, autistic infants experience reduced reinforcement from the amygdala to enhance innate preference for faces (Kelly et al., 2005; Turati et al., 2002). This leads to a reduction in attention for faces (Adolphs et al., 2005) and a subsequent failure to develop face-specific skills in related brain areas such as face regions of the fusiform gyrus, as has been frequently shown among individuals with autism (e.g., Schultz et al., 2000; Wang et al., 2004). Such failure could markedly reduce the development of social scaffolding (i.e., an impaired “language of faces”), leading to further deficits in social and communicative functioning and to the emergence of repetitive behaviors due to social isolation (Suomi & Harlow, 1971).
Whereas Schultz's model highlights the amygdala's role in social perception deficits in autism, Bachevalier and Loveland (2006) focus on developmental abnormalities in the amygdala in relation to the regulation of social behavior, that is, “the ability to select and initiate complex behaviors in response to the specific conditions of the social environment” (Bachevalier & Loveland, p. 98). This skill requires a cooperative effort between the amygdala, which detects emotional significance, and the orbitofrontal cortex, which evaluates the functional significance of inputs from the amygdala in the context of a constantly changing social world (Loveland, 2001; see also Sabbagh, 2004; Vollm et al., 2006). In general, orbital frontal cortex and related areas of the ventromedial prefrontal cortex (vmPFC) are critical for comparing past and present emotional salience, and for modulating amygdala activation in response to environmental changes (Rauch et al., 2006; Sotres-Bayon et al., 2004). Disruption at any point in this ongoing cycle of perception and action would adversely affect both processes and impair the ability to track and respond to rapid changes in social situations (including gestures, face and voice expressions, and variations in verbal meaning).
Nonsocial Emotion Processing in Autism
In contrast to research on social cognition in autism, there is little research regarding perception and learning for nonsocial emotions, despite the critical role of the amygdala in many processes that involve emotion more generally (reviewed by Davis and Whalen, 2001). For instance, the amygdala plays a critical role in the detection of naturally occurring threats (Merckelbach et al., 1995; Ohman, 2005; see also Larson et al., 2006); classic conditioning to aversive stimuli (Cheng et al., 2006; Phelps & LeDoux, 2005); and the anticipation of nonsocial reward (Balleine & Killcross, 2006; Bechara et al., 1995).
Although an early influential review by Baron-Cohen et al. (1999) recommended that future studies examine both fear recognition and fear conditioning in autism, only a few studies published since then have specifically examined fear outside of the social context. Bernier et al. (2005) found no differences in latency or amplitude of the potentiated startle reflex between autism spectrum and comparison groups, suggesting that the amygdala mechanisms involved in fear conditioning and subsequent potentiation of the startle pathway may not be affected in autism. Johnson et al. (2006) found mixed evidence for abnormality in Asperger's syndrome on the Iowa Gambling task, which has been shown to depend on the critical emotion monitoring loop between the amygdala and medial prefrontal cortex described above.
Taken together, functional and structural imaging research suggest amygdala abnormalities in autism, at least for face processing, while the few existing studies that use fear conditioning without a social element have found intact performance in autism. Thus, it is unclear whether there is global amygdala abnormality in autism, or whether impaired function is specific to socially relevant emotion stimuli. The purpose of the present study was to determine whether individuals with autism exhibit abnormal processing of emotion stimuli or situations that are not socially relevant but that are nevertheless known to depend on the integrity of the amygdala.
To that end, we used four behavioral tasks that are known to engage amygdala activity for the evaluation of emotional significance (see Baxter & Murray, 2002; Phelps & LeDoux, 2005). Consistent with the amygdala theory of autism, we expected that our sample of 37 adolescents and adults with autism would demonstrate reduced facilitation of emotional cues, relative to an age- and IQ-matched comparison group, even in the absence of socially salient information.
