Introduction
The principal biophysical action of all types of ionising radiation (IR) is to eject outer (Compton effect) or inner (photoelectric effect) shell electrons from atoms or molecules (ionisation), resulting in breakage of chemical bonds. These ionisations can lead to significant damage of cellular compartments such as mitochondria, cell membranes, the endoplasmic reticulum, the Golgi complex, as well as to macromolecules, the most important being nuclear DNA (Refs Reference Somosy1, Reference Tremi2). Multiple types of DNA lesions are formed due to IR. The energy deposited in a cell by 1Gy of radiation produces approximately 105 ionisations or excitations, but only a small fraction (5–10%) of these events will induce permanent molecular damage in a cell nucleus (Ref. Reference Nikjoo3). Biological effects differ based on the linear energy transfer (LET) (energy and type of ionising particle) as well as the cell/tissue type. For instance, the average yield of DNA damage in a typical mammalian cell after 1Gy of high-LET radiation, consists of about 1000 base alterations, 500 single-strand breaks (SSBs), 50–70 double-strand breaks (DSBs), 3 chromosomal aberrations and 2.6 lethal lesions (Refs Reference Nikjoo3, Reference Nikjoo4). As high-LET radiations (such as α-particles and heavy ions) are densely ionising, they deposit large amounts of energy within a small distance along the track of each particle thus producing high levels of dense breaks, ROS and RNS (Ref. Reference Schardt, Elsaesser and Schulz-Ertner5). In general, LET-increase is expected to lead to more DSBs per dose unit and increased DSB complexity (Refs Reference Watanabe, Rahmanian and Nikjoo6, Reference Baiocco7). In fact, the concept of DSB complexity was developed precisely to explain how high-LET IR can be so much more effective, without producing many more (if at all) DSBs as discussed previously in (Refs Reference Hada and Georgakilas8, Reference Georgakilas, O'Neill and Stewart9).
Due to the nature of particle radiation, these lesions are not evenly distributed in a cell nucleus; rather, they ‘cluster’ along the particle track and especially towards the end. This clustering refers to the so-called concept of ‘clustered lesions’ which occur simultaneously at a very close distance of a few nm (Ref. Reference Hada and Georgakilas8). Of all the types of damage, clustered lesions, due to their complexity, constitute the biggest challenge for DNA repair systems. Previous studies on the processing of IR-induced DNA damage, indicated that clustered diverse lesions like DSBs, SSBs and oxidised bases will trigger the activation of several DNA repair pathways. Starting from DSBs, the erroneous non-homologous end joining (NHEJ; canonical and alternative) mechanism is believed to process the majority of DSB lesions. For cells in the S-G2 phase, the accurate and of high-fidelity homologous recombination (HR) will indeed process DSBs. However, recent findings challenge the theory that all DSBs in S-G2 phase are processed by HR and open the door for alternative NHEJ pathways to participate in the case of highly complexed DSBs (Refs Reference Iliakis, Mladenov and Mladenova10, Reference Murmann-Konda11). For all other lesions (SSBs and base lesions) base excision repair (BER) is believed to be the main player. SSB repair (SSBR) is considered a specialised, sub-pathway of BER, processing normal and abnormal strand breaks that arise either from reactions with DNA-damaging agents (endogenous or exogenous) or as intermediates in certain enzymatic events like in the processing of clustered base lesions (Ref. Reference Georgakilas, O'Neill and Stewart9). As extensively discussed in (Ref. Reference Abbotts and Wilson12), SSBR consists of (1) strand break detection; (2) removal of 5′- or 3′-terminal blocking group; (3) gap-filling repair synthesis; and (4) nick-sealing by a DNA ligase. Two pivotal proteins orchestrate SSBR: poly(ADP-ribose) polymerase 1 (PARP1) and X-ray cross-complementing protein 1 (XRCC1) acting rather as scaffolds for the buildup of the various repair components required to mantle the SSB and restore the phosphodiester backbone.
Therefore, high-LET irradiations, even at low doses, can hobble the cellular DNA repair machinery and lead to delayed repair or misrepair, resulting in mutations and complex chromosomal aberrations (Refs Reference Yatagai13, Reference Kawata14). Misrepair is usually the result of lesion proximity or choice of the DNA repair pathway (Ref. Reference Schipler and Iliakis15). For example, the repair of DSBs induced by carbon ions is dependent on resection, a process directly related to the repair pathway selected (Refs Reference Averbeck16, Reference Grabarz17). Misrepair is also believed to play an important role in the increased relative biological effectiveness (RBE) values after high-LET radiation compared to the low-LET one (Ref. Reference Averbeck18).
Particle radiation therapy (RT) as a clinical modality may utilise different particles, such as electrons, protons or carbon ions; doses, such as high- and low- dose brachytherapy; dose rates, such as ultra-high dose (FLASH) RT as well as various conformality modes, such as brachytherapy, external beam RT, intensity-modulated RT and stereotactic body RT. Understanding the major advantages and disadvantages of low and high LET treatment options is of critical importance for the more accurate tumour treatment and the minimisation of toxicity in the patients. The differential level and types of biological damage induced in the tumour versus healthy tissues are of significant clinical importance and therefore, we will focus on the mechanisms of DNA damage induction for the high-LET RT modalities like protons, carbons, α-particle-emitting radionuclides which are highly cytotoxic and are thus promising candidates for use also in targeted radioimmunotherapy of cancer (Refs Reference Nickoloff19, Reference Seidl20).
Overview of medium to high-LET radiotherapies (protons, neutrons, a-particles, carbon ions)
Proton radiation therapy
Proton therapy uses a beam of protons of primarily medium LET which are positively charged particles (essentially hydrogen nuclei) with a very well-defined range of tissue penetration, to deliver radiation to a targeted tumour. Higher-LET values do exist across the track of the proton beam particularly towards the distal edge of the spread-out Bragg peak (SOBP), compared with conventional X-ray radiation (Ref. Reference Vanderwaeren21). This radiation modality allows for a better distribution of the delivered dose of radiation compared to photon (X-Ray) radiotherapy and has led to an expansion of the treatment options in radiation oncology. The SOBP is a combination of several Bragg peaks in such a way, that an entire tumour can be targeted with minimal dose deposition to adjacent normal tissues. The idea to use protons in cancer treatment was conceived by the physicist Robert R. Wilson in 1946 (Ref. Reference Wilson22) and the first patients were treated in 1954 (Ref. Reference Lawrence23). We have now amassed a substantial amount of clinical evidence on the efficacy of proton radiation treatment (Ref. Reference Yuan, Zhan and Qian24). In a recent comparative analysis of protons versus photons in combination with chemotherapeutic regimens, proton chemoradiotherapy presented significantly reduced acute adverse events that were causing unplanned hospitalisations, with similar disease-free and overall survival (Ref. Reference Baumann25).
