Introduction and Motivation
Analyses of ice-core particulates, trapped atmospheric gases and oxygen and hydrogen stable-isotope ratios offer a means to reconstruct climate characteristics and variability over hundreds of thousands of years. These analyses provide paleoclimatic records of ice accumulation rates, temperature, aridity, dust and volcanic sources as well as greenhouse gas concentrations (e.g. Reference Legrand, Lorius, Barkov and PetrovLegrand and others, 1988; Reference PetitPetit and others, 1999; Reference Dunbar, Zielinski and VoisinDunbar and others, 2003). Collecting multiple ice cores over a spatial array allows differentiation of regional weather patterns from global climate signals and ultimately improves our understanding of Earth’s climate history (Reference Dixon, Mayewski, Kaspari, Sneed and HandleyDixon and others, 2004; Reference SteigSteig and others, 2005, Reference Steig2013; Reference Frey, Bales and McConnellFrey and others, 2006). Creating a spatial array of 40 ka old global ice-core records is a central aim of the international ice-core community in order to further understand glacial–interglacial climate change response and processes (Reference Brook and WolffBrook and Wolff, 2006).
More than thirteen deep ice cores have been recovered from Antarctica to date, including seven sites in East Antarctica (Dronning Maud Land, Vostok, Law Dome, Taylor Dome, Dome C, Dome F and Talos Dome) and six in West Antarctica (Byrd Station, Siple Dome, WAIS Divide, Roosevelt Island, Berkner Island and Fletcher Promontory) (Fig. 1). A number of shallow cores have been obtained and analyzed from South Pole (e.g. Reference Mosley-Thompson and ThompsonMosley-Thompson and Thompson, 1982; Reference Kirchner and DelmasKirchner and Delmas, 1988; Reference Saltzman, Aydin, Tatum and WilliamsSaltzman and others, 2008) as well as in surrounding areas through the International Trans-Antarctic Scientific Expedition (ITASE) program (e.g. at Hercules Dome; Reference Jacobel, Welch, Steig and SchneiderJacobel and others, 2005). However, no record longer than 2 ka has been obtained south of 82° S. A new 1500 m ice core will be recovered near the South Pole during the 2014/15 and 2015/16 field seasons, providing the first environmental and climate record of the past 40 ka from this sector of East Antarctica. This paper presents the logistic requirements and glaciologic factors used in selecting the South Pole drill site location.

Fig. 1. Map of Antarctica with deep ice-core locations labeled: South Pole (SP, to be drilled), Berkner Island (BI), Byrd (BY), EPICA Dome C (DC), Dome Fuji (DF), Dronning Maud Land (DML), Fletcher Promontory (FP), Law Dome (LD), Roosevelt Island (RI), Siple Dome A (SD), Talos Dome (TA), Taylor Dome (TR), Vostok (V), WAIS Divide (WD).
Among the motivations for recovering an ice core from the South Pole are:
-
1. The relatively high accumulation rates at the South Pole offer the potential to obtain the highest-yet temporal resolution climate record from the interior of East Antarctica.
-
2. The cold temperatures and low impurity concentrations at the South Pole better preserve atmospheric trace gases (e.g. carbon monoxide, carbonyl sulfide, methyl chloride) than other ice-core sites.
-
3. South Pole is in a climatically distinct region that is influenced both by the conditions of the high East Antarctic plateau and by weather systems that traverse the West Antarctic ice sheet and the Filchner–Ronne and Ross Ice Shelves.
-
4. The proximity of the drill site to South Pole Station reduces the logistic footprint and cost, providing an efficient opportunity to demonstrate the capability of the newly developed US intermediate-depth drill.
-
5. The 1500 m deep, 40 ka old ice core will record climate oscillations from glacial to interglacial periods, allowing study of climate forcing and response.