METHODS
Participants
A total of 37 (36 males) participants with autism (the AUT group) and 38 (36 males) typically developing comparison participants (the TC group) completed the study. IQ testing was completed at the same time as other study measures, with three exceptions for participants who had been part of previous research projects: one 29-year-old AUT group participant whose IQ data were 4 years old, and 2 TC group participants whose IQ data were 3 years old. This study was conducted as part of a larger research project that used a standard algorithm for comparing scores from the different IQ tests given as appropriate to age and developmental status. The algorithm and the number of participants tested with each measure are shown in Table 1. Groups were comparable for age and IQ (see Table 2).
Algorithm of factor definitions used for each IQ test
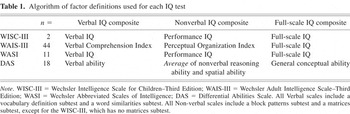
Group comparisons of age and IQ scores
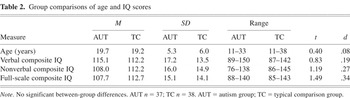
All AUT group participants were diagnosed with Autistic Disorder by one or more authors (J.E.L., W.M.M., S.O., M.S.) experienced in the diagnosis of autism. Diagnostic decisions were based on individual observation, parent interview, and data from structured research assessments (summarized in Table 3) including the Autism Diagnostic Interview–Revised (ADI-R; Lord et al., 1994), which measured lifetime severity; and from the Autism Diagnostic Observation Schedule–Generic (ADOS-G; Lord et al., 2000), a measure of current symptom severity. Final diagnosis was according to DSM-IV criteria regardless of whether strict cutoffs were met for the research diagnostic measures.
Summary of diagnostic measures for 35 AUT participants
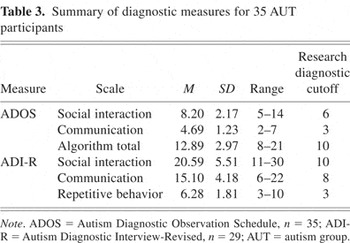
All consent and research procedures were approved by the University of Utah Internal Review Board. Both AUT and TC participants were recruited as part of ongoing research at the Utah Autism Research Project, either from existing research databases, from flyers sent to autism service agencies or posted on the University of Utah campus, or by word of mouth in the community. Experimental tasks were administered via computer using the DirectRT software package (Empirsoft Inc., 2003), except for the Gambling task, which used a stand-alone program. Participants were instructed to use only their dominant hand to respond using a custom-built box with six arcade-style buttons. Most participants (85%) were tested in research offices using a desktop PC (15-inch display); the rest (10 AUT, 1 TC participants) were tested in a quiet place in their home using a notebook personal computer (14-inch display). For all tasks, response latencies and accuracy were recorded and used as dependent variables in statistical analyses.
MEASURES
Brief summaries of the measures, along with evidence for amygdala involvement in task performance and possible functional pathways, are presented in Table 4.
Amygdala contributions to performance on experimental tasks
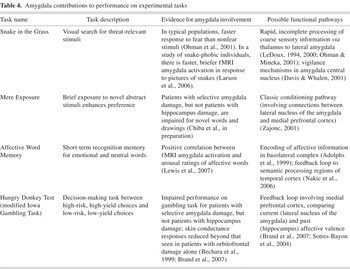
Snake in the Grass Task (Flykt, 2005; Ohman et al., 2001)
LeDoux (1994) proposed a dual-route model through which the amygdala receives input on emotional salience: a coarse but rapid subcortical input directly from the thalamus, and slower routes from cortical sensory areas. Beyond extensive findings from the animal literature, support for the rapid subcortical pathway in humans comes from numerous neuroimaging studies using facial expressions (Morris et al., 1999; Pasley et al., 2004; Reinders et al., 2006). Ohman et al. (2001) reported decreased response time to fear-relevant stimuli (snakes and spiders) versus fear-irrelevant stimuli (flowers and mushrooms) in a visual search task administered to healthy college students. They suggest that this “snake in the grass” effect arises from an evolutionary bias toward immediate detection of threat, mediated through thalamo-amygdala pathways. Recently, Larson et al. (2006) reported brief but strong amygdala responses to pictures of spiders in a functional magnetic resonance imaging (fMRI) study of spider phobic participants, in contrast to weaker but more sustained amygdala activation in healthy comparison participants. Support for this pathway also comes from potentiated startle studies (Merckelbach et al., 1995).
