1 Introduction
The Laboratory for Intense Lasers (L2I) is a high intensity laser facility hosted by Instituto Superior Técnico at the University of Lisbon. It is dedicated to experimental research in high intensity laser science and technology, ultrashort diagnostics and laser plasma interaction, with emphasis in plasma particle accelerators, high harmonic generation and advanced radiation sources. L2I has been recently selected to a top group of research infrastructures of national strategic relevance, in the scope of which it is undergoing a renovation programme with the goal of enabling external users to benefit from the available and future laser systems.
The main laser of L2I is a 15 TW, hybrid Ti:sapphire–Nd:glass system, based on the chirped pulse amplification technique and operating at 1053 nm. This laser has been serving internal users for several years, e.g., for the development and testing of plasma diagnostics[Reference Boné, Lemos, Figueira and Dias1], guiding schemes for plasma accelerators[Reference Lemos, Grismayer, Cardoso, Geada, Figueira and Dias2–Reference Bendoyro, Onofrei, Sampaio, Macedo, Figueira and Lopes4] and high harmonic generation[Reference Sardinha, Abreu, Dias, Douillet, Figueira, Imran, Kozlova, Lemos, Lopes, Miranda, Rey, Zeitoun and Fajardo5].
Over the past years we have been developing a diode-pumped laser programme, with the goal of improving the experimental capability of the laboratory, namely by improving the repetition rate from the mHz to the Hz rate. We have successfully concluded a dual-stage, diode-pumped amplifier based on Yb:
$\text{CaF}_{2}$
and Yb:YAG operating at 1030 nm and delivering 100 mJ, sub-ps pulses at 1 Hz, and made this laser available for high harmonic generation experiments. Simultaneously this amplifier has been designed to pump an ultrabroadband optical parametric chirped pulse amplifier (OPCPA) based on the nonlinear crystal yttrium calcium oxyborate (YCOB), with the goal of installing a homemade 20 mJ, sub-20 fs laser source. This crystal has been shown to exhibit the required qualities for J-level, few-fs amplification based in OPCPA[Reference Pires, Galimberti and Figueira6–Reference Sun, Ji, Bi, Wang, Kang, Xie and Lin9] and has the potential to become an efficient alternative to current options such as BBO, LBO or (D)KDP for high peak and high average power amplification.
In this paper we describe the current status of the implementation of high-power, ultrashort pulse capability at L2I, in particular evaluating the potential of the ultrabroadband OPCPA system. We start by describing the design and performance of the 100-mJ-level diode-pumped amplifier and the creation of a broadband, low energy signal by white-light continuum generation in bulk media. Next we describe the concept for ultrabroadband noncollinear OPCPA in YCOB and evaluate recent results and perspectives.
2 Diode-pumped amplifier
In the current setup (Figure 1), a Ti:sapphire modelocked oscillator (Coherent Mira 900F
$+$
10 W Verdi) generates an
${\sim}150$
fs modelocked pulse train at 1030 nm with a spectral bandwidth of 24 nm, which is sent through a Faraday isolator to a high contrast 10 Hz pulse picker. The pulse train is sent to a two-stage stretcher, consisting of a [positive group delay dispersion (GDD)] double-pass chirped volume Bragg grating (CVBG) assisted by a (negative GDD) compact diffraction grating pair (DG1 and DG2). The chirp rate of the CVBG is 26 ps nm
$^{-1}$
, which together with the grating pair and taking into account the transmitted bandwidth yields a stretched pulse duration of 330 ps, corresponding to a GDD of
${\sim}30~\text{ps}^{2}$
. The goal of the grating pair is to precompensate the (positive) GDD introduced by the optics inside the regenerative amplifier[Reference João, Pires, Cardoso, Imran and Figueira10].