South Pole Drill Site Logistics and Drill Description
There has been ongoing scientific research at the South Pole since the Amundsen-Scott South Pole Station (South Pole Station) was established during the International Geophysical Year in 1957. Located on the polar plateau, with an elevation of ∼2835 m.a.s.l., South Pole Station resides within an Antarctic Specially Managed Area (ASMA), specifically ASMA 5 (Antarctic Treaty, 2007). An ASMA is internationally managed and regulates human activities to provide scientific, environmental and historical protection and preservation (Conference on Antarctica, 1959).
South Pole Station remains a major hub of science and logistic activities for the United States Antarctic Program. As such, the ice-core site selection was driven by the following scientific and logistic constraints: location within 5 km travel distance of South Pole Station, avoidance of old station structures/roadways, avoidance of the clean-air and seismic sectors to minimize impact on ongoing science in those areas, and minimal influence from current and former station emissions stored in near-surface ice. These constraints focused our site selection activities grid westward of South Pole Station (Fig. 2). The ice-core site ultimately selected is at 89.99° S, 98.16° W (or grid N 48 800, E 42 600) ∼2.7km from South Pole Station (Fig. 2; Table 1). Other projects currently operating in this area include IceCube South Pole Neutrino Observatory (http://icecube.wisc.edu/; Reference KarleKarle and others, 2003) and the Askaryan Radio Array (ARA) neutrino detector (http://ara.wipac.wisc.edu/; Reference AllisonAllison and others, 2012).

Fig. 2. Map of drill-site location relative to dark, clean-air, quiet and downwind sectors, existing firn- and ice-core studies, ice flow velocity and prevailing wind direction (based on Antarctic Treaty (2007) map updated May 2011, modified with drill site, previous core positions). The drill site is ∼2.7km travel distance from South Pole Station. Previous firn- (green) and ice (red)-core retrieval locations are marked on the map, described by reference publications as follows: EMT core (Reference Mosley-ThompsonMosley-Thompson, 1980), Gow core (Reference KuivinenKuivinen, 1983) and 2002 firn core (Reference Aydin, Williams, Tatum and SaltzmanAydin and others, 2008).
Table 1. South Pole drill site location and glaciologic characteristics

A new US intermediate-depth drill (IDD) has been developed by Ice Drilling Design and Operations at the University of Wisconsin-Madison (Reference Johnson, Shturmakov, Kuhl, Mortensen and GibsonJohnson and others, 2014). The IDD builds upon the heritage of the Hans Tausen (Reference JohnsenJohnsen and others, 2007) and Danish deep drill (Reference RandRand, 1980) designs. The electromechanical IDD collects 98mm diameter ice cores in 2 m lengths to a total depth of 1500 m (see drill specifications in Table 2). The South Pole ice core will be retrieved using ESTISOL™ 140 drilling fluid. Recovery of the first ∼700 m of ice core is planned for the austral summer 2014/15 season, and recovery of the following 800 m of ice is planned for the austral summer 2015/16 season. The resulting ice-core record will span ∼40 ka, meeting the International Partnerships in Ice Core Sciences (IPICS) consensus on temporal length for polar ice cores to study climate evolution and climate change mechanisms (Reference Brook and WolffBrook and Wolff, 2006). The following sections summarize the climatology and glaciology of the South Pole area, and discuss the major factors that led to selection of the drilling site.
Table 2. Drill specifications of the new intermediate-depth drill to be used to recover the new South Pole ice core (IDDO, 2013; Reference Johnson, Shturmakov, Kuhl, Mortensen and GibsonJohnson and others, 2014)

Climate of South Pole
The interior of East Antarctica is a climatically distinct region of Antarctica that is yet unsampled by deep ice coring (Fig. 1). The climate in the South Pole region is characterized by a combination of influences. West Antarctica is among the most rapidly warming regions on Earth (Reference Steig, Schneider, Rutherford, Mann, Comiso and ShindellSteig and others, 2009; Reference BromwichBromwich and others, 2013; Reference Steig and OrsiSteig and Orsi, 2013). An influx of heat- and moisture-bearing storms from the Atlantic Weddell Sea and Pacific Ross Sea have been mapped reaching the South Pole from the northwest and southwest, respectively (e.g. Reference HarrisHarris, 1992; Reference Nicolas and BromwichNicolas and Bromwich, 2011). The South Pole more commonly has dry continental, katabatic winds from higher on the plateau, with a prevailing wind direction from 45° E, moving grid southwest across the region. While other East Antarctic plateau sites occasionally experience cyclonic events (e.g. Reference Hirasawa, Nakamura and YamanouchiHirasawa and others, 2000), such events are more common (though still comparatively rare) at South Pole. An example of such an event is illustrated in Figure 3.