A new exciting development in the field of radiotherapy is the so-called ‘FLASH’ radiotherapy which has quickly evolved into a highly promising radiation modality. Proton and other particles (such as electrons or carbon ions) are delivered at ultra-high (‘FLASH’) dose rates (currently at >40 Gy/sec of higher) whereas in the clinic, the ‘standard’ dose rates are at approximately 5 Gy/min (Ref. Reference Hughes and Parsons26). FLASH radiotherapy has demonstrated increased sparing of the normal tissue while maintaining an equipotent antitumour efficacy compared to conventional radiotherapy in multiple preclinical studies (Refs Reference Favaudon27–Reference Diffenderfer34). The medium LET that characterises proton radiation, along with the SOBP can enhance the potency of ‘FLASH’ effect during proton radiotherapy (Ref. Reference Hughes and Parsons26). A major advancement in the field of FLASH radiotherapy is the onset of the world's first human clinical trial of FLASH RT by the Cincinnati Children's/UC Health Proton Therapy Center. The study named as FAST-01 (FeAsibility Study of FLASH Radiotherapy for the Treatment of Symptomatic Bone Metastases) (NCT04592887), will be delivering a single radiotherapy session to the recruited cancer patients in a fraction of a second. For a recent analytical review on FLASH radiotherapy and potential biological mechanisms underlying its biological effects the reader is directed to (Ref. Reference Hughes and Parsons26).
Carbon ion therapy
Carbon ion radiotherapy (CIRT) is another form of particle beam therapy which provides a series of physical and biological advantages (Refs Reference Malouff35, Reference Brown and Suit36). The larger mass of carbon allows for even more reduced beam scattering, characterised by a steeper dose distribution area with minimal penumbra and sharper Bragg peak (Refs Reference Rackwitz and Debus37–Reference Durante and Loeffler39) compared to protons, theoretically allowing for better tolerance of radiosensitive organs (Refs Reference Kamada40–Reference Imada42). Moreover, carbons demonstrate higher LET than protons, something which leads to a two to three fold-higher RBE compared to protons and photons (Ref. Reference Raju and Carpenter43) due to the more dense and complex DNA damage (Ref. Reference Mohamad38). Other differences of CIRT compared to other radiotherapies include the lack of oxygen effect, sub-lethal damage repair and the reduced impact of the cell cycle on radiosensitivity (Ref. Reference Ebner and Kamada44). Today, there are 13 CIRT centres in five countries treating tumours, such as prostate, head and neck, lung, liver, bone, soft tissue sarcomas, locally recurrent rectal cancer and pancreatic cancer (Refs Reference Kamada40, Reference Shinoto45). Unfortunately, there is still scarce evidence (e.g. comparative clinical trials) comparing head-head the efficacy and toxicity of CIRT to other particle or photon RT.
Nuclear radiotherapy
The biological effects of external beam Radiotherapy are sufficiently described by the ionisation effects occurring while accelerated ions traverse and interact with the biological matter based on nuclear physics laws. Many decades ago, a combinatorial Radiotherapy modality was proposed, one where in addition to primary ionisations, accelerated particles would also cause, nuclear reactions to atoms of a suitable substrate, thus creating heavier ions (commonly α-particles), which are characterised by higher LET values. In this review, we use the term Nuclear Radiotherapy to distinguish this modality from conventional external beam RT. The adjective ‘Nuclear’ within the term implies the need of nuclear physics to describe the reactions taking place, the possible nuclear energy states of implicated particles as well as their kinetic energy and finally their LET values. High LET ions are expected to cause more severe damage to the targeted cancer cells. In this review, we will focus on proton-boron (fusion/) capture therapy (PBCT) and boron-neutron capture therapy (BNCT) (see below and Supplementary data). Although initially there was a great deal of promise in the use of Nuclear Radiotherapy approaches, these have not fulfilled their promise for reasons we will outline below. A major shortcoming of Nuclear Radiotherapy has been attributed to the limited possibility of a nuclear interaction in practice. Even if an accelerated charged particle has the exact amount of energy to excite the targeted molecule/atom and its trajectory indeed points towards the target, the possibility (cross section) for the nuclear reaction to be triggered is at least 104 times smaller than the corresponding one for the ionisation process, even when high-energy ions or electrons are used as primary particles (Refs Reference Kaganovich46, Reference Tawara and Kato47). In the case of fast neutrons, an elastic and inelastic scattering are in general the dominant mechanisms for energy moderation, since human tissues act as an excellent absorber for neutron capture (n,γ) reactions in the thermal (0.025 eV) and epithermal energy region (0.025–4 eV). Thus the predominant mechanisms for radiation damage and energy deposition are usually not the intended ones via the specific, useful (n, α) nuclear reactions. Additionally, the delivery of adequate amounts of boronated compounds to the tumour while avoiding any significant uptake in normal tissues constitutes one of the most challenging issues for a successful treatment with the BNCT or PBCT techniques (Ref. Reference Malouff48). Thus, there are still many issues open for scientific investigation before the practical clinical stage is reached. Despite the clinical failure of these approaches, they have laid the foundations for a more efficacious approach based on similar principles such as PBCT (see below and in Supplementary data).
Proton–boron capture therapy
PBCT is an innovative method towards increased proton biological effectiveness. PBCT is based on pure nuclear physics principles and uses a nuclear fusion reaction between low-energy protons and 11B atoms, that is



The reaction produces high LET α-particles of enhanced damaging potential ideally solely on the desired target tumour area that is SOBP, without damaging neighbouring normal tissues (Refs Reference Bláha49, Reference Maliszewska-Olejniczak50).
Proton Boron reaction involves the production of 12C in one of its excited (nuclear) states (12C*). For the resonance occurring at 0.675 MeV (projectile energy) (Ref. Reference Ajzenberg-Selove51) there are three main options of de-excitation. Two of them are described by the 2-step reaction ((1) and (2)) and the third by the 1-step reaction (1) (Ref. Reference Stave52). According to Stave et al. the predominant one is the 2-step reaction, which involves the production of a Be nucleus (Ref. Reference Stave52). In order to investigate the α-particles biological effects, exact knowledge of their LET is required. To calculate the LET values we need to know the energy values that they are emitted with and this can be considered as a rather complicated problem. In the following, we describe why this issue is a bit complicated. Figure S1 (Supplementary data) illustrates the phenomenon. The p + 11B → 3α reaction releases an amount of energy Q = 8.59 MeV for example [http://nrv.jinr.ru/nrv/webnrv/qcalc/], which has to be shared (along with a fraction of approx. 11/12 of the incoming proton's kinetic energy) among the three ejectiles, that is the α-particles with varying energies as shown in Table S1 (maximum 231 keV/μm).