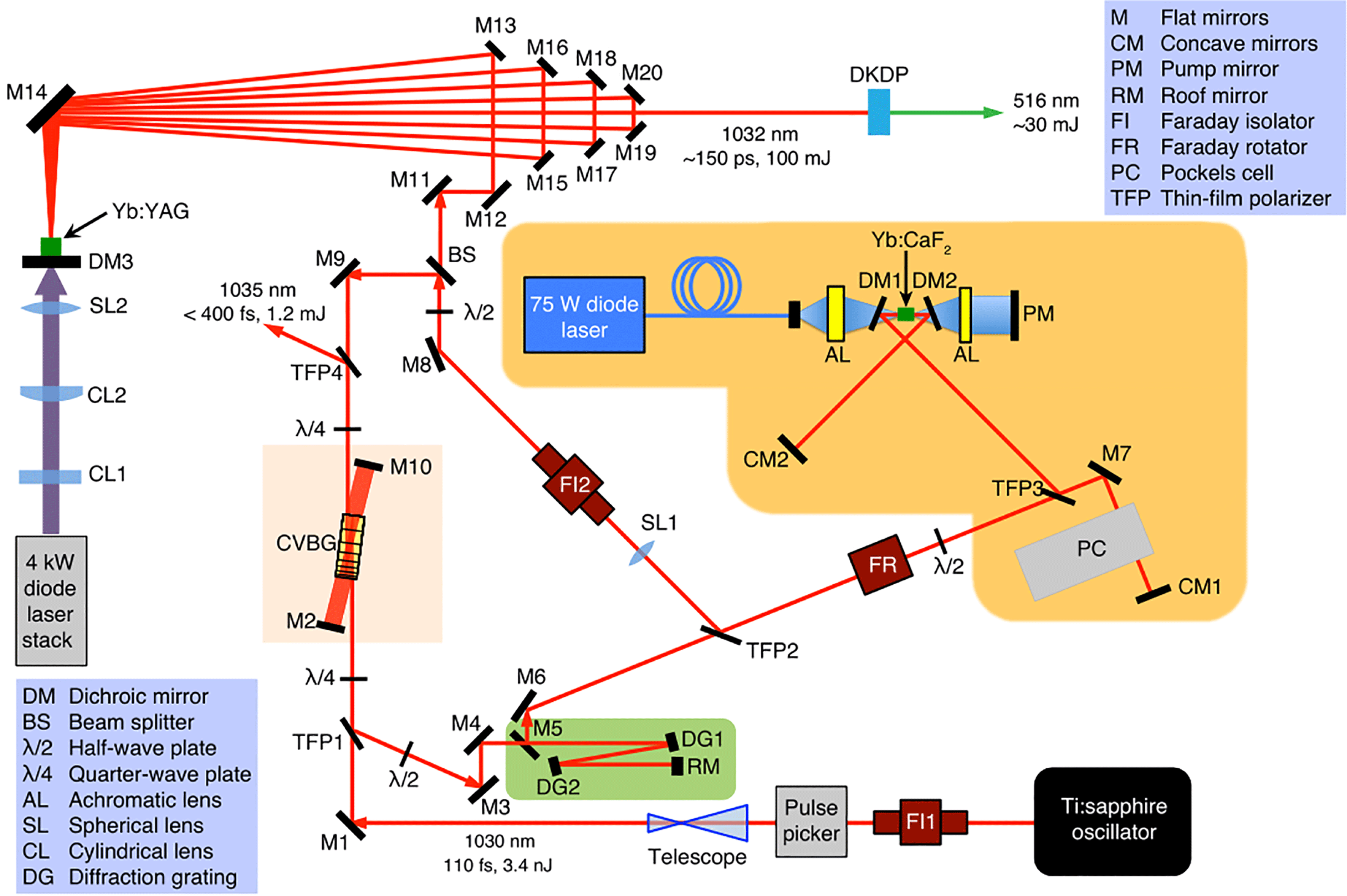
Figure 1. Schematic of the double-stage diode-pumped amplifier, with Yb:CaF2 regenerative amplifier (orange box, right) and Yb:YAG multipass amplifier (left).