This study used the Snake in the Grass task, which displays a series of 3 × 3 photographic arrays of threat-relevant (spiders or snakes) and threat-irrelevant (flowers or mushrooms) stimuli imposed on a grid background. Participants were instructed to quickly search each array and indicate whether all nine pictures were from the same object category (e.g., all snakes or all mushrooms), or whether there was a discrepant stimulus in the picture (e.g., one spider in the midst of eight flowers). There were 144 stimulus arrays evenly distributed with regard to category (each category appeared the same number of times in both discrepant and nondiscrepant conditions) and grid position. Response time and task accuracy were measured. We expected that reduced emotional facilitation would result in less of a difference in reaction time for the fear versus the nonfear condition.
Mere Exposure Task
In the mere exposure paradigm (Zajonc, 1968), preference for a particular stimulus object is enhanced by repeated exposures to that object, even when the exposure is brief or masked and cannot be recalled in explicit memory. The amygdala is important for implicit (nonconscious) processing of affective stimuli (Hannula et al., 2005; Phelps & LeDoux, 2005) and also plays a key role in the formation of reward-based preference in humans and animals (Adolphs & Tranel, 1999; Gilbert et al., 2003). Although the neural substrates of the mere exposure effect have not been systematically defined, Zajonc (2001) has postulated that it may involve a classic conditioning mechanism involving the amygdala (but see Willems et al., 2002, for alternative explanations). Chiba et al. (in preparation) demonstrated the mere exposure preference effect for participants with either left or right temporal lobe resections that were limited to temporal neocortex and/or the hippocampus, as well as for participants with either left or right partial complex temporal lobe epilepsy. However, participants with left or right temporal lobe resections that included the amygdala in addition to the hippocampus did not display the exposure preference effect.
This study presented an adaptation of the Chiba et al. task. During the first study phase, participants were shown a series of eight real but unfamiliar English words (i.e., higgle, milter), and eight novel abstract grayscale brushstroke drawings created using computer paint software. Each stimulus was shown for 800 ms, followed by a 500-ms interstimulus interval. During the second liking phase, participants were shown each of the original target stimuli sideby-side with a novel distracter stimulus. Participants were instructed to respond as quickly as possible to the question “which one do you like better?” Stimuli remained on the screen until a response was chosen. Stimulus order within a block was different during each phase of the task and was the same for all participants; the presentation of words always preceded the presentation of drawings. We hypothesized that reduced emotional facilitation would be demonstrated by a decreased preference for the target vis-á-vis the distracter stimuli and decreased benefit in reaction time for target versus distracter stimuli.
Affective Word Memory Task
The critical role of the human amygdala for processing affectively charged stimuli has been demonstrated across many modalities, including affective pictures (Sabatinelli et al., 2005); sounds (Sander et al., 2003); odors (Winston et al., 2005); and words (Lewis et al., 2007). Research regarding human responses to affectively charged stimuli has traditionally focused on two dissociated attributes of valence (i.e., whether the affect is positive or negative), and arousal (i.e., how intense is the reaction elicited by the stimulus). A recent fMRI study of viewing affective words (Lewis et al., 2007) found that amygdala activation is more strongly correlated with arousal, whereas vmPFC activation is more strongly associated with valence.
For the present study, participants were initially presented with a list of 40 words appearing sequentially for 1250 ms each (100-ms intertrial interval). There were 12 unpleasant (negative) words (6 low-arousal, e.g., “waste,” and 6 high-arousal, e.g. “crash”); 12 pleasant (positive) words (6 low-arousal, e.g., “relaxed,” and 6 high-arousal, e.g. “riches”); and 16 emotionally neutral words (“paper,” “cork”). All words were short and easy to read, taken from the Affective Norms for English Words (Bradley & Lang, 1999). Participants were next shown a mix of the same 40 target stimuli and 40 new distracter stimuli, also presented sequentially. Instructions were to choose as quickly as possible whether each word had been seen previously; stimuli remained on the screen until the participant responded, up to 8000 ms. Stimulus lists were ordered pseudorandomly so that no valence/arousal combination appeared three times consecutively, and were identical for all participants. Data were collected for response time and accuracy (recall of previously seen words). For this task, evidence of reduced emotional facilitation would consist of less marked benefit in recognition and reaction time for high arousal versus neutral or low arousal words.