Fig. 3. An example of the influence of weather systems which impact both West Antarctica and the South Pole. The figure compares (a) an infrared satellite image from Reference Nicolas and BromwichNicholas and Bromwich (2011) and (b) an Antarctic Mesoscale Prediction System (AMPS) weather forecast of precipitation rate on the same day, 5 August 2006. Both images illustrate the penetration of moisture-bearing systems across West Antarctica, all the way to the South Pole.
The South Pole receives a relatively high annual precipitation of 20 cm of snow, equating to ∼8cmw.e. a−1 (Reference Mosley-Thompson, Paskievitch, Gow and ThompsonMosley-Thompson and others, 1999). Based on the European Centre for Medium-Range Weather Forecasts (ECMWF) reanalysis data, we estimate that ∼50% of the snow accumulation at the South Pole originates in storms such as illustrated in Figure 3, and about an equal amount in the form of clear-sky, ‘diamond dust’ precipitation (see, e.g., Reference Radok and LileRadok and Lille, 1977). The South Pole mean annual air temperature is −49°C, based on a 50 year surface climatology (Reference Lazzara, Keller, Markle and GallagherLazzara and others, 2012) with an average 15 m depth firn temperature of –51°C (recorded in 1998) (Reference Severinghaus, Grachev and BattleSeveringhaus and others, 2001). Annual average wind speed at South Pole Station ranges from 2 to 5ms−1, and monthly maximum wind speeds can reach 20–25m s−1 (Reference Lazzara, Keller, Markle and GallagherLazzara and others, 2012). Winds in the region result in considerable blowing snow, where atmospheric thickness of the blowing snow profile can range from 400 to 1000m off the surface (Reference Mahesh, Eager, Campbell and SpinhirneMahesh and others, 2003).
In comparison to other deep ice-core sites, the South Pole has a high accumulation compared to its cold surface temperature. The EPICA Dronning Maud Land core site is both warmer (−44°C vs South Pole −49°C) and receives less accumulation (6.4 cm w.e. a−1 vs South Pole 8cm w.e. a−1; Reference OerterOerter and others, 2000; EPICA Community Members, 2006). The Talos Dome core site has a similar accumulation rate to that of South Pole but is significantly warmer (−41°C; Reference StenniStenni and others, 2002). South Pole has a substantially higher accumulation rate than East Antarctic plateau sites Dome C, Vostok and Dome Fuji, which receive between 1.4 and 2.5 cm w.e. a−1 (EPICA Community Members, 2006).
The low temperature and relatively high accumulation rate at the South Pole preserve atmospheric gases well. The low impurity content will also be advantageous with respect to post-depositional alteration of gases due to in situ chemical reactions, which has been observed at other locations (Reference Aydin, Williams, Tatum and SaltzmanAydin and others, 2008). Prior analyses of trace gases that yield information on climate and atmospheric composition have proven successful around the South Pole (e.g. carbonyl sulfide; Reference Aydin, Williams, Tatum and SaltzmanAydin and others, 2008). The upcoming South Pole measurements are expected to place in a longer-term context recent decreases in fossil fuel emissions of ethane and methane (Reference AydinAydin and others, 2011) and may also provide further understanding of unexpectedly high levels of nitric oxide that have been documented around the South Pole in several recent studies (e.g. Reference DavisDavis and others, 2001; Reference Neff, Helmig, Grachev and DavisNeff and others, 2008).