The amplification chain consists of a Yb:
$\text{CaF}_{2}$
regenerative amplifier followed by a Yb:YAG 8-pass amplifier, providing a total net gain greater than
$10^{8}$
. The regenerative amplifier consists in a 1.35 m folded cavity bound by two concave mirrors (
$R=500$
and 1000 mm). A 3 at%, 5 mm thick Yb:
$\text{CaF}_{2}$
crystal is placed at the cavity waist (
$250~\unicode[STIX]{x03BC}\text{m}$
diameter) and is surrounded by two dichroic mirrors, transparent to the pump radiation. The pump source is a 75 W, 940 nm fibre-coupled diode laser (Amtron GmBH) set at a pulse width of 3 ms, focused by achromatic lenses into the crystal in a double-pass configuration. Through a combination of a Pockels cell, Faraday rotators and polarizers, the output pulses from this amplifier are sent to the multipass amplifier. This consists in a 9 m long, 8-pass setup, where each pass through the gain medium is ensured by angular multiplexing. An 8 mm long, 15 mm diameter, 3 at% doping level Yb:YAG crystal is placed close to a
$0^{\circ }$
dichroic mirror (DM3), highly reflective around 1030 nm but transparent for the pump radiation. The crystal is pumped by a 4 kW, 940 nm, 25 bars laser diode stack (Jenoptik) with a pulse duration set between 1 and 3 ms. The pump beam is focused on the crystal by a pair of different cylindrical and spherical lenses.
Both the mJ-level output of the regenerative amplifier and the 100-mJ-level output of the multipass amplifier can be compressed and used for experiments. In either case, we use a double passed compressor consisting of a pair or parallel gratings and optimized for cancelling the stretcher parameters.
Concerning the amplifier performance, at a pump power of 55 W the regenerative amplifier generates pulses at 10 Hz with an average energy of 3.1 mJ after achieving saturation, corresponding to around 200 round trips, with an RMS fluctuation below 2%. The output spectrum is slightly shifted towards 1035 nm, with an output bandwidth of 5.7 nm. When using the diode-pumped amplifier as the main laser system for experiments, the full output pulse energy is fed into the multipass amplifier. However, in the OPCPA configuration a fraction (
${\sim}1.2$
mJ) of this output is compressed, either using the same CVBG or the grating compressor, resulting in a compressed pulse duration of 400 fs in the first case and 230 fs in the second. This compressed mJ-level pulse is then focused on a 10 mm thick sapphire block, and through white-light continuum (WLC) generation an ultrabroadband (650–1000 nm) signal for seeding the OPCPA is produced. Results for WLC generated with a similar setup can be found in Refs. [Reference Imran, Hussain and Figueira11, Reference Imran and Figueira12].
The Yb:YAG multipass amplifier is currently capable of delivering a maximum output energy of 106 mJ at 1 Hz, limited by optical damage to the final mirror surface, for a pump power of 1.4 kW. The output spectrum peak is shifted to 1032 nm, with an FWHM spectral bandwidth of
${\sim}4$
nm. When used for experiments, the amplified pulses are sent to the grating pair compressor mentioned earlier, resulting in a compressed pulse duration of 730 fs, with a compressor efficiency of 54%. Pulse spectra were acquired by using a high resolution, broadband spectrometer (Ocean Optics HR2000
$+$
) coupled to an integration sphere (Ocean Optics model FOIS-1). Figure 2 shows the evolution of the spectrum transmitted by the two amplifiers and by the compressor, evidencing the mentioned spectral shift between the amplifiers. This shift can be explained by the different spectral properties of Yb:
$\text{CaF}_{2}$
and Yb:YAG[Reference Koerner, Vorholt, Liebetrau, Kahle, Kloepfel, Seifert, Hein and Kaluza13]. In the first case, the balance between a smoothly decreasing gain cross section and an also decreasing absorption between 1030 and 1040 nm results in an effective amplification peak at
${\sim}1034.5$
nm. When injected with the broadband signal from the oscillator, this central wavelength is preserved (black curve in Figure 2). In the second case, Yb:YAG exhibits a very sharp absorption peak at
${\sim}1032$
nm, coincident with a local, broad absorption region, resulting in a strong, narrowband gain around this wavelength. This leads to the pronounced gain-induced shift and slight gain-induced narrowing (5.7–4.0 nm) that is observed.