Gambling Task
The amygdala response to emotion is modulated by close connections to monitoring regions in the vmPFC, which in humans is essentially involved in rapid integration of sensory information and subsequent decision making (Brothers, 1996). The Iowa Gambling Task (IGT; Bechara et al., 1994) was developed as a laboratory analog of real-life decision making. Participants choose cards from four decks, which indicate varying amounts of reward or punishment. Successful completion requires inhibition of the impulse to take from the decks that provide large reward but also large punishments and eventually result in a net loss. Patients with vmPFC damage perseverate in choosing from the disadvantageous decks, despite showing skin conductance responses in anticipation of making a bad choice (Bechara et al., 1994). In a subsequent study, Bechara and colleagues (1999) found that patients with amygdala damage did not show conditioned response acquisition (i.e., no skin conductance either in anticipation of a bad choice or response to the punishment), despite intact explicit memory of both the conditioned and unconditioned stimulus properties. Patients with damage to the hippocampus but intact amygdala showed appropriate conditioning. Brand et al. (2007) likewise report that three patients with Urbach–Wiethe disease, which selectively damages amygdala bilaterally, demonstrate impaired performance and reduced skin conductance response for the IGT.
We used a variation of the IGT intended to be appropriate for younger or developmentally atypical participants, the Hungry Donkey Test (HDT; Crone & van der Molen, 2004). Instead of choosing cards from decks and using money as the currency, the HDT requires participants to choose from four doors to reveal several apples to feed a “hungry donkey” pictured at the bottom of the screen. Participants are informed that “mean donkeys” behind each door might also take apples away (in which case the pictured apples are crossed out), and that the number of apples behind each door may change from one trial to the next. Two of the doors provided larger rewards on most trials but also unpredictable large losses in either frequent small doses or infrequent large doses; the second set of doors provided for a smaller net gain, but also smaller losses, delivered in identical dose proportions as the first set. Choosing the disadvantageous doors will always result in long-term loss, while the advantageous doors bring small-scale long-term gain. The reinforcement schedule was proportionally the same as for the original Iowa Gambling Task, but the amount was reduced by a factor of 25. There were 200 trials and a graphical representation of net apples gained was displayed throughout the task. The primary variable was the net difference in results (i.e., number of apples earned) resulting from the mean number of advantageous and disadvantageous choices, calculated across 10 blocks of 20 trials each. Choice strategy was analyzed by examining the number of choices made for the two advantageous doors (e.g., small reward but small punishment) versus number of choices made for the two disadvantageous doors. We hypothesized that reduced emotional facilitation would result in a reduced difference in choosing the advantageous doors, that is, a flatter learning curve, as well as a flatter curve for reaction time data.
RESULTS
Due to technical problems or participant refusal, data were missing for 1 TC participant on the Exposure task, 4 participants (3 AUT) for the Snakes task, and 2 participants (1 AUT) for the Words task response time variable. Difficulties with the user interface on the separate Gambling task resulted in lost data for 13 participants (5 AUT, 8 TC). There were no significant differences for age or IQ between diagnostic groups within this smaller sample (n = 62) for the Gambling Task.
Contrary to expectation, both the TC and AUT groups demonstrated clear evidence of emotional facilitation, as shown in Figures 1, 2, 3, and 4. For every task, repeated-measures analysis of variance (ANOVA) analyses on the primary dependent variable demonstrated significant effects of emotion condition and no interaction of diagnostic group × emotion condition. Specific tasks results are detailed below.