Surface temperature records from multiple areas in the interior East Antarctic, including South Pole, show slight climate cooling over the past 30–50 years (Reference DoranDoran and others, 2002; Reference Thompson and SolomonThompson and Solomon, 2002; Reference TurnerTurner and others, 2005). Another slight cooling trend has been recorded at coastal East Antarctic Halley Station near the Weddell Sea (75°58’ S, 26°65’ W; maintained by the British Antarctic Survey since January 1956) (Reference TurnerTurner and others, 2005). Halley and South Pole Stations both experience occasional wintertime blocking highs that develop over East Antarctica and correspond to low-pressure anomalies over the South Atlantic sector (east of the Weddell Sea). These events bring moisture and heat to Halley Station and up the slope to the South Pole (Reference Hirasawa, Nakamura and YamanouchiHirasawa and others, 2000; Reference Schneider, Steig and ComisoSchneider and others, 2004). Analyses from ice cores across West Antarctica show that the occurrence of anomalous atmospheric circulation related to tropical Pacific climate variability (e.g. El Niño events) is strongly recorded in the stable-isotope ratios in precipitation (Reference Schneider and SteigSchneider and Steig, 2008; Reference SteigSteig and others, 2013). Reference Legrand and Feniet-SaigneLegrand and Feniet-Saigne (1991) and Reference Meyerson, Mayewski, Kreutz, Meeker, Whitlow and TwicklerMeyerson and others (2002) suggested that variations in the chemistry of a core from South Pole also reflect El Niño events. Analysis of the long-term stable-isotope and chemistry records from the new South Pole core can thus be expected to further elucidate differences between East and West Antarctic climate in relation to larger-scale climate variability.
Glaciology of the South Pole Region
Accumulation and topography
South Pole Station has provided a base for dozens of glaciological studies over the past 50 years, providing a wealth of data not generally available at the outset of a major ice-coring effort. Here we summarize the key measurements that informed our ice-core site selection.
The accumulation rate of snow has been measured in great detail at South Pole. Accumulation rates measured in the late 1950s to early 1960s were 5–7 cm w.e. a−1 over the previous 10–200 years (Reference Giovinetto and SchwerdtfegerGiovinetto and Schwerdtfeger, 1965; Reference GowGow 1965). Two firn cores ∼10 0m in depth with a 900 year record were retrieved in 1974 (EMT core (Reference Mosley-ThompsonMosley-Thompson, 1980); Fig. 2) and 1982 (Gow core (Reference KuivinenKuivinen, 1983); Fig. 2). Studies detailed in Reference Van der Veen, Mosley-Thompson, Gow and MarkVan der Veen and others (1999) found annual snow accumulations of 5–8 cm w.e. a−1 using these cores. More recent snow accumulation studies have revealed mean accumulation of 10.3 cm w.e. a−1 over a small 50-stake network (5–10 m pole spacing, 50 m × 60 m area) located 400 m grid east from South Pole Station during a 7year period (1988–95; Reference McConnell, Bales and DavisMcConnell and others, 1997) and of 8.5 cm w.e. a−1 over a larger 236-stake network (500 m pole spacing, 10 km hexagon area) located 500 m from South Pole Station during a 5 year period (1992–97; Reference Mosley-Thompson, Paskievitch, Gow and ThompsonMosley-Thompson and others, 1999).