Figure 2. Output spectrum from regenerative amplifier (black), multipass amplifier (blue) and grating compressor (red).
Figure 3 shows an image of the beam profile (acquired using an intensity and wavefront meter, Phasics model SID4) evidencing the excellent spatial quality of the amplifier and compressor. In the OPCPA pumping configuration, the uncompressed output is directly frequency doubled in a 15 mm long DKDP crystal, resulting in 40 mJ pulses centred at 516 nm. These are then directed to the double-stage YCOB amplifier, as described next.

Figure 3. Near-field beam profile at the output of the multipass amplifier (left); near (centre) and far (right) beam profile at the grating compressor output.
3 Noncollinear, ultrabroadband OPCPA based on YCOB
In general, a noncollinear configuration for optical parametric amplification allows the broadest phase matching bandwidth[Reference Di Trapani, Andreoni, Solcia, Foggi, Danielius, Dubietis and Piskarskas14]. For these conditions, YCOB has been shown to offer a very large gain bandwidth, in excess of 130 nm[Reference Sun, Ji, Bi, Wang, Kang, Xie and Lin9], which is remarkable even when compared to BBO or LBO. It is also known that for YCOB, being a biaxial crystal, the highest value of the nonlinear coefficient is obtained using a combination of phase matching angles outside of the principal planes[Reference Pires, Galimberti and Figueira6, Reference Chen, Shao, Jiang, Wei, Lin, Wang, Ye, Lv, Wu, Jiang, Yoshimura, Mori and Sasaki15]. Other advantages include its large transparency range and high damage threshold. In addition, recent progress in the growth of YCOB has made available 100 mm aperture boules, showing the great potential of this nonlinear medium for high energy, ultrahigh intensity optical parametric amplification.
We have developed a double-stage noncollinear OPCPA based in YCOB crystals cut with their angles optimized for maximum nonlinear coefficient. Figure 4 shows the schematic of the overall setup. Pulses from the modelocked oscillator are first amplified in the two-stage diode-pumped amplifier described earlier. The output of the regenerative amplifier is split into two arms by using an 80/20 beamsplitter. As mentioned above, the major fraction of the energy is compressed in a CVBG, and focused in a sapphire crystal, leading to white-light continuum generation. A suitable bandpass filter limits the continuum to the range 650–1000 nm, which is used as the seed for the parametric amplifier. The remaining fraction of the regenerative amplifier is sent to the multipass amplifier, amplified to the 100 mJ level and frequency doubled in a KDP crystal. The resulting 50 mJ of green light is then split and used to pump both YCOB crystals.

Figure 4. Schematic of diode-pumped OPCPA system.
We started by developing a 1D numerical model for OPCPA in a general biaxial crystal, and used the parameters for YCOB in order to evaluate the gain and phase matching bandwidth for an amplifier pumped at 515 nm[Reference Pires, Galimberti and Figueira6]. This has resulted in an optimum noncollinear angle of
$3.5^{\circ }$
and optimum phase matching angles
$\unicode[STIX]{x1D703}=115^{\circ }$
and
$\unicode[STIX]{x1D719}=-43.5^{\circ }$
for a 15 mm crystal. For a signal centred at 800 nm, this yields a (continuous FWHM) maximum gain bandwidth of 110 nm.
As mentioned earlier, the seed pulses for the OPCPA are generated by taking the mJ-level output of the regenerative amplifier and generating a WLC in a sapphire block. The great advantage of this approach is that it ensures optical synchronization between pump and signal, and they remain separated only for a distance corresponding to the optical path of the multipass amplifier (
${\sim}9$
m).
The continuum was characterized by a homemade cross correlation frequency resolved optical gating (XFROG) device in order to evaluate the distribution of frequencies over time and the operating zone for amplification and compression. To overcome phase matching limitations, crystal dithering must be used[Reference O’Shea, Kimmel, Gu and Trebino16]. The full spectrum was then retrieved using commercial software, resulting in a usable region extending from 650 to 1000 nm[Reference Imran, Hussain and Figueira11]. One advantage of using a pump at 1030 nm is that the original pulse can be removed by using a suitable high pass filter. One limitation of this method is that the onset of multiple filamentation due to crystal damage may sometimes prevent obtaining a smooth and regular spectrum. Typically the generated white light contains a central white portion where the supercontinuum is stable, and a blue-green outer ring that is removed by an iris.