Snakes Task
As shown in Figure 1, there was a significant main effect of emotion condition [fear < no-fear, F(1,69) = 39.01; p < .001] and also a main effect for group [AUT > TC, F(1,69) = 7.12; p < .01], but there was no significant group × emotion interaction [F(1,69) = .02; p = .90], indicating the facilitation of the threat stimuli was comparable for both groups. Planned post hoc analyses indicated that the effect of emotion was significant for each group separately, with both groups performing faster during the fear than the no-fear condition (AUT t = 4.0; TC t = 4.89; p's < .001). Both groups correctly identified >95% of the target stimuli, and there were no effects of emotion or group for the accuracy variable.
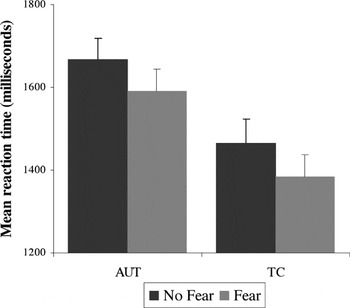
Response time for the Snakes task: main effect of emotion condition (fear condition is faster than no-fear condition) for both groups. There is a main effect (TC faster than AUT) but no group × condition interaction. Error bars represent 1 standard error of the mean. AUT = autism group; TC = typical comparison group.
Mere Exposure Task
As shown in Figure 2, both groups demonstrated a comparably strong “mere exposure” effect, an accuracy measure that indicates a statistically significant preference for previously displayed target stimuli over novel contrast stimuli [F(1,72) = 10.15; p < .001]. Planned post hoc analyses indicated that the effect of exposure was significant for each group separately (AUT t = 4.18; TC t = 4.56; p's < .001). Reaction time analyses showed that the previously seen stimuli were identified faster than distracters for both groups [F(1,72) = 29.37; p < .001]. There was no significant main effect for diagnostic group [F(1,72) = .45; p = .51] or group × exposure condition interaction [F(1,72) = .34; p = .56].
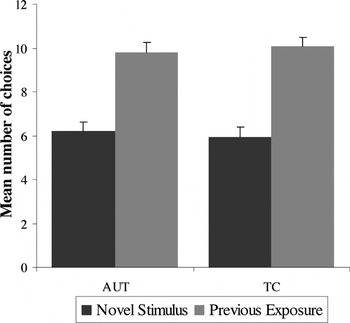
Accuracy for the Mere Exposure task: main effect of condition (previously exposed condition is preferred over the novel condition) for both groups; no main effect for group or group × condition interaction. Error bars represent 1 standard error of the mean. AUT = autism group; TC = typical comparison group.
Affective Words Memory task
The accuracy variable was measured as the percentage of target words that were correctly remembered. As shown in Figure 3, there were significant main effects of emotion condition for separate analyses of valence condition [negative > neutral > positive, F(2,70) = 22.62; p < .001]; and arousal condition [high > neutral > low, F(2,70) = 5.82; p < .001]. There were no significant effects of group or group × condition interaction for either analysis: valence group, F(2,70) = 3.02; p = .09; interaction, F(2,70) = .14; p = .87; arousal group, F(2,70) = 3.02; p = .09; interaction, F(2,70) = .33; p = .72.
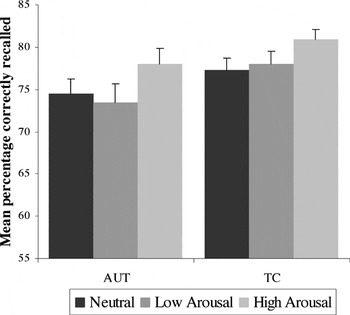
Accuracy for the Emotion Word Recognition task: main effect of arousal condition (high arousal is recalled more accurately than low arousal and neutral conditions) for both groups; no main effect for group or group × condition interaction. Error bars represent 1 standard error of the mean. AUT = autism group; TC = typical comparison group.