The South Pole is beyond the geographic limit of many satellite-based observations (e.g. Ice, Cloud and land Elevation Satellite (ICESat), 86°S; Special Sensor Microwave/Imager (SSM/I), 87°S; CryoSat-2, 88° S). Some airborne and ground-based radar surveys provide segments of ice stratigraphy data in the South Pole area (Reference Bingham, Siegert, Young and BlankenshipBingham and others, 2007). Ice elevation, ice thickness and bed topography in the South Pole region are estimated in Bedmap2, a compilation of multiple field, airborne and satellite measurements (Reference FretwellFretwell and others, 2013). The Bedmap2 South Pole surface elevations are derived primarily from Reference Bamber, Gomez-Dans and GriggsBamber and others (2009). The digital elevation model from Reference Bamber, Gomez-Dans and GriggsBamber and others (2009) has an elevation uncertainty south of 86° S of 77 m, although even this uncertainty cannot be rigorously quantified (Reference Griggs and BamberGriggs and Bamber, 2009). In a field study, Reference HamiltonHamilton (2004) measured meter-scale surface topography in an 8km × 10km grid upstream of South Pole Station using kinematic GPS. Results from this study demonstrated significant variability in snow accumulation rates due to the interaction of prevailing winds with meter-scale surface topography; for example, a concave depression can receive up to 18% more accumulation than adjacent steeper locations. Overall, surface topography in the larger South Pole area is largely unknown outside of the few traverses, including the US-ITASE 2002, 2003 and 2007 routes (e.g. Reference DixonDixon and others, 2013).
Ice flow and velocity
The South Pole is not on or near an ice divide unlike many previous deep ice cores. Due to its location far from an ice divide, the South Pole core site is best described as a flank site. Ice cores drilled at an ice divide generally contain ice that has had relatively little lateral transport since deposition at or near the coring site. At South Pole, the ice at depth may originate hundreds of kilometers away. The catchment upstream of South Pole spans from Titan Dome (88.50° S, 165.00° E) ∼180 km away to Dome A (81.67° S, 148.82° W) ∼1000km away. Both Support Force Ice Stream (Reference Bamber, Vaughan and JoughinBamber and others, 2000) and Academy Ice Stream (Reference Rignot, Mouginot and ScheuchlRignot and others, 2011) initiate near South Pole.
Uncertainties in the surface velocity field at South Pole are large (Reference Bamber, Gomez-Dans and GriggsBamber and others, 2009; Reference Rignot, Mouginot and ScheuchlRignot and others, 2011). A limited amount of ice surface velocity data at South Pole is available from in situ GPS (e.g. ∼10m a−1; Reference Schenewerk, Mackay, Hothem and ShupeSchenewerk and others, 1994) and regional flow from balance velocities (e.g. ∼10m a−1; Reference Wu and JezekWu and Jezek, 2004). Balance velocities are spatially variable due to the presence of tributaries of Support Force Ice Stream in the vicinity of the South Pole (Reference Le Brocq, Payne and SiegertLe Brocq and others, 2006; Reference Bingham, Siegert, Young and BlankenshipBingham and others, 2007). Reference HamiltonHamilton (2004) measured South Pole ice velocities of 9.6 ma−1 at two locations, 2.5 and 5 km upstream of South Pole, and found ice-flow directions to be 42° W and 36° W, respectively. Modern-day ice velocities measured via GPS average 10.1 ma−1 along 40° W (IceCube Collaboration, 2013). The primary challenge associated with drilling at a flank site is to distinguish the effects of ice advection from a location with a different climate (e.g. Reference Dahl-Jensen, Thorsteinsson, Alley and ShojiDahl-Jensen and others, 1997; Reference Huybrechts, Rybak, Pattyn, Ruth and SteinhageHuybrechts and others, 2007) from the climate changes of interest, and to derive the accumulation rate history from the measured annual-layer thickness (Reference Neumann, Conway, Waddington, Catania and MorseNeumann and others, 2008).
Stratigraphy
Ice thickness and internal layer stratigraphy in the South Pole region have been measured along several transects using airborne radio-echo sounding surveys by the Scott Polar Research Institute-British Antarctic Survey-Technical University of Denmark consortium during the 1970s. A second survey was flown by the University of Texas Institute for Geophysics (UTIG) (Reference Bingham, Siegert, Young and BlankenshipBingham and others, 2007) in 1997/98. Of particular interest are those radar profiles aligned approximately in the along-flow direction over the South Pole, as ice layers in these profiles display continuous layering, with minimal stratigraphic disturbance in the upper 1500 m, and an ice thickness near our core site of ∼2700 m (Fig. 4). The UTIG radar data show some stratigraphic disturbances below ∼2000m. These stratigraphic disturbances in along-flow profiles within ∼10km of our target drilling site are present only at depths well beyond our goal of 1500 m (40 ka).