BNCT is a radiotherapeutic modality which targets cancer cells via a two-step procedure. During the first stage, the patient is injected with a tumour-localising drug which carries the stable isotope boron-10 (10B) which has a high tendency to capture low energy ‘thermal’ neutrons (n th). The most widely used boron delivery agents are the sodium borocaptate (BSH) and the boronophenylalanine (BPA), while the latter can be associated to fructose to yield BPA-F with enhanced solubility (Ref. Reference Barth, Mi and Yang53). In the second stage, the patient is subjected to irradiation with neutrons and the subsequent nuclear reactions occurring when 10B is hit with neutrons, lead to the emission of high-energy α-particles (4He) and recoiling lithium-7 (7Li) nuclei that kill the cancer cells that have accumulated sufficient quantities of 10B (Refs Reference Barth, Mi and Yang53–Reference Barth55). The basic interaction equations are described below:


Using catkin 2.03 and jinr Q-value_calc [http://nrv.jinr.ru/nrv/webnrv/qcalc/] one can easily determine the levels of the kinetic energy of implicated particles, considering for neutrons Tn = 0.025 eV:

Figure S2 (Supplementary data) illustrates the energy levels implicated in boron-neutron fusion. The major benefit of this approach is the protection it affords to critical normal tissues from radiation-induced damage, even at the margins of the tumour (Ref. Reference Barth, Zhang and Liu54). This supports the use of BNCT as an alternative therapeutic option for the treatment of multiple, locally invasive malignant tumours such as high-grade gliomas (Ref. Reference Miyatake56), recurrent cancers of the head and neck region (Ref. Reference Wang57) and primary tumours or cerebral metastases of melanoma (Ref. Reference Hiratsuka58). Current clinical evidence mostly revolves around the use of non-radioactive 10B. Gadolinium-157 (Gd), another non-radioactive isotope has also been used but the present data have been strictly derived from in vivo studies without further examination of its efficacy in the clinic (Refs Reference De Stasio59–Reference Shih and Brugger61).
There are prerequisites for the ideal boron-containing candidate such as low systemic toxicity, much higher tumour uptake compared to the normal tissue and rapid clearance from normal tissues. Currently, the most successful boron delivery agent, BPA selectively accumulates in tumours through cancer-related amino acid transporters including LAT1 (Ref. Reference Nomoto62). However, this approach also carries disadvantages such as reduced solubility and a short retention time (Ref. Reference Fukuda63). The demand for better tumour targeting properties and a noticeable difference in the rate of uptake of boron-containing agents by different patients, have ushered in the production of several generations of boron-containing agents including boron-containing porphyrins, amino acids, polyamines, nucleosides, peptides, monoclonal antibodies, liposomes, nanoparticles of various types, boron cluster compounds and co-polymers (Refs Reference Barth, Mi and Yang53, Reference Barth55, Reference Farr64–Reference Coderre67). The current list of malignancies for which BNCT has shown at least some clinical benefit is quite long (Ref. Reference Malouff48) and includes glioblastoma multiforme (Refs Reference Miyatake56, Reference Chanana68–Reference Kawabata70), meningioma (Ref. Reference Miyatake71), head and neck cancers (Refs Reference Wang57, Reference Kankaanranta72–Reference Wang76), lung cancer (Ref. Reference Suzuki77), sarcomas (Ref. Reference Futamura78), cutaneous malignancies (Refs Reference Menéndez79–Reference Yong81), extramammary Paget's disease (Ref. Reference Hiratsuka58), paediatric cancers (Refs Reference Nakagawa82–Reference Kageji84) and metastatic disease (Refs Reference Wittig85, Reference Zonta86). These studies underscore the promise of PBCT as a therapeutic strategy, especially for radiotherapy-resistant tumours. Moreover, if coupled with proton FLASH radiotherapy modalities, BPCT could further enhance the proton therapy therapeutic index. (Ref. Reference Bláha49).
Alpha-particle emitter radiopharmaceutical therapy (α-RPT)
Cancer therapy based on α-particle radiopharmaceutical emitters is a relatively new technological advancement and clinical modality with only a few such drugs available in the market for clinical use (Ref. Reference Sgouros87). α-particles emitted from radionuclides have a high LET (>100 keV/μm) which produces a short, highly dense track of ionisation events within cellular targets, leading to repair-resistant complex DNA damage and more effective cell killing compared to sparsely ionising forms of radiation, such as γ- or X-rays. Moreover, based on well-known radiobiology from the 1980s, alpha-emitting radionuclides exhibit a high RBE for cell killing (as high as 8) (Ref. Reference Yard88) accompanied by a significantly reduced dependency on hypoxia which is responsible for solid tumour radioresistance (Ref. Reference Stewart89). Currently, drugs being used in patients or under clinical trials are based on 223Ra and 224Ra as α-particle emitters (Diffusing Alpha Radiation Emitters Therapy; DaRT). A search in NCI clinical.trials.gov on 21/11/2021 using the keywords ‘alpha emitters’ or ‘alpha particle emitter’ or ‘alpha radiation emitter’ retrieved a maximum of 19 studies for treating resistant prostate or breast cancer with metastases as well as skin and soft tissue tumours. Radium-223 dichloride (Xofigo®, Bayer HealthCare Pharmaceuticals Inc.) is the first α-RPT approved by the FDA, with benefits in overall survival and delay in symptomatic skeletal events for patients with metastatic castrate-resistant prostate cancer (CRPC). Recently, an automated bone scan algorithm has shown its value in determining the response to radium-223 (Ra-223) treatment for patients with metastatic CRPC (Ref. Reference Anand90).
DNA Damage (DSB, SSBs) involved in each high-LET modality
Proton Based therapies
Proton therapy is a quickly expanding radiotherapy modality where accelerated proton beams are utilised to accurately transport their maximum of energy and dose to the tumour but is usually ineffective against highly radioresistant tumours. In the case of protons, typical energies used in the clinic regularly range from 60–250 MeV including the case of the latest advances in the field that is, the FLASH method where ultra-high dose rates 40–200 Gy/s can be achieved through the delivery of very short proton ms-pulses (Refs Reference Darafsheh91, Reference Kourkafas92).
Theoretical considerations
In an effort to have a better understanding of the primary molecular effects of high-LET radiations, we have mined accumulated data on the induction rates of double and single-strand breaks (DSBs and SSBs respectively) for a variety of biological systems and both experimental and simulation data (Tables 1–3). Theoretical predictions of biological damage from DNA to cellular survival are always very helpful and have aided significantly the planning of experiments and interpretations of results. By the word of theoretical predictions, we refer to the well-accepted and acknowledged stochastic modelling using Monte Carlo (MC) simulations and particle track structure codes [for recent reviews please see (Refs Reference Nikjoo93, Reference Chatzipapas94)] as well as others non-stochastic using mathematical or machine learning-based calculations (Refs Reference Kalospyros95–Reference Papakonstantinou97). It must be mentioned though that all simulations of any type are optimised based on experimental data. For example, using the Geant4DNA MC code and an improved DNA geometry model as well as clustering algorithms, DNA damage yields were calculated and compared to literature (Ref. Reference Zhao98). The model was tested against published experimental and computational results for DSB rates and RBE values. A general agreement was observed over the whole simulated proton energy range. The DSB yield was 8.0 ± 0.3 DSB Gy−1Gbp−1 for 60Co, and 9.2 ± 0.2 DSB Gy−1Gbp−1for 250 kVp X-rays, and between 11.1 ± 0.9 and 8.1 ± 0.5 DSB Gy−1Gbp−1for 2–100 MeV protons. The specific results suggest that DSBs consist of direct and indirectly-induced (through ROS) SSBs that account approximately for half of the total DSBs (Ref. Reference Zhao98).