Mean reaction times for valence indicated that the negative emotion condition was fastest for both groups: AUT negative M = 1024.1 ms (SD = 245.9), positive M = 1065.78 ms (264.4), neutral M = 1054.1 ms (211.8); TC negative M = 916.77 ms (134.8), positive M = 955.2 ms (155.1), neutral M = 654.68 ms (157.7). The main effect of emotion condition was statistically significant [F(2,70) = 4.20; p < .05], as was the main effect for group [AUT > TC; F(1,71) = 5.77; p < .05]; there was no significant condition × group interaction [F(2,70) = .11; p = .90]. Mean reaction times for arousal indicated that the emotion arousal conditions were faster than neutral for both groups: AUT high arousal M = 1050.15 (257.5), low arousal M = 1039.73 (248.9), neutral M = 1054.1 (211.8); TC high M = 948.6 (156.0), low M = 923.37 (136.4), neutral M = 954.68 (157.7). There was a significant main effect for diagnostic group [AUT > TC; F(1,71) = 5.77; p < .05] but not for emotion condition [F(2,70) = 1.56; p = .21] or a condition × group interaction [F(2,70) = .23; p = .80].
Gambling Task
As seen in Figure 4, both groups demonstrated a change over time in favor of more advantageous responses. Repeated-measures ANOVA analysis using choice condition as the dependent variable, trial block as the within-subjects factor, and diagnostic group as the between-subjects factor showed a significant main effect of time intervals across the 10 blocks, indicative of improved performance over the course of the experiment: F(9,52) = 4.07, p < .001. There were no significant effects of group [F(1,60) = .01, p = .94] or group × time interval interaction [F(9,52) = 1.09, p = .39].
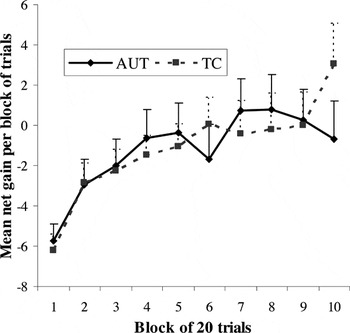
Net gain across trial blocks for the Gambling Task: main effect for trial block (net gain per block increases over time) for both groups; no main effect for group or group × trial block interaction. Error bars represent 1 standard error of the mean. AUT = autism group; TC = typical comparison group.
Reaction time data (seen in Figure 5) indicated that both groups showed faster performance as the task progressed, with both groups appearing to stabilize their response times by approximately the fourth block of trials (see Figure 5). Change in response time across the 10 blocks was statistically significant [F(9,52) = 7.64, p < .001], and there was a significant effect of group [AUT > TC; F(1,60) = 5.75, p < .05]. There was again no group × block interaction [F(9,52) = 1.21, p = .31]. Analysis of choice strategy demonstrated that both groups favored the same strategy of reduced frequency of punishment and a small but steady advantage in reward value, as opposed to the doors containing large but infrequent losses and gains [F(9,52) = 5.47, p < .001]; there were no significant group or group × choice type interactions. Although this version of the Gambling task was modified to be appropriate for younger or more impaired samples, neither the AUT nor TC group demonstrated ceiling effects for this study.
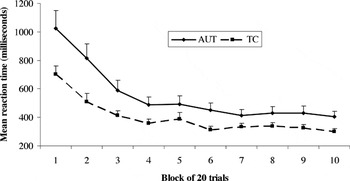
Decrease in mean reaction time for the Gambling Task across trial blocks. Significant main effects for trial block and for group; no group × trial block interaction. Error bars represent 1 standard error of the mean. AUT = autism group; TC = typical comparison group.
Effects of Age
Due to concerns about the heterogeneity of age in our sample, we reanalyzed our data after dividing the sample into older (19 and older) and younger groups. There were no meaningful changes in results or interpretations. Effects were identical to the combined analyses already reported, with two exceptions: the Gambling task net choices variable did not show a significant main effect across trial blocks for the younger group [F(9,16) = 1.67, p = 1.18); and the main effect of emotion condition for Words response time was not significant in the older group [F(2,37) = 1.35, p = .27].