Fig. 4. Along-flow airborne radar collected by UTIG in 1997/98 over South Pole. The profile displays continuous layering with minimal stratigraphic disturbance in the upper 1500 m. The predominant reflector in the radargram is a reflection from South Pole Station.
The stratigraphy in profiles oriented approximately across-flow is more variable (Fig. 5). There are variations at wavelengths shorter than the bed topography, suggesting either the features were caused by rough bedrock terrain upstream of the radar lines or that there has been irregular flow in the region. Cavitte and others (unpublished information) have mapped the depths of the internal layers, finding that the layers older than 10 ka become significantly deeper to the grid southwest of the South Pole. The relatively sharp change in layer depth across tens of kilometers is unlikely to be the result of spatial variations in the accumulation rate. Cavitte and others suggest that the difference in layer depth is due to a different pattern of ice flow prior to the Holocene. Given the proximity of the South Pole to ice-stream onset regions (Reference Bingham, Siegert, Young and BlankenshipBingham and others, 2007), it is possible that the ice may have been streaming at velocities well in excess of the modern value of 10 ma−1 in the past. This is unlikely to have disturbed the continuous climate record, as discussed in the next section, but it does suggest that determining the provenance of the ice at ages >10ka ago may be difficult without more comprehensive geophysical surveys of the South Pole area, coupled with ice-flow modeling work.
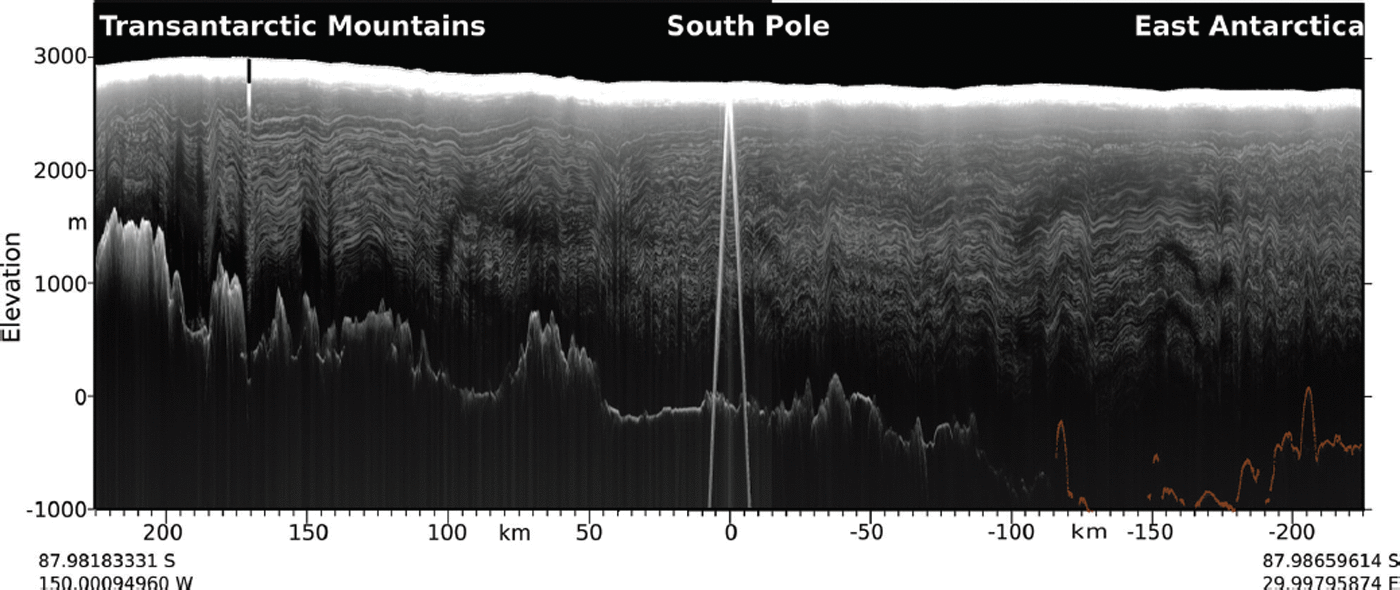
Fig. 5. Across-flow airborne radar collected by UTIG in 1997/98 over South Pole. The profile displays continuous layering with minimal stratigraphic disturbance in the upper 1500 m near the South Pole. The predominant reflector near the center of the radargram is a reflection from South Pole Station.