Table 1. Protons and electromagnetic irradiation

Table 2. Carbons and electromagnetic radiations

Table 3. α-particles and electromagnetic radiations

Table 1 contains data from in vitro and in silico works using protons and electromagnetic irradiation. Variations among different simulations can be attributed to different initial conditions fed into the algorithm, and especially the concentration of antioxidants, oxygen levels and nuclear DNA density. As a general conclusion, it can be deducted that an increase in LET leads to an increase of DSBs and to a reduction of SSB levels as well as of other non-DSB oxidative damage (Refs Reference Zhao98–Reference Pater101). This of course suggests a higher level of complexity for DSBs at least.
Carbon Ion radiotherapy
CIRT is the treatment of choice for proton-resistant tumours like sarcomas (Ref. Reference Malouff35). Table 2 contains data from in vitro and in silico studies of carbons. Keta et al. (Ref. Reference Keta102) irradiated three malignant cell lines with protons or carbons and they evaluated DNA damage by quantifying γH2AX foci. No significant differences were observed in the γH2AX foci formation between the two radiation modalities and they attributed this result to the limited resolution that ICC offers when performed using conventional fluorescence microscopy. Yokota et al. (Ref. Reference Yokota105) using pulsed-field gel electrophoresis (PFGE) quantified DSBs yields in protoplasts from the tobacco BY-2 cell line. They identified a difference in the induced DSB between γ-rays and carbon ions, but they could not satisfactorily detect a difference in the DSB yields among the several LET values of carbons. Moreover, their approach failed to exhibit expected results that is higher DSBs for the highest LET used. They interpreted this result by the phenomena occurring to the carbons penumbra region and to the possible dosimetry inaccuracies. Back in 1995, Heilmann et al. (Ref. Reference Heilmann, Taucher-Scholz and Kraft106) irradiated CHO-K1 cells with carbons of different energies and assessed DNA damage using constant-field gel electrophoresis. Their results, although not revealing an increased number of DSBs, underline the possible inability of electrophoretic approaches to detect very small DNA fragments as a result of highly complex DSBs, a phenomenon also discussed in (Ref. Reference Hada and Georgakilas8). Shiina et al. (Ref. Reference Shiina107) compared carbons of 60 keV/μm LET and X-rays using agarose gel electrophoresis (AGE), in several Tris scavenger concentrations. Comparing results for minimum (1.0 × 106/s) and maximum (Tris 1.0 × 1010/s) scavenging capacities of Tris. Corresponding values of Tris scavenging capacity that mimics cellular environment, that is 3.0 × 108/s are also presented in Table 2. An increase in scavenging capacity causes a decrease of both DSB and SSB levels, for every radiation modality.
α-particles Produced during nuclear radiotherapy
α-particles are produced during nuclear radiotherapy as well as during brachytherapy. They have short ranges in the body, depending on their emission energy, usually less than 100 μm. Their energy and consequently their LET change dramatically with distance, when traversing tissues or water, which is considered roughly radiologically equivalent to tissue. Table 3 includes studies using plasmid DNA, protoplasts, cells or simulations: Pater et al. (Ref. Reference Pater101) using MC simulations observe similar dependence of DSB and SSB with LET for α-particles and for protons: LET increase leads to DSB increase against SSB levels which decrease. Yokota et al. (Ref. Reference Yokota105) counted DSB yields after exposure to α-particles or γ-rays, by using protoplasts from the tobacco BY-2 cell line and by performing PFGE: LET increase resulted to increase of DSB levels. In another study, by using plasmid pUC18 and AGE, it was found that LET increase leads to an increase of DSB yields but it was not detected to affect the number of SSBs per Gy and per Gbp (Ref. Reference Urushibara110). Prise et al. (Ref. Reference Prise, Pullar and Michael111) compared α-particles and γ-rays irradiation using pNSG-CAT plasmid and AGE at two concentrations of the ROS scavenger Tris. At both concentrations, similar results with (Ref. Reference Pater101) for both scavenging conditions were acquired. A study using V79-379A hamster cells and PFGE shows increased DSB levels for α-irradiation compared to X-rays (Ref. Reference Claesson112). To summarise, all the studies show that the LET increase leads to DSB increase against SSB, at least for moderate LET values.
Overview of DNA repair pathways involved in each high-LET radiotherapy 4.1. The role of DNA repair in high-LET radiotherapy
Cellular damage response pathways are expected to be activated even after the induction of low levels of biological damage in DNA, proteins or lipids (Refs Reference Gorgoulis115, Reference Chatgilialoglu116). Although, we acknowledge here the importance of damage to protein and lipids by IR, we give emphasis to the damage induced to genetic material and specifically, DNA. To mitigate threats on genome integrity posed by genotoxic agents like high-LET radiations, cells have evolved a complex set of repair mechanisms collectively called the DNA Damage Response (DDR) (Ref. Reference Gorgoulis115). DDR coordinates DNA repair, DNA replication, and cell-cycle checkpoint pathways with cell fate, including apoptosis and senescence. Various DNA repair mechanisms are engaged for the processing of DNA damaged sites, especially the repair of DNA DSBs. DNA repair pathways of mammalian cells, include the BER, the nucleotide excision repair (NER), the mismatch repair (MMR) as well as the pathways responsible for the repair of DSBs, which are the HR, the classical non-homologous end joining (c-NHEJ), the backup non-homologous end-joining (B-NHEJ) and single-strand annealing (SSA). The choice of the repair pathway for DNA DSBs primarily depends on radiation quality (Ref. Reference Sridharan117) and possibly on DSB load (Ref. Reference Iliakis, Mladenov and Mladenova10). The induction of DNA lesions of diverse and varying levels of complexity is expected to trigger simultaneously different DNA repair pathways. Currently, it is not yet clear if there is a preference in the choice of repair pathway between low-LET and high-LET radiation (Fig. 1) (Ref. Reference Zhao118).