DISCUSSION
This study found what appears to be intact performance on four separate tasks that are known to depend in part on amygdala function, in a sample of 37 participants diagnosed with high-functioning autism relative to age- and IQ-matched typically developing controls. Our results suggest that there may be aspects of emotion processing, known to involve amygdala function, that remain intact in higher functioning individuals with autism. In contrast to the majority of previous studies of amygdala function in autism, these tasks had little overt social relevance, suggesting that amygdala dysfunction in autism may be specific to social information. In light of these and other recent, related findings (e.g., Bernier et al., 2005; Johnson et al., 2006), it is clear that future research will need to explicate specific processes and pathways of amygdala development in autism to understand the contribution of amygdala-based mechanisms to the primary social dysfunction that is the hallmark of autism.
The present study had several limitations. We did not use a task that was explicitly and exclusively based on social perception; findings of a crossover interaction between social and nonsocial tasks in future studies would greatly strengthen our conclusions. Although our analyses did not find significant effects due to age, the wide age range in our autism sample (11–33 years of age) remains a concern: it is possible that some participants with autism in our study, especially given their relatively high cognitive functioning, had developed compensatory strategies to overcome earlier amygdala dysfunction. Research with autism samples from a lower range of cognitive functioning would help clarify whether aspects of amygdala function are intact in all individuals on the spectrum or only those who are higher functioning. Although the AUT sample in this study generally showed substantial symptom severity, there was considerable variability and some individuals demonstrated mild autism symptoms (see Table 2). All IQ scores were above the mental retardation range, which significantly narrows generalizability across the autism spectrum.
Specificity of Amygdala Pathways
The amygdala is a complex structure of 13+ nuclei that share many similarities in structure and function, but also are involved in numerous distinct pathways throughout the brain. LeDoux and colleagues (e.g., Sotres-Bayon et al., 2004) have described a circuit for classic fear conditioning that depends on the association of incoming sensory stimuli that arrive in close temporal proximity in the lateral nucleus of the amygdala, and sequential activation of the fear response through the central nucleus. Sotres-Bayon et al. demonstrated that this pathway does not involve the basal nucleus, which lies adjacent to the lateral and central nuclei. However, amygdala pathways involved in rapid detection and interpretation of facial expressions, which also depend on sensory inputs to the lateral nucleus, provide very rapid feedback to ventral visual pathways in the temporal lobe that leave the amygdala through the basal nucleus (Vuilleumier & Pourtois, 2007; see also Amaral, 2002). This finding opens the possibility, although more exact mechanisms have yet to be defined, that the basal nucleus is particularly important in social information processing. Balleine and Killcross (2006) posit that the lateral and central nuclei work in parallel in the case of appetitive conditioning, each encoding separate aspects of sensory reward and motivation. This explanation could likewise account for impairment in some but not all aspects of associative learning, rather than a more comprehensive deficit that has been previously proposed for autism (Schultz, 2005; Waterhouse et al., 1996). Alternatively, Amaral and colleagues (Amaral & Corbett, 2003) propose that symptoms of abnormal fears and increased anxiety are independent of social impairment in autism, and that clinical manifestations of atypical amygdala function in autism are limited to comorbid (but not causally related) anxiety.
In light of current technical limitations on the ability to effectively image specific nuclei of the amygdala in humans, behavioral experiments using both humans and animals may be the best available method of differentiating specific amygdala circuits and their functionality in people with autism. For example, Adolphs et al. (2005) have proposed that the amygdala specifically contributes to the recognition of fearful facial expressions by directing attention to the eye region. Davidson and colleagues (Dalton et al., 2005) report that gaze fixation on the eye regions while viewing faces is positively correlated with fMRI activation of the amygdala and fusiform gyrus in one autism sample. Dalton et al. further suggest that eye gaze in some individuals with autism leads to heightened amygdala activation, perhaps indicating increased fear or anxiety in social situations. Such interactions between behavioral and neuroimaging studies of basic amygdala functions (for instance, classic fear conditioning) are sorely needed in the field.
There is a similar need to increase the specificity of “amygdala phenotypes” across different forms of psychopathology. Similar circuits are implicated in other disorders of affective regulation, including deficits in memory for fearful faces in children and adolescents with major depressive disorder; as well as associations between response to threat and amygdala activation in fMRI, which may be linked to anxiety disorders (reviewed in Pine et al., 2004). Associations between amygdala function and/or activation have been documented for psychopathy, bipolar disorder, substance abuse, schizophrenia, and posttraumatic stress disorder. Contrasting the nature of different amygdala phenotypes at both the biological and behavioral level will necessarily involve multiple methods using both human and animal models (Nelson et al., 2002). Increased specificity of behavioral and neuroimaging markers may in turn lead to the identification of genetic markers that correspond directly to subnuclei of the amygdala (Zirlinger & Anderson, 2003).
It is also important to consider whether amygdala capacity or function may initially be intact, then becomes compromised as a secondary effect of dysfunction elsewhere in the social perceptual system. For instance, if an abnormality in the white matter pathways between the fusiform face area and the limbic system prevented vital social perceptual information from reaching the amygdala, the amygdala would not be able to provide salient feedback to other ventromedial/orbitofrontal systems that are essential to the evaluation and response to social information.
Developmental Factors
There is a great need to better understand developmental influences on emotion processing in typical and developmentally abnormal populations. The amygdala grows significantly in structure and function through childhood and adolescence (Giedd et al., 1996; Killgore & Yurgelun-Todd, 2004). The lower age range for this study was limited by the nature of the tasks, and future research in this area would benefit from designs and samples that allow for more precise attention to age-based characteristics and, in particular, to younger children. Future studies would also benefit from reaction time control tasks that parse the effects of cognitive processing speed and motor speed.
Perception and Motivation
It is often difficult to ascertain whether atypical levels of motivation in autism are a primary feature of the condition or are secondary to the repeated failures that arise from the cognitive, language, and social deficits of the syndrome. Our current autism sample did not demonstrate insensitivity to reward: it appears they were able to appreciate immediate feedback for a choice and use that feedback to influence upcoming decisions. Future studies may rely on more sophisticated manipulations of reward and punishment contingencies to elucidate motivational pathways in autism. Future designs may also incorporate different categories of nonsocial emotions, including variations in type (e.g., fear, disgust, and pleasure) as well as gradations in valence and arousal.
All of the tasks used in this study are adaptable for both psychophysiological and neuroimaging studies that would provide more direct measures of amygdala and other regional activation. Such measures would be especially useful at separating differences in emotional response from possible global impairments in motor speed or generalized processing problems.
Although the AUT group was significantly slower on several tasks, there were no interactions between group and emotion condition on response time variables, and we do not believe that the between-groups differences are a function of differences in emotion processing. Nonetheless, measures such as skin conductance response or fMRI activation to fearful targets would provide more evidence regarding amygdala involvement in these tasks.
Conclusion
This report highlights the challenge of modeling neural mechanisms through which selective impairments may arise, despite intact functioning elsewhere in the same systems. Developmental influences on emotion and learning is an essential area of study in research on autism. As research regarding emotion processing in autism progresses, it will become increasingly important to improve the specificity of models regarding the similarities and differences in amygdala development and function across syndromes and subtypes of developmental psychopathology.
ACKNOWLEDGMENTS
This work was supported by an NIMH National Research Service Award (F-31 MH 12566) and a University of Utah Marriner S. Eccles Graduate Fellowship in Political Economy to Mikle South; and by an NICHD program project grant (PO1 HD 35476) to William M. McMahon and Janet E. Lainhart, as part of the NICHD/NIDCD Collaborative Network on the Neurobiology and Genetics of Autism. We thank Nicanor Garcia for assistance with data collection; Hilary Coon, PhD, for statistical consultation; and Sherri Provencal, PhD, and Judith Miller, PhD, for assistance with assessment and project design. We especially thank the participants and their families for their continued dedication to research on autism.