New high-frequency ground-penetrating radar (GPR) data near the selected drill site were collected in November 2013. The primary motivation for this survey was to assess the potential for buried debris at or near our coring site from the past ∼50 years of station operations. The GPR system used in data acquisition was a Geophysical Survey Systems Inc. (GSSI) SIR 20B GPS system, with a 400 MHz antenna operating at ∼100 kHz pulse repetition frequency. This GPR field survey was conducted in November 2013 in a rectangular survey region approximately 330 m × 330 m, using an integrated GPS system to provide geolocation control of the gridlines. By collecting 31 transects in one direction, and an additional 31 transects in the orthogonal direction, the drill-site GPR survey has just over 10m line spacing (Fig. 6). The GPR data were processed using GSSI’s RADAN software, with the contrast set to accentuate features in the upper 150 ns of two-way travel time (or ∼30m depth). Results indicate smooth and continuous layering, with no obvious disturbances in the upper 15 m on any of the profiles within our target drilling area (Fig. 7).

Fig. 6. Map of the location of the orthogonal GPR survey tracks 19 (acquired north to south) and 49 (acquired east to west) relative to the core site. Each GPR track is 330 m in length. The photo at the lower right shows the drill site as surveyed in November 2013.

Fig. 7. Firn stratigraphy near the selected drill site collected by GPR in November 2013. Survey track 19 was collected from north to south, and perpendicular survey track 49 was collected from east to west. The length of each track is 330 m. Both GPR survey tracks show smooth and continuous layering in the upper 15 m.
Modeling depth-age, annual-layer thicknesses and accumulation rate history
An approximate age–depth relationship for South Pole has been established by comparison with other well-dated ice cores, dating of ash layers imaged by borehole cameras and dust stratigraphy determined by borehole optical logging of the IceCube hot-water holes (Fig. 8a; Reference Price, Woschnagg and ChirkinPrice and others, 2000; Reference Bay, Rohde, Price and BramallBay and others, 2010; IceCube Collaboration, 2013). These data are very unusual to have prior to a major coring effort and are a particularly useful constraint on ice-flow modeling in the area. The depth-age scale has been determined to 2500 m depth and 95 ka by matching laser dust logs between South Pole and EPICA Dome C (IceCube Collaboration, 2013). The upper 1000 m is bubbly ice, so the depth-age relationship was developed by matching five ash layers from the past 25 ka (see IceCube Collaboration, 2013, table 1). Below 1000 m depth, the ice becomes optically clear, allowing a more detailed record of dust variations, and 40 match points with EPICA Dome C between 25 and 95 ka have been identified. These data show the ice is roughly 10 ka old at ∼700 m, and 40 ka old at ∼1500 m. The high degree of correlation of dust layers at South Pole with those at EPICA Dome C shows that although the ice flow history from deposition to recovery in an ice core may be complex, ice flow variations have not compromised the stratigraphic record. Therefore, a continuous climate record should exist at the South Pole.

Fig. 8. (a) The depth–age relationship derived by matching optical dust logs between South Pole and Dome C (ash depth–age data from IceCube Collaboration, 2013). Blue circles are the match points and the thin red line is the modeled depth–age. (b) Average layer thicknesses inferred from depth–age relationship in (a). The red line denotes modeled annual-layer thickness.
The depth–age match points were used to estimate average annual-layer thicknesses (Fig. 8b). Both thinning due to ice flow and lower accumulation rates in the glacial period reduce average annual-layer thickness from ∼10 cm ice eq. at the surface to ∼2 cm at 1500 m depth. From the depth-age relationship and corresponding annual-layer thicknesses, we estimate accumulation rates. We use a one-dimensional (1-D) ice flow model and a nonlinear inverse procedure (Reference Fudge, Waddington, Conway, Lundin and TaylorFudge and others, 2014) to find the smoothest accumulation rate history that fits the depth-age data within a tolerance. The ice-flow model is a Reference Dansgaard and JohnsenDansgaard and Johnsen (1969) model modified following Reference Dahl-Jensen, Gundestrup, Gogineni and MillerDahl-Jensen and others (2003) to account for basal melting and sliding (e.g. Reference Neumann, Conway, Waddington, Catania and MorseNeumann and others, 2008). To find the smoothest accumulation rate history, the ice-flow model requires histories of basal melting, the fraction of surface motion from basal sliding, the ice thickness, and the transition height between zones of constant and linear vertical strain, which dictates the shape of the vertical velocity profile.
We allow the inverse procedure to fit the depth–age data to a tolerance of 150 years, which is similar to the uncertainty in the age relative to the EPICA Dome C timescale (personal communication from R. Bay, 2013). This tolerance allows a smooth accumulation rate history to be found that does not over-fit the data. The smooth accumulation rate history is sufficient in representing the limited depth–age data and unknown upstream spatial variations in ice thickness and accumulation rate. The accumulation rate histories for two different ice-flow parameterizations are shown in Figure 9. These accumulation histories show the general pattern we would expect from other Antarctic accumulation rate histories (e.g. Reference VeresVeres and others, 2013; WAIS Divide Project Members, 2013). The accumulation rate is relatively flat during the Holocene, is about half the modern value during the Last Glacial Maximum (20–28 ka ago), and then a little greater between 30 and 40 ka ago.

Fig. 9. Accumulation rate histories inferred from the depth–age tie points (vertical black lines) using a 1-D ice flow model. Red line uses an approximation appropriate for a flank site frozen to the bed. Black line assumes ice flow is entirely by basal sliding.
Discussion and Conclusions
The existing South Pole region radar profiles and dust layer stratigraphic ties between South Pole and Dome C indicate that the internal stratigraphy at the core site is undisturbed in the upper 1500 m. The newly collected GPR data also show that there has been no significant anthropogenic disturbance at the core site over the past 50 years of South Pole Station operation. Simple ice-flow modeling constrained by the depth–age scale at South Pole produces consistent and plausible accumulation rate histories, further indicating that no significant stratigraphic disturbances have affected the ice-core drill site at the target depths. Therefore, a continuous climate record should exist at the South Pole.
A lack of radar data upstream of the South Pole precludes large-scale flow pattern identification as variations in layer depth due to flow over rough bedrock topography cannot be assessed. Additional measurements of ice thickness, surface elevation, accumulation rate and spatial gradients in snow geochemistry (e.g. stable-isotope ratios and aerosols) upstream of South Pole will be necessary to fully interpret the climate records preserved in the ice.
Ultimately, the upcoming 1500 m South Pole ice core will be recovered using the newly developed US intermediate-depth drill and will provide important climate and environmental records for the past 40 ka. Due to the low temperatures, high accumulation rates and low impurity concentrations at South Pole, the chemistry and trace-gas records are expected to be well preserved in the core. The 1500 m core will provide a high temporal resolution climate history of the connection and confluence of the West Antarctic moisture-laden air masses with the cold dry East Antarctic high-plateau air masses. The core will provide potential to investigate climate sensitivity and identify tropical teleconnections including El Niño signals.
Acknowledgements
This work was supported by US National Science Foundation (NSF) grants 1141839 to E.J.S. and the NASA Cryospheric Sciences Program. We acknowledge the Antarctic Support Associates for collecting the November 2013 GPR data, and Zoe Courville of the US Army Cold Regions Research and Engineering Laboratory for GPR data processing. This is UTIG contribution No. 2789.