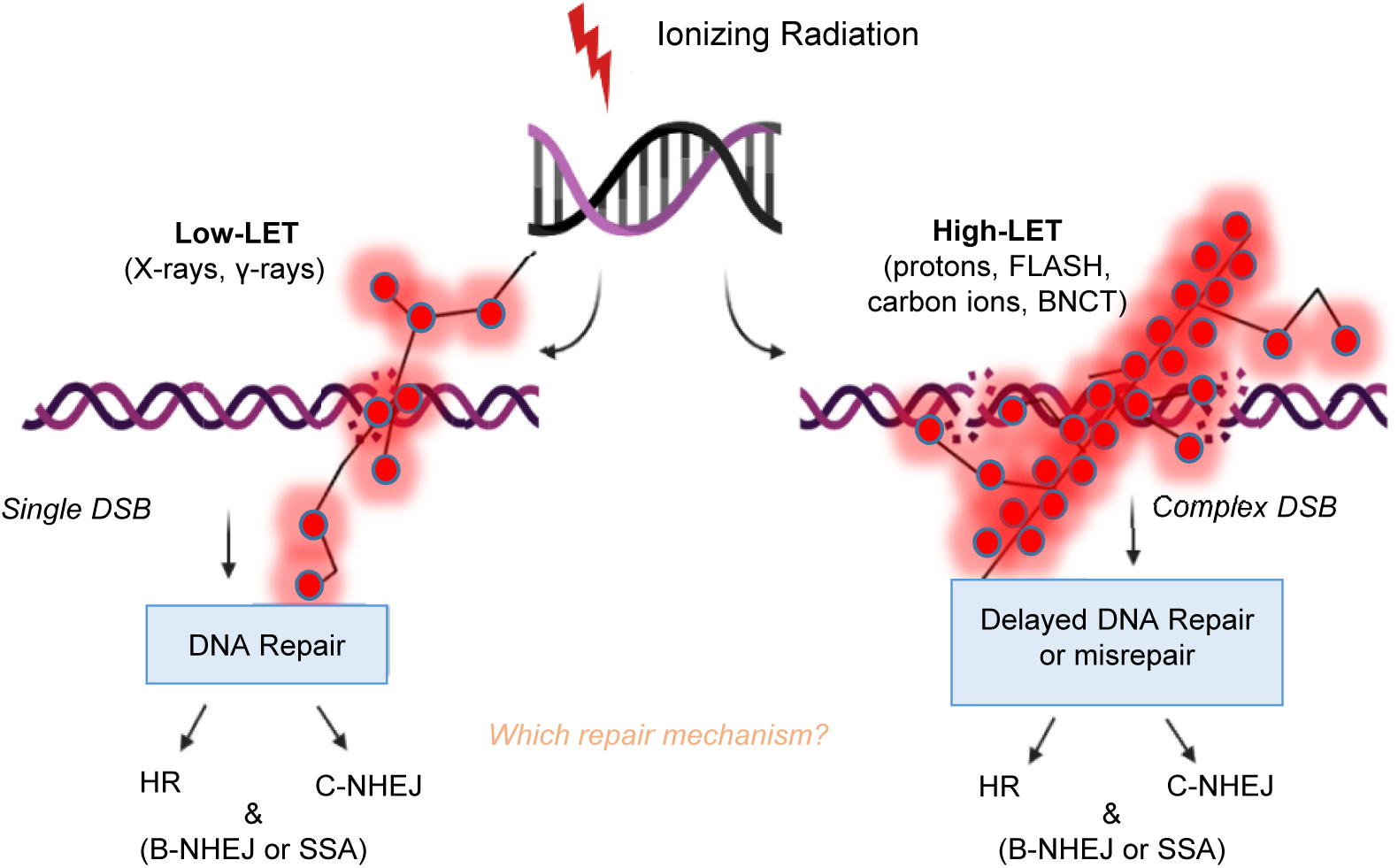
Fig. 1. Double-strand breaks (DSBs) repair pathways after low- and high-LET radiation. Protons maybe considered medium to high-LET only towards the distal end of their track.
In general, isolated DSBs induced by low-LET radiation are mainly repaired by c-NHEJ and HR, while clustered DSBs generated by high-LET radiation are difficult to repair (Ref. Reference Shrivastav, De Haro and Nickoloff119).To examine the effects of radiation on DNA DSB repair and radiosensitivity, Takahashi et al. used embryonic fibroblasts bearing repair gene (NHEJ-related Lig4 and/or HR-related Rad54) knockouts (KO) and showed that c-NHEJ plays a more essential role compared to HR in defining radiosensitivity after high-LET (carbon ions) radiation (Ref. Reference Takahashi120). This implies that c-NHEJ might be the principle repair mechanism used in response to high-LET IR-induced DSBs. Another study from Okayasu et al. showed that the clustered DSBs induced by high-LET (heavy ions) radiation may markedly depend on c-NHEJ (Ref. Reference Okayasu121). Sridharan et al. further supported this hypothesis by showing that Artemis, a key complex of c-NHEJ is involved in the repair of the clustered DSBs induced by high-LET radiation (Ref. Reference Sridharan122). In addition to the c-NHEJ and HR pathways, residual simple and clustered DSBs induced by IR can also be repaired by the pathways of alt-EJ and SSA, but the detailed processes of the two pathways in response to high- and low-LET radiation are still unclear (Ref. Reference Iliakis, Mladenov and Mladenova10). Even though high-LET IR induces DNA damage complexity, DSBs induced by 56Fe+ ions are actively processed, compared to low-LET IR (e.g. X-rays) albeit with a somewhat reduced efficiency (Ref. Reference Singh123). Again, this leads to the conclusion that a significant amount of high-LET IR-induced DSBs is processed by c-NHEJ.
In contrast to the notion that c-NHEJ maybe the preferable pathway for lesions of increased complexity stated above, there are studies that support the idea that the HR pathway may be favoured for the repair of heavily charged particle-induced clustered DNA lesions. For instance, Zafar et al. propose that complex DSBs generated by high LET irradiation from very high-Z particles are repaired by HR and not NHEJ in mammalian cells (Ref. Reference Faria124). Other studies with protons also support this notion (Refs Reference Grosse125, Reference Rostek126), since mammalian cells deficient in HR factors such as Rad51 paralogs are sensitive to heavy particle radiation (Ref. Reference Faria124). Interestingly, most studies that suggest a major role for NHEJ in the repair of DNA damage, are mainly carbon ion-based studies (Ref. Reference Zhou127). Ariungerel et al. suggest that the NHEJ pathway plays an important role in repairing DSBs induced by both clinical proton and carbon ion beams (Ref. Reference Ariungerel128). However, they propose that in the case of carbon ions, the HR pathway appears to be involved in the repair of DSBs to a greater extent compared to gamma rays and protons. Recently, c-NHEJ has also received strong interest in BNCT-induced damage (Refs Reference Mohamad38, Reference Kondo129). Kondo et al. showed that DNA damage induced by BNCT is partially repaired by a key player of the NHEJ pathway, ligase IV (Ref. Reference Kondo129). Another study on human thyroid follicular cancer cell line (WRO) demonstrated that after BNCT, HR is the main activated pathway based on high expression of Rad51 and Rad54, while in the human melanoma cell line (Mel J) both, the c-NHEJ and HRR pathways were activated (Ref. Reference Rodriguez130).
In vitro experiments in A549 cells, have shown that there is no difference in the initial formation (within 15–30 min) of γ-H2AX foci between low- (γ-rays) and high-LET (carbon ions) radiation (Ref. Reference Ghosh131). However, when the DNA damage repair is investigated at later time points, it shows delayed or less complete repair of DSBs in case of high-LET radiation (Refs Reference Rydberg132, Reference Autsavapromporn133). Recently, the cellular responses in terms of DNA damage and repair efficacy after a mixture of radiation beams have gained interest. (Ref. Reference Maliszewska-Olejniczak50). In the clinic, cancer patients treated with proton and carbon beams are exposed to mixed radiation fields of, that is to high-energy nuclear particles which, based on nuclear interactions, produce low-energy secondary particles (for example electrons) of high-LET that contribute considerably to overall radiation effects (Ref. Reference Saha134). Many therapies besides BNCT, such as intensity-modulated radiotherapy (IMRT), proton therapy, and hadron therapy also produce mixed fields of radiation (Ref. Reference Facoetti135). Experiments using neutron and gamma rays mixed fields showed some minor differences compared to single neutron or gamma radiation exposures (Ref. Reference Okumura136). While results on mixed irradiation with α-particles and X-rays show an increased amount of DNA damage (above the combined additive outcome) and delay in repair, which substantiate the idea that exposure to mixed beams presents a challenge for the DDR (Ref. Reference Cheng137).
The Role of apoptosis, autophagy, necrosis and senescence in high-LET radiotherapy
Cells exposed to IR can exhibit a multitude of responses. Depending on the radiation type, LET (low- or high-), dose, dose rate and radiosensitivity of the exposed cell type, IR may induce cell death through apoptosis, autophagy or necrosis, mitotic catastrophe or even cell cycle arrest and accelerated senescence (Ref. Reference Golden138). For most cancer cell types, mitotic catastrophe is the most common end result of IR, whereas apoptosis (programmed cell death) is associated primarily with hematopoietic cancers (Refs Reference Eriksson and Stigbrand139, Reference Radford140) which have a lower apoptotic threshold. The primary aim of RT is to clonogenically kill cancer cells, causing the minimum disruption to healthy ones; (Refs Reference Di Pietro141, Reference Jinno-Oue142). Apoptotic pathways after IR have been defined and these are the membrane stress pathway, the intrinsic pathway and the extrinsic pathway (Ref. Reference Rahmanian, Hosseinimehr and Khalaj143). Different studies for example on the head and neck cancer underline the differences between cell death mechanisms for low or high-LET radiation therapies (for a recent review please see (Ref. Reference Fabbrizi and Parsons144)).
The intrinsic pathway of apoptosis is mediated by DNA SSBs and DSBs. When DNA lesions are repairable, the emerging growth arrest is transient, eventually resulting in DNA repair, cell cycle continuation, and a return to cell replication and growth. In contrast, when DNA lesions are severe or irreparable (e.g. severe DNA damage or complex damage), prolonged DDR signalling is triggered, resulting in apoptosis or senescence (permanent growth arrest) (Ref. Reference Campisi and d'Adda di Fagagna145). The extrinsic pathway on the other hand, involves signalling through death receptors (DRs), which belong to the tumour necrosis factor (TNF) receptor superfamily. The accumulation of p53 is critical to IR-induced apoptosis or senescence (Ref. Reference Alvarado-Ortiz146). Several target genes of the p53 transcription factor encode proteins, such as p21 and p27 that induce cell-cycle arrest, PAI1 and CDKN1b which are involved in the induction of senescence, or PUMA, BAX and NOXA which are mediators of apoptosis (Ref. Reference Kastenhuber and Lowe147). Most solid tumour cells have either inactivating p53 mutations or defective apoptotic machinery, which makes them radioresistant and might affect the response to RT (Refs Reference Rödel148, Reference Cuddihy and Bristow149). Necrosis, another type of cell death, is an outcome usually appearing in response to very large doses of radiation (>55 Gy), although as a rare side-effect (Ref. Reference MacDonald, Gunderson and Tepper150). There are cases though, that even doses as low as 2–4 Gy can cause significant necrosis. Most replicating cells die by the mitotic catastrophe which mistakenly some researchers equate to necrosis [the reader can consult this seminal review (Ref. Reference Brown and Wouters151)].
It may occur in lower doses as well, but it is often viewed as an unregulated event. Autophagy is a mechanism responsible to maintain metabolic homoeostasis through the segregation of damaged or unwanted cytoplasmic constituents into autophagosomes, destined for lysosomal degradation. Autophagy is directly correlated to the DDR, since there is evidence that is activated by DNA damage and is required for several functional outcomes of DDR signalling, including repair of DNA lesions, senescence, cell death and cytokine secretion (Ref. Reference Eliopoulos, Havaki and Gorgoulis152). In response to IR, it is yet unclear whether autophagy promotes cell survival or cell death (Refs Reference Wu153–Reference Palumbo and Comincini155) although it could be either of the two depending on cell context and dose. Senescence is the process by which cells irreversibly stop proliferating and enter a state of permanent growth arrest (Ref. Reference Gorgoulis156). IR has been shown to induce senescence especially via the induction of DNA damage. Understanding the mechanisms that result in one of the above cellular responses is extremely important especially for an effective RT. Patients that have received stereotactic body radiation therapy (SBRT), frequently experience recurrence [e.g. non-small cell lung cancer (NSCLC) patients, stage I], reducing the overall effectiveness of radiotherapy effective (Ref. Reference Spratt157). It has been postulated that tumour recurrence is can derive from senescent transformed cells in the tumour area which reverse or escape the senescent phenotype (Ref. Reference Liao158).
Due to the importance of RT induced-cell death, many studies have been performed with proton, carbon and even FLASH radiation in an attempt to improve the efficacy of RT. For example, Zhang et al. showed that irradiation of chemoresistant cancer stem cells (CSCs) with 4Gy of protons significantly lowered cell viability, invasiveness and a number of tumour spheres compared to gamma rays. This effect was accompanied by increased apoptosis and higher ROS level (Ref. Reference Zhang159). In general, there is an agreement in the literature that proton radiation causes increased levels of apoptosis than photon radiation (Refs Reference Di Pietro141, Reference Zhang159, Reference Ristic-Fira160). Miszczyk et al. investigated the rate of cell killing by apoptosis or necrosis in human peripheral blood lymphocytes and found that irradiation with 60 MeV SOBP caused higher levels of cell death compared to 250 kV photons through necrosis (Ref. Reference Miszczyk161). Wang et al. assessed cell death in four HNSCC cell lines after a single dose of 4 Gy 6 MV photons or 200 MeV SOBP protons and didn't find any differences between the levels of necrosis and apoptosis. They did however, observe increased senescence and mitotic catastrophe following proton irradiation (Ref. Reference Wang162). These differences can be attributed to the different cell types used for the experiments. Normal human diploid lung fibroblasts exposed to FLASH dose rate protons, showed that proton dose rate did not influence cell killing, but by increasing the dose or the dose rate they reported different rates of senescence. After they exposed cells to 20 Gy 4.5 MeV protons, they found that compared to 0.05 Gy/s, the FLASH dose rates of 100 Gy/s or 1000 Gy/s led to a reduced number of senescent cells (Ref. Reference Buonanno, Grilj and Brenner163). Han et al. used FLASH radiation (~109 Gy/s, with doses in the range of ~10–40 Gy) on normal mouse embryonic fibroblast cells and analysed early apoptosis, late apoptosis and necrosis of cells under both normoxic and hypoxic conditions (Ref. Reference Han164). Their results showed that FLASH-IR compared to conventional proton therapy induced significant early apoptosis, late apoptosis and necrosis, and the levels of apoptosis increased with time, in either hypoxic or normoxic conditions. Also, in hypoxic conditions, apoptosis and necrosis were significantly lower. As also shown conclusively in Table 4, in most cases, protons induce mainly apoptosis and not necrosis. This could impact radiotherapy because cells undergoing necrosis lose membrane integrity and leak their intracellular components some of which serve as danger signals that stimulate inflammation and strong innate immune responses (immunogenic cell death) (Ref. Reference Rock and Kono165) impacting tumour radiosensitisation and radiation toxicity (Ref. Reference Golden138).
Table 4. Reported cell death responses after high-LET radiation

Atsushi et al. irradiated human glioma cells (NP-2), with carbon ions and found that at 6 Gy the majority of the cells die by apoptosis and autophagy. The residual cells could not form a colony and showed a morphological phenotype consistent with cellular senescence (Ref. Reference Jinno-Oue142). The detection of senescent cells was also confirmed with SA-β-gal staining. The same group also found that irradiation with carbon ions induced cellular senescence in the same cell line lacking functional p53. Finally, one study has provided significant data in response to carbon ion radiation and autophagy, implying that targeting autophagy might enhance the effectiveness of heavy-ion radiotherapy (Ref. Reference Jin173). They also found that by inhibiting autophagy apoptotic cell death was accelerated, resulting in increased radiosensitivity. The biological cell death effects discussed in this section are summarised below in Table 4.
Combinational therapies with high-LET and immune therapies
Radioimmunotherapy aims at delivering radioactive atoms that emit beta particles, alpha particles and Auger electrons to tumours after chemically conjugating the radionuclides to monoclonal antibodies which recognise specific receptors and tumour antigens (Refs Reference Gill174–Reference Larson176). The variable physicochemical properties of radionuclides such as half-lives and path lengths can be harnessed for the delivery of more personalised therapy and have rendered radioimmunotherapy a burgeoning field of research in the past thirty years. There is a plethora of data derived from animal tumour models using different radionuclides and monoclonal antibodies. Two radioimmunotherapeutic agents, 131I-tositumomab (Bexxar) and 90Y-ibritumomab tiuxetan (Zevalin), have been approved by the US Food and Drug Administration (FDA) (Refs Reference Witzig177, Reference Kaminski178) for the treatment of CD20+ B-cell lymphomas and Zevalin has also been approved by European Medicines Agency in 2000 (Ref. Reference Kręcisz179). Despite their clinical efficacy, neither has been broadly utilised in clinical practice due to financial and logistic issues. The recently added arrow into the radioimmunotherapy quiver is the US FDA and European Medicines Agency (EMA) approved 177Lu-oxodotreotide (177Lu-DOTA-Tyr3-octreotate, 177Lu-DOTA-TATE, Lutathera). LUTATHERA is a radiolabeled somatostatin analogue and was designed for the treatment of somatostatin receptor-positive gastroenteropancreatic neuroendocrine tumours (GEP-NETs) (Ref. Reference Kręcisz179).
The clinical success of the radioimmunotherapy agents has yet to be matched for the treatment of solid tumours as most of the monoclonal antibodies are conjugated to low LET β-particle emitters such as 131I, 177Lu or 90Y (LET = 0.1–1.0 keV/μm) which are less potent (Ref. Reference Aghevlian, Boyle and Reilly175). Moreover, β-particle emitters pose dose-limiting normal tissue toxicity to the hematopoietic system due to their wide (2–10 mm) range. However, it has been postulated that toxicity to the bone marrow could potentially be avoided with the use of antibodies conjugated to α-particles emitters (e.g. 225Ac, 213Bi, 212Pb or 211At) and Auger electron emitters (e.g. 111In, 67Ga, 123I or 125I) due to their smaller ranges (28–100μm for a-particles and nanometres for Auger electrons). It has also been hypothesised that the higher LET of α-particles and Auger electrons (50–230 keV/μm and 4 to 26 keV/μm, respectively) can shift radioimmunotherapy towards higher antitumour efficacy (Ref. Reference Aghevlian, Boyle and Reilly175). The exploration of the properties of α-particles and Auger electrons as well as the testing of their antibody-conjugated emitters on animal tumour models have been thoroughly reviewed by Aghevlian et al. (Ref. Reference Aghevlian, Boyle and Reilly175). Prostate cancer (Refs Reference Tan180, Reference Miederer181), disseminated lymphomas (Ref. Reference McDevitt182), ovarian cancer (Ref. Reference Borchardt183), brain tumours (Refs Reference Miederer181, Reference Zalutsky184), mammary carcinoma (Ref. Reference Song185), glioma xenografts (Ref. Reference Zalutsky184), colon cancer xenografts (Refs Reference Eriksson186–Reference Milenic189), leukaemia (Refs Reference Orozco190, Reference Huneke191), breast cancer (Ref. Reference Costantini192) are some of the malignancies that have been treated with radioimmunotherapy using high LET α-particle and Auger electron emitters. Importantly, these findings have resulted in the initiation of several Phase I clinical trials for patients with advanced myeloid leukaemia (ClinicalTrials.gov Identifier: NCT00672165 and NCT01756677) with malignant gliomas (Ref. Reference Zalutsky193) and ovarian cancer (Refs Reference Andersson194–Reference Meredith196).
Conclusions
Particle radiotherapy (RT) and hadron / ion-therapy have substantially advanced the practice of radiotherapy which has traditionally relied on the use of photons. The beneficial potential of particle RT stems from the physical properties of charged particles and the essence of their interactions as they traverse matter. They deposit their energy in a non-uniform way, in contrast to photons and the result is the high level of ionisation clustering due to the increased LET (Fig. 2). The heavy particles' energy to traversing distance curve forms a peak, known as Bragg Peak. Thus, when targeting a tumour they tend to spare more healthy tissue. Ion therapy efficacy varies with the accelerated ion type. A critical characteristic of ion radiations is their relatively higher LET with heavier particles to have greater LET values; LET increases when particles are decelerated by their interaction with matter; the distance before the Bragg Peak depends on their initial energy; a superposition of ions within a range of well-defined energy values forms the so-called SOBP that is used in the clinic.