The WLC is stretched to
${\sim}50$
ps and chirped in a 125 mm long block of SF11 glass. The pulses are then time-delayed in order to account for the optical path of the multipass amplifier and the frequency doubling stage of the pump. We started by comparing the amplification performance for three YCOB crystals (3.5, 7 and 14 mm long) at a
${\sim}3.5^{\circ }$
noncollinear angle, at the first amplifier stage. This first amplifier is run at very high gain, with no considerable saturation or reconversion, due to the very low energy of the signal pulses (
${<}5~\unicode[STIX]{x03BC}\text{J}$
). Since pump depletion was negligible even for the longest crystal, we decided to use the full pump power on the first stage and to recycle it for the second crystal. The maximum output spectral bandwidth obtained for each of the crystals is shown in Figure 5. For each case, a small adjustment of the angles can be made in order to either obtain a narrower continuous bandwidth, or an equally long but interrupted amplification bandwidth. As expected, the longer crystals enable a larger, less modulated bandwidth. Both the 7 and 14 mm long crystals could provide up to 150 nm full width at
$1/e^{2}$
. For the longer crystal it was also possible to amplify a continuous bandwidth encompassing up to 1030 nm by using a slightly larger noncollinear angle. The 3.5 mm crystal could exhibit gain over 100 nm, although this occurred mostly at the peak of the supercontinuum leading to a highly modulated spectrum.

Figure 5. Maximum optimum amplification bandwidth versus YCOB crystal length: 3.5 mm (pink), 7 mm (blue) and 14 mm (grey).
We performed a preliminary evaluation of two-stage amplification, but due to space limitations it was not possible to increase the pump diameter sufficiently in order to avoid optical damage after the first amplification stage. By carefully tuning the phase matching angle of the second OPCPA stage we were able to increase the output bandwidth by slightly detuning the gain away from the pulse central wavelength towards
${\sim}920$
nm (Figure 6). The resulting amplified bandwidth spanned over 225 nm (of which 150 nm were continuous and above the
$1/e^{2}$
level). Such bandwidth is enough to support pulse compression to the few-fs level, well within the goals of generating sub-20 fs pulses. Currently we are working on the implementation of the compression stage, based on transmission gratings, prisms and chirped mirrors.

Figure 6. Gain bandwidth for the first (green) and second (blue) OPCPA stages.
4 Conclusions
We have described the implementation of high energy, ultrashort pulse capability at L2I, following the progress of our diode-pumped programme. We have successfully developed and commissioned a 100 mJ, 1 Hz diode-pumped amplifier based on Yb:
$\text{CaF}_{2}$
and Yb:YAG operating at 1030 nm. This amplifier can work as an independent high-power pulse source, by having the output pulses compressed to the sub-ps level, resulting in
${\sim}0.1$
GW pulses at 1 Hz. Alternatively the output of the amplifier can be frequency doubled and used to pump an optically synchronized, two-stage OPCPA based on the nonlinear crystal YCOB. The tests performed so far have evidenced the ultrabroadband nature of this material, resulting in amplified gain bandwidths in excess of 200 nm, corresponding to few-cycle Fourier transform limited pulse durations. Future work will include the installation and characterization of an ultrabroadband compressor based on a combination of transmission gratings, prisms and specially designed chirped mirrors. Once completed, the new ultrashort laser system will be made available to users.
Acknowledgements
This work is partially supported by Fundação para a Ciência e a Tecnologia, Laserlab-Europe (EC’s FP7, grant agreement no. 284464). This work has been carried out within the framework of the EUROfusion Consortium and has received funding from the Euratom research and training programme 2014–2018 under grant agreement No 633053. The views and opinions expressed herein do not necessarily reflect those of the European Commission.