Introduction
The goal of the present review is to present an overview of the most studied hormonal factors that contribute to the central regulation of food intake and energy homeostasis which simultaneously provide peripheral feedback signals to the relevant hypothalamic feedback mechanisms. The most important hormones playing such dual roles are ghrelin, leptin, thyroid hormones, insulin and oestrogen. Of course, in addition to the listed hormones there are several other humoral factors, such as catecholamines, etc, contributing directly or indirectly to the regulation of energy metabolism; however, attempts to cover all contributions are beyond the scope of the present overview. The listed factors highly influence the appetitive trigger that stimulates food-seeking behaviour, ingestion and energy expenditure, thereby establishing a dynamic and plastic information channel between central, mostly hypothalamic, circuits and peripheral tissues. In the present search for the blueprint of the complex regulatory machinery, the hypothesis is that the functions of the hormones discussed in the present review cannot be understood and may be misleading if examined in isolated environments (i.e. singled out from the complex pool of the regulatory circuit). They can only function appropriately when acting together and adequate hormone interactions take place. Thus, we will compare and review the literature on the individual roles of the selected hormones with those observed under the influence of one or more of these hormones present in the actual context examined or discussed. We believe that comparison of individual and combined hormone effects will highlight the shortcomings of classical experimental protocols operating on the basis of only one variable and then interpreting the resulting data as lone-standing constituents of a more complex mechanism. In fact, we believe that this comprehensive review underscores that physiologically relevant data can only be obtained from complex biological systems, and clinical application of experimental results should be designed on the basis of the complex interplay of the biological factors involved.
To best illustrate their individual and combined roles, each of the factors will be discussed separately, then in combination based on the available literature. Considering that we are reviewing an enormous field of research, we will keep the main stream of discussion within the boundaries of general physiology. However, we will use comparative animal physiology or specify sex differences where the examples provide clarity and reveal their complexity for the discussion of feedback loops or mechanisms in the regulation of metabolic homeostasis.
General physiological roles of ghrelin, leptin, thyroid hormones, oestrogen and insulin
Ghrelin
Ghrelin is an acylated twenty-eight-amino acid orexigenic peptide hormone first isolated from rat stomach by Kojima et al. (Reference Kojima, Hosoda and Date1). Ghrelin serum concentrations are below detectable levels during fetal life but rise sharply during the second to third postnatal weeks(Reference Lee, Wang and Englander2). Significant ghrelin production has been observed in the human and rat placenta(Reference Gualillo, Caminos and Blanco3). The X/A-like secretory cells in the oxyntic glands of the stomach are responsible for most of the circulating levels of ghrelin(Reference Kojima, Hosoda and Date1), although the hypothalamus, hypophysis, kidney and the intestines also contribute to ghrelin production. A high degree of ghrelin expression was detected in several types of tumours, particularly the adrenocorticotroph hormone-secreting thymus tumours, gastrinomas, insulinomas, and in human pituitary carcinomas(Reference Korbonits, Bustin and Kojima4, Reference Kanamoto, Akamizu and Hosoda5). Plasma levels of ghrelin are reduced by about 80 % after gastrectomy or fundectomy(Reference Dornonville de la Cour, Bjorkqvist and Sandvik6), indicating that most ghrelin is produced by the oxyntic glands of the stomach.
Besides its role in the regulation of neural circuits that underly appetitive behaviour, feeding(Reference Sun, Asnicar and Smith7–Reference Campa, Pardini and Naccarati9), digestion(Reference Dass, Munonyara and Bassil10–Reference Tesauro, Schinzari and Caramanti12) and thermogenesis(Reference Holst, Holliday and Bach13), ghrelin modulates a number of additional physiological processes that, overall, may affect energy homeostasis, such as growth hormone production(Reference Sun, Asnicar and Smith7, Reference Dass, Munonyara and Bassil10, Reference Smith14), cell proliferation(Reference Campa, Pardini and Naccarati9, Reference Korbonits, Bustin and Kojima15, Reference Volante, Allìa and Gugliotta16), apoptosis(Reference Campa, Pardini and Naccarati9), sleep–wake behaviour(Reference Motivala, Tomiyama and Ziegler17) and cardiovascular functions(Reference Tesauro, Schinzari and Caramanti12, Reference Broglio, Prodam and Me18, Reference Dimitrova, Dimitrov and Iliev19). In addition, ghrelin receptors are found on neurons in the arcuate nucleus of the hypothalamus where appetite-stimulating neuropeptide Y (NPY) and agouti-related protein (AgRP) are co-expressed(Reference Holst, Holliday and Bach13, Reference Holst and Schwartz20), within the hypothalamus, pituitary, small and large intestines, placenta, kidney, testes, ovary and lymphocytes and on insulin-secreting β-cells in a dose-dependent manner(Reference Ghelardoni, Carnicelli and Frascarelli11, Reference Tesauro, Schinzari and Caramanti12, Reference Reimer, Pacini and Ahren21).