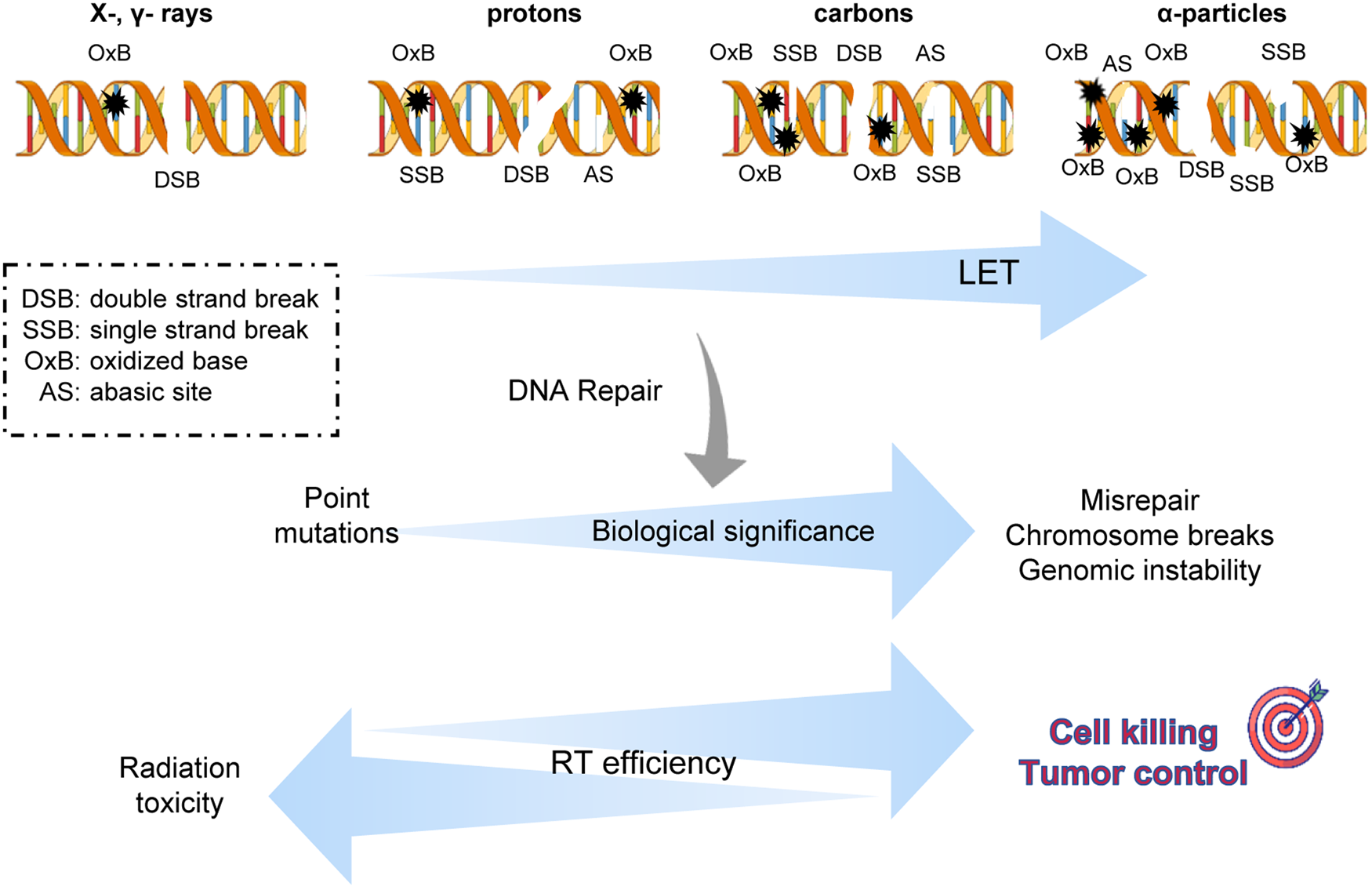
Fig. 2. Biological significance of complex DNA damage induced by high-LET radiation therapy. As the linear energy transfer increases the potency of particle radiation therapies against cancer cells increases due to a higher level of damage complexity that is DNA lesions in clustered form. But more research is needed towards the wide spectrum of biological response not only to tumour cells but normal cells usually receiving smaller doses.
Proton radiotherapy is the most widespread particle-RT in a clinic. Proton RT can be combined with chemotherapeutic agents, likely increasing efficacy and potentially reducing acute adverse events. Another emerging modality is FLASH RT where ultra-high dose rates appear to spare normal tissues to a higher extent than conventional radiation doses, though the precise mechanism it achieves this is currently unknown. Carbon ions allow better tolerance of radiosensitive organs, since they are characterised by a steeper dose distribution area with minimal penumbra compared to protons. α-particles emitted from radionuclides endoscopically placed near the tumour constituting brachytherapy. Another modality exploiting α-particles' high-LET is the one that we called herein as ‘Nuclear radiotherapy’, which is a type of combinatorial particle RT that involves molecule targets that have to be administrated in the tumour area. Nuclear reactions take place and their products, usually α-particles, have higher LET than the incident beam. The efficacy of nuclear RT is limited by a nuclear reaction cross section that is at least 104 times smaller than the corresponding one for the ionisation process.
In conclusion, there are several theoretical appealing features of medium-to-high LET particle radiation therapies as anti-tumour modalities. However, it is evident that, more thorough research is needed for a better understanding of the basic biological mechanisms underlying their effectiveness and for their safer application in the clinic so as to ensure maximum tumour control and minimisation of possible normal tissue toxicity in long term cancer survivors.
Supplementary material
The supplementary material for this article can be found at https://doi.org/10.1017/erm.2022.6.
Financial support
Z.N. is co-financed by Greece and the European Union (European Social Fund- ESF) through the Operational Programme «Human Resources Development, Education and Lifelong Learning» in the context of the project ‘Reinforcement of Postdoctoral Researchers – 2nd Cycle’ (MIS-5033021), implemented by the State Scholarships Foundation (IKY). I.T. would like to state that this research is co-financed by Greece and the European Union (European Social Fund- ESF) through the Operational Programme «Human Resources Development, Education and Lifelong Learning» in the context of the project ‘Strengthening Human Resources Research Potential via Doctorate Research – 2nd Cycle’ (MIS-5000432), implemented by the State Scholarships Foundation (ΙΚΥ). V.G.G is funded by the National Public Investment Program of the Ministry ofDevelopment and Investment/General Secretariat for Research andTechnology, in the framework of the Flagship Initiative to addressSARS-CoV-2 [2020ΣΕ01300001]; the Welfare Foundation for Social & Cultural Sciences (KIKPE), Athens, Greece; H. Pappas [donation]; grants no. [775(Hippo) and 3782 (PACOREL)] from the Hellenic Foundation for Research andInnovation (HFRI); and NKUA-SARG grant [70/3/8916]. A.V and C.K are partially supported by a grant from the National Institutes of Health 1P01CA257904-01.
Conflicts of interest
All authors declare no conflict of interest.