Intracerebroventricular or peripheral (intravenous) application of ghrelin stimulates food intake (appetite)(Reference Tschop, Smiley and Heiman22, Reference Wren, Small and Ward23). Studies by several groups have demonstrated that ghrelin supports lipogenesis and suppresses fat pad degradation(Reference Nakazato, Murakami and Date24, Reference De Ambrogi, Volpe and Tamanini25), increasing body weight and adiposity, and leading to obesity. However, skeletal mass or bone growth remains unaffected(Reference Nakazato, Murakami and Date24, Reference De Ambrogi, Volpe and Tamanini25). Thus, ghrelin accelerates the maturation and growth of adipocites in vitro, but may not augment adipocyte differentiation in vivo (Reference Choi, Roh and Hong26, Reference Davies, Kotokorpi and Eccles27).
Although ghrelin is an energy-deficit signal, ghrelin-null or growth hormone secretagogue receptor (GHS-R)-null mice do not display deficiencies in skeletal growth, body composition and food intake(Reference Sun, Ahmed and Smith28, Reference Wortley, Anderson and Garcia29). On high-fat diets, these knock-out animals usually do not become obese and/or insulin resistant(Reference Wortley, del Rincon and Murray30, Reference Longo, Charoenthongtrakul and Giuliana31).
The major stimulus in ghrelin secretion is fasting(Reference Tschop, Wawarta and Riepl32). All parameters, including the pulse amplitude of ghrelin release, are augmented by fasting(Reference Bagnasco, Kalra and Kalra33). Circulating levels of ghrelin increase after fasting and revert after feeding. However, while a relatively short, 24 h fasting increases serum ghrelin levels, a longer period of food restriction does not affect postprandial ghrelin concentrations(Reference Natalucci, Reidl and Gleiss34, Reference Liu, Prudom and Nass35), suggesting that ghrelin levels reflect short-term energy deficits and corroborate a positive energy balance. The preprandial rise of ghrelin's plasma level in obese animals is slower and delayed compared with those animals of normal weight(Reference Ariyasu, Takaya and Hosoda36, Reference le Roux, Patterson and Vincent37). While programmed feeding allows for the observation of marked changes in plasma ghrelin levels, under ad libitum feeding, ghrelin (as well as leptin) secretion shows a pulsatile rhythm or pattern, with a peak every 30 min on average. Ghrelin release is not influenced by simple expansion (mechanical distention) of the stomach, but depends on the ingredients of the meal(Reference le Roux, Patterson and Vincent37–Reference Zhang, Yin and Li39), thus indicating that the energy content of the meal may be a determining factor(Reference Wertz-Lutz, Jennings and Clapper40).
In rodents and humans, ghrelin plasma concentrations follow changes in body weight and adiposity, but show no alterations in circulating levels in ageing rats and mice. Likewise, no alterations were found in ghrelin receptor expression in the pituitary gland and brain of ageing mice(Reference Sun, Garcia and Smith41, Reference Hsu, Pan and Cho42). Ghrelin binds to HDL, decreasing their ability to bind and carry lipid molecules(Reference Beaumont, Skinner and Tan43), inhibiting lipid export from responsive tissue. A circadian rhythm in the fluctuation of plasma ghrelin levels exists in rodents, with the highest concentrations of circulating ghrelin occurring before the dark period(Reference Bodosi, Gardi and Hajdu44) and after waking/just before breakfast in humans(Reference Yildiz, Suchard and Wong45). Gastric ghrelin secretion increases in the dark period. In contrast, daytime hypophyseal ghrelin release seems to be higher compared with night-time values. The biodegradation of ghrelin has an important role in the adjustment of plasma ghrelin levels. Tissue esterases and proteases are the key enzymes in the inactivation and biodegradation of ghrelin in the plasma.
The hypothalamic arcuate nucleus is a principlal target of ghrelin. Ghrelin receptors are found on neurons in the arcuate nucleus of the hypothalamus where NPY and AgRP are co-expressed(Reference Holst, Holliday and Bach13, Reference Holst and Schwartz20). Distinct populations of neurons were identified in the arcuate nucleus as major players in the regulation of food intake, such as NPY- and AgRP-containing neurons(Reference Hahn, Breininger and Baskin46) responsible for orexigenic effects (hunger cells), and the melanocyte-stimulating hormone/pro-opiomelanocortin (α-MSH/POMC) satiety cells and cocaine- and amphetamine-regulated transcript (CART)-producing neurons responsible for anorexigenic mechanisms. Hewson et al. (Reference Hewson and Dickson47) suggest that ghrelin exerts its stimulatory effect on appetite and food intake/feeding activity by stimulating NPY release. Intracerebroventricular administration of anti-NPY IgG suppresses ghrelin's (appetite-stimulating) action, although anti-AgRP IgG had the same effect. Nakazato et al. (Reference Nakazato, Murakami and Date24) also describe ghrelin's enhancing effect on AgRP and NPY mRNA levels but found no modification of the influence of NPY/AgRP neurons on energy expenditure.
In concert with these findings, immunohistochemical analyses show a direct connection between NPY/AgRP neurons and ghrelin-containing fibres(Reference Cowley, Smith and Diano48). According to Andrews et al. (Reference Andrews, Liu and Walllingford49), ghrelin seems to act on NPY/AgRP neurons by inducing mitochondrial respiration of these cells, most probably by influencing mitochondrial proliferation and activity of NPY/AgRP neurons via affecting uncoupling protein (UCP) 2.
Some diseases and disorders are marked by altered ghrelin secretion. Prader–Willi syndrome (a genetic disorder characterised by mental retardation, hyperphagia, short stature and muscular hypothonia) presents with higher plasma ghrelin concentrations(Reference DelParigi, Tschop and Heiman50–Reference Haqq, Farooqi and O'Rahilly52), a possible factor for the hyperphagic symptom of the disease. Anorexia nervosa is also characterised by a high ghrelin plasma concentration(Reference Cuntz, Fruhauf and Wawarta53, Reference Soriano-Guillen, Barrios and Campos-Barros54) which returns to a normal level after recovery and weight gain(Reference Otto, Cuntz and Fruehauf55). Patients suffering from short-bowel syndrome have lower plasma ghrelin levels, supposedly due to the missing ghrelin-producing tissues(Reference Krsek, Rosicka and Papezova56). The same is observed after gastric bypass surgery(Reference Geloneze, Tambascia and Pilla57), since ghrelin is predominantly produced in the stomach.
Since more than 90 % of ghrelin is produced by the stomach and duodenum, parts of the gastrointestinal tract affected by gastric bypass surgery, a significant decrease in ghrelin level was observed in most patients who underwent this operation(Reference Cummings, Weigle and Frayo58–Reference Cummings, Foster-Schubert, Carlson and Pitombo60). Indeed, one of the mechanisms by which gastric bypass reduces body weight is suppression of ghrelin production. However, the effects of bypass surgery are much more complex and are not fully understood. There are several other sources reporting that there were no alterations in ghrelin levels after gastric bypass or gastric banding(Reference Rodieux, Giusti and D'Alessio61). This discrepancy in post-surgical ghrelin levels may be due to the difference in surgical techniques involving, for example, treatment of the vagus nerve(Reference Williams, Grill and Cummings62).
Ghrelin appears to be evolutionarily conserved, as it has been found in birds, reptiles, amphibians and fish, although its effects may have served different functions in specific species. In mammals, it is the only gut-derived hormone that stimulates food intake, while in chickens, it has the contradistinct effect of inhibition of feeding(Reference Furuse, Tachibana and Ohgushi63, Reference Kaiya, Furuse and Miyazato64). Ghrelin's functions in other bird species are still unknown, albeit energy balance and thermogenesis may be regulated by ghrelin there as well. Nevertheless, the mechanism is different from that of mammals and is yet to be determined.
Leptin
Leptin is anorexigenic, inhibits excessive deposition of lipids in various tissues and enables the organism to adapt to periods of food deprivation or undernutrition(Reference Chilliard, Delavaud and Bonnet65). The name ‘leptin’ is derived from the Greek word leptos, meaning ‘thin’. Leptin is a member of the cytokine family of peptide hormones first identified as a 16 kDa protein secreted by the adipose tissue to regulate feeding behaviour and energy balance(Reference Zhang, Proenca and Maffei66, Reference Kelesidis, Kelesidis and Chou67). The gene encoding for leptin is identical to the ob gene, discovered in 1995 by Geffroy et al. (Reference Geffroy, De Vos and Staels68) as the factor responsible for obesity when mutated. Hence, the gene was called ‘ob’, abbreviated from the word ‘obesity’. Geffroy et al. localised the leptin's ob gene to the 7th chromosome. Initial evidence correlated levels of serum leptin with adipose tissue mass, suggesting that it was produced by the adipose tissue itself(Reference Attele, Shi and Yuan69). Higher levels of ob mRNA are expressed in the fat of obese population samples compared with thin controls, and leptin release correlates with the size of adipocytes, i.e. larger cells produce proportionally higher quantities of leptin due to accumulation of TAG(Reference Maffei, Halaas and Ravussin70, Reference Friedman and Halaas71). Leptin is produced predominantly, but not exclusively, by white adipose tissue(Reference Soukas, Cohen and Socci72). It is also released by brown adipose tissue, placenta (syncytiotrophoblasts), ovary, skeletal muscle, stomach (epithelial cells in the lower part of fundic glands), mammary epithelial cells, heart, bone marrow, pituitary and the liver (especially in birds)(Reference Taouis, Chen and Daviaud73). In these organs and tissues, leptin acts as a paracrine or autocrine hormone(Reference Chilliard, Delavaud and Bonnet65, Reference Trayhurn74, Reference Zieba, Amstalden and Williams75).
In an early experiment, mouse ob protein (leptin) was purified and administered in small doses both centrally and peripherally to genetically obese (ob/ob) mice, diet-induced obese mice and diabetic (db/db) obese mice. Leptin reduced food intake and body weight in both genetically obese and diet-induced obese mice, but had no effect on db/db obese animals. The behavioural effects after central (intracerebral) administration suggested that leptin acted directly on the neuronal networks that control feeding and energy balance(Reference Campfield, Smith and Guisez76). To summarise, it was proposed that the ob gene encoded the ob protein (leptin) that regulated food intake and energy balance, and that the extreme obesity of the genetically obese (ob/ob) mice was attributable to mutations in the ob gene. It was also suggested that the db gene encoded the receptors for this factor and the extreme obesity of diabetic (db/db) mice was attributed to mutations in the db gene(Reference Coleman77). In another human study on two severely obese children, serum leptin levels were very low despite the markedly elevated fat mass. In both children, a mutation in the leptin gene was found, providing further evidence to support the role of the ob gene in the regulation of leptin levels(Reference Montague, Farooqi and Whitehead78).
Leptin receptor (Ob-R) is coded by the db gene. Cloning of the mouse db gene revealed that it encodes five alternative isoforms (a–e). All isoforms possess identical ligand-binding domains (extracellular), but differ in their C-terminus (intracellular), with Ob-Rb as the only isoform able to activate the correct signal transduction pathway(Reference Tartaglia, Dembski and Weng79). A further experiment showed that the mutant genotype db/db lacks Ob-Rb, and the phenotype of the affected mice was identical to that of genetically obese (ob/ob) mice, characterised by obesity and diabetes (from which the name of the gene, db, is derived)(Reference Brann, Wade and Dhandapani80). Expression of the other receptor isoforms (Ob-Ra, Ob-Rc, Ob-Rd and Ob-Re) remained unaffected, leading to the conclusion that, of the known receptor isoforms, only the Ob-Rb receptor is essential for leptin's weight-regulating effects(Reference Chen, Charlat and Tartaglia81).
Using in situ hybridisation, Ob-Rb receptors were detected in high levels in hypothalamic neurons and hypophyseal cells, suggesting that these anatomical regions are crucial sites of leptin's actions(Reference Fei, Okano and Li82). This is supported by evidence that a single intracerebroventricular injection of leptin reduces food intake at doses with no measurable effect when delivered peripherally(Reference Halaas, Boozer and Blair-West83). In addition to the central nervous system, relatively high Ob-Rb mRNA expression levels were detected in the adipose tissue, skeletal muscle and liver, suggesting that leptin is likely to play a role in peripheral lipid metabolism as well(Reference Fruhbeck84, Reference Chelikani, Glimm and Kennelly85).
The transport mechanisms of leptin through the blood–brain barrier is still undefined, but it has been hypothesised that the receptor Ob-Ra could be involved in an active transport mechanism in certain Ob-Rb-containing neurons that actually project to neurohaemal brain regions (i.e. circumventricular organs) and thus avoid the blood–brain barrier(Reference Ondicova and Mravec86).
In the hypothalamus, the lateral hypothalamic and ventromedial nuclei project both within and outside the hypothalamus and modulate the activity of the parasympathetic and sympathetic nervous systems, two overlapping and redundant mechanisms of the central nervous system that depend upon fight-or-flight or homeostatic demands of the organism(Reference Yi, Serlie and Ackermans87–Reference Kalsbeek, Bruinstroop and Yi89). The dorsomedial hypothalamic nucleus, via its direct anatomical connections with the parasympathetic nervous system, may be involved in integrating information between the lateral hypothalamic, ventromedial and paraventricular nuclei. The paraventricular nucleus controls secretion of peptides from both the posterior and anterior pituitary and projects to nuclei with sympathetic or parasympathetic efferents(Reference Ondicova and Mravec86, Reference Hayes, Skibicka and Grill90, Reference Rinaman91).
Leptin's central effects on the regulation of food intake and energy metabolism are not confined to a single mechanism of action. Besides its direct effects on relevant neural circuits, indirect mechanisms of leptin actions are also known. In general, leptin down-regulates orexigenic peptides, and up-regulates anorexigenic peptides, leading to a reduction in food intake. However, there is a dynamic and interactive effect between orexigenic and anorexigenic factors. For example, α-MSH agonists decrease food intake, while α-MSH antagonists act in the opposite manner to reduce the anorexigenic effect of leptin(Reference Satoh, Ogawa and Katsuura92). On the other hand, peripheral and central effects of leptin can merge to shape the final biological outcome: by the facilitation of lipolysis in the adipose tissue, leptin increases the plasma level of NEFA, the latter which influence the function of relevant hypothalamic neurons, while leptin also acts directly on hypothalamic orexigenic neurons. Additionally, leptin up-regulates uncoupling in UCP-expressing hypothalamic neurons and probably other cells as well, thereby propelling energy metabolism/utilisation/use of energy. Therefore, along with its stimulatory effect on sympathetic tone, leptin's overall effects also include increased heat production(Reference Scarpace, Matheny and Pollock93). In addition to these central actions, leptin exerts considerable peripheral effects that can be considered upstream signals of extended hypothalamic leptin effects. In short, leptin decreases the levels of insulin and glucocorticoids, enhances the secretion of growth hormone, catecholamines and the thyroid hormones, thus stimulating energy expenditure and hepatic lipogenesis(Reference Chilliard, Delavaud and Bonnet65). Leptin augments mitochondrial fatty acid oxidation especially in hepatocytes and skeletal muscle cells(Reference Chilliard, Bonnet and Delavaud94, Reference Gallardo, Bonzon-Kulichenko and Fernandez-Agullo95) and increases insulin sensitivity and glucose metabolism in myocytes(Reference Chilliard, Delavaud and Bonnet65, Reference Bobe, Young and Beitz96). NPY/AgRP neurons are major targets of leptin in the hypothalamus(Reference Mizuno and Mobbs97, Reference Baskin, Hahn and Schwartz98). In these cells, the two antagonistic hormones, ghrelin and leptin, act in opposite ways to modulate neuronal activity and influence food intake, as discussed below.
Thyroid hormones
Traditionally, the hormone products of the thyroid gland have been known as ‘thyroid hormones’. Today, the source and function of these hormones comprise a large body of knowledge, allowing for a remarkably complex view on the physiological roles of thyroid hormones. This brief summary is confined to those salient thyroid hormone effects that, in one way or another, are involved in the hypothalamic regulation of food intake.
There are various types of thyroid hormones: thyroxine (T4), containing four iodine atoms, is generally considered to be a prohormone, while triiodothyronine (T3), containing three iodine atoms, is biologically more active than T4. These two hormones are inactivated by conversion to reverse triiodothyronine (rT3) or diiodothyronine (T2).
Thyroid hormones are crucial for the maintenance of energy equilibrium of the body: thyroid hormones regulate the rate of metabolic processes (including the BMR), increase O2 consumption and heat production. Consequently, thyroid hormones are equally necessary for normal reproduction, growth and development(Reference Connor, Laiakis and Fernandes99).
In humans, most (80 %) of the circulating T3 originates from the periphery (i.e. not from the thyroid gland), where T4 is converted into T3 by the corresponding deiodinase enzymes. The most important peripheral T3-producing organs are the liver, kidney and the skeletal muscle(Reference Larsen100). In rats, more T3 is produced in the thyroid gland (55 %) than in other peripheral tissues(Reference Hulbert101), while in birds almost all (99 %) T3 is synthesised in peripheral tissues, outside of the thyroid gland(Reference Darras, Verhoelst and Reyns102).
The function of the thyroid gland is regulated by a negative feedback mechanism. The hypothalamic nuclei produce thyrotropin-releasing hormone that stimulates the adenohypophysis to produce and secrete thyroid-stimulating hormone (TSH). TSH increases T4 production in the thyroid gland. An increase of plasma T4 concentration, in turn, down-regulates the production of thyrotropin-releasing hormone, TSH, and T4 as well(Reference Tveit and Larsen103).
The peripheral T4-to-T3 conversion is catalysed by deiodinase enzymes(Reference St Germain and Galton104). There are three types of deiodinases in different tissues: type I deiodinase (D1), type II deiodinase (D2) and type III deiodinase (D3). D1 is expressed in the central nervous system, especially the pituitary, in rats but not in humans(Reference Campos-Barros, Hoell and Musa105–Reference Gereben, Zeold and Dentice107). Of the lower vertebrates, the fish brain was found to contain D1(Reference Valverde, Orozco and Becerra108).
D2 expression was described in the brain (including the pineal gland) and pituitary of rats(Reference Bianco, Salvatore and Gereben106) mainly as a glial enzyme most abundant in astrocytes and tanycytes (near the mediobasal hypothalamus)(Reference Guadano-Ferraz, Obregon and St Germain109, Reference Tu, Kim and Salvatore110). In humans, D2 is the only thyroid hormone-activating 5′ deiodinase in the brain(Reference Campos-Barros, Hoell and Musa105).
D3 is ubiquitously expressed in the adult rat brain, especially in the forebrain, cerebral cortex, pyramidal cells of the hippocampus, granule cells of the dentate gyrus, and in the piriform cortex(Reference Tu, Legradi and Bartha111). These brain regions are the richest in thyroid hormone receptors(Reference Puymirat, L'Héreault and Dussault112). In neonatal rat brain, D3 expression is age dependent(Reference Escamez, Guadano-Ferraz and Cuadrado113). In contrast to D2, D3 can be found mostly in neural cells(Reference Tu, Legradi and Bartha111).
D1 and D2 activate (when pH < 7, D1 may also inactivate), while D3 inactivates, thyroid hormone. In mammals, the bulk production of T3 is mediated by D1. D1 is expressed in the liver, kidneys, thyroid gland, skeletal muscles, lung, and in the hypophysis(Reference Rudas, Bartha and Frenyo114–Reference Bianco, Larsen, Braverman and Utiger118). In mammals, hepatic and kidney-derived D1 is the most important source of T3. These organs produce T3 and release it into the bloodstream. Therefore, these are called hormone-exporting organs(Reference Kohrle116, Reference Pezzi, Accorsi and Vigo117, Reference Larsen, Silva and Kaplan119). D2 is also expressed in the liver in birds but not in mammals(Reference Darras, Verhoelst and Reyns102).
Peripheral tissues can adjust the ratio of active and inactive thyroid hormones, according to their actual energy needs and energy supply through their deiodinase activity. Therefore, the peripheral tissues can become more or less independent from the central regulatory processes. In the case of appropriate energy supply, the body primarily needs T3, thereby turning on thyroid hormone-activating deiodination(Reference Pezzi, Accorsi and Vigo117, Reference Larsen, Silva and Kaplan119, Reference Rudas and Pethes120).
Thyroid hormones act through binding to their cognate receptors (thyroid hormone receptors). Thyroid hormone receptors are nuclear receptors that can stimulate ligand-dependent transcription of certain genes(Reference Sap, de Magistris and Stunnenberg121, Reference Brent, Moore and Larsen122). In mammals, there are four thyroid hormone receptor isoforms, thyroid hormone receptor α (TRα) 1–2 and thyroid hormone receptor β (TRβ) 1–2, encoded by two highly conserved genes (Thrα and Thrβ, respectively)(Reference Shi, Yaoita and Brown123, Reference Zhang and Lazar124). TRα2 has not been found in non-mammalian species(Reference Koenig, Lazar and Hodin125). Unlike the other thyroid hormone receptors, TRα2 acts as a weak repressor instead of functioning as a receptor of T3(Reference Salto, Kindblom and Johansson126). Thyroid hormone receptors have both T3-dependent and -independent functions. Interestingly, T3 can convert a T3-independent repressor into a T3-dependent activator(Reference Chen and Evans127).
The distribution pattern of Thrα and Thrβ is age and tissue dependent. While TRα1 is widely expressed, TRβ 1–2 mRNA expression is restricted to specific ontogenetic states and is highly tissue specific(Reference Bradley, Towle and Young128). Based on knock-out and knock-in experiments, Thrα was found to influence cardiac functions, thermogenesis, haemopoiesis, and the maturation of intestines and bones(Reference Bradley, Towle and Young128–Reference Plateroti, Kress and Mori131). Thrβ is crucial for the proper endocrine and sensory functions such as the hypothalamic–pituitary–thyroid axis, hepatic reactions to T3, behaviour, audition, colour sensation and tactile senses(Reference Amma, Campos-Barros and Wang132–Reference Forrest, Erway and Ng138). TRα1 and TRβ1 isoforms can be co-expressed in the same tissues and can substitute for each other's function to a certain extent(Reference Forrest, Erway and Ng138, Reference Gothe, Wang and Ng139).
Nutrients entering the small intestines elicit enteroglucagon production in the intestinal wall that reaches the liver before the absorption of the nutrients(Reference Drucker140). Absorbed nutrients quickly enter the liver through the portal vein. Carbohydrates, and to a lesser extent proteins, induce hepatic D1 gene expression(Reference Danforth and Burger141–Reference Brown, Maloney and Kinlaw143) and down-regulate D3 activity. T3, in turn, increases hepatic and muscular GLUT (GLUT-1 and GLUT-5) gene expression(Reference Hulbert101, Reference Matosin-Matekalo, Mesonero and Laroche144). The latter also enhances hepatic thyroid hormone activation(Reference Sato and Robbins145). Increased energy intake, therefore, induces increased plasma T3 concentration that increases BMR(Reference Pezzi, Accorsi and Vigo117). Increased, but still physiological plasma T3 concentrations, in turn, trigger appetite in the hypothalamic ventromedial nucleus. This is a self-strengthening mechanism(Reference Kong, Martin and Smith146, Reference Győrffy, Rónai and Áprily147).
In piglets, thyroid hormone plasma concentration (mostly T3) increases sharply after food intake, reaching its peak after 60 min. The rise in thyroid hormone concentration mainly depends on the energy content of the food and is relatively independent from the glucose concentration of the blood(Reference Hulbert101). In contrast to feeding, starvation elicits a decrease in plasma T3 concentration in mammals. T4 response to starvation, however, depends on the species. For example, starvation leaves plasma T4 concentration unaffected in humans(Reference Hugues, Burger and Pekary148), but in rats(Reference Kaplan and Utiger149, Reference Boelen, Wiersinga and Fliers150) and swine(Reference Slebodzinski, Brzezinska-Slebodzinska and Drews151–Reference Houpt, Reimers and Boyd153) plasma T3 concentrations drop after starvation. In chickens, starvation causes decreased plasma T3 and increased plasma T4 concentration(Reference Van der Geyten, Van Rompaey and Sanders115, Reference King, King and Eshleman154–Reference Reyns, Janssens and Buyse157) due to increased hepatic D3(Reference Tu, Legradi and Bartha111) and decreased hepatic D2 activity and gene expression(Reference Győrffy, Sayed-Ahmed and Zsarnovszky158). In cows, effects of starvation are difficult to examine due to the characteristics of rumen function. Therefore, the effect of fluctuations in feeding on plasma thyroid hormone concentration was tested and it was found that reduced food intake resulted in decreased plasma thyroid hormone concentration(Reference Pethes, Bokori and Rudas159).
Dairy cows represent a unique category with regard to thyroid hormone physiology. These animals can enter a physiologically starvation-like period during lactation when energy metabolism turns towards so-called negative energy balance. Under such conditions, plasma T4 concentration reaches its nadir and T3 plasma concentration is low. Later, plasma thyroid hormone concentrations increase and reach their peak during the dry period(Reference Pethes, Bokori and Rudas159). Romo et al. (Reference Romo, Elsasser and Kahl160) and Pezzi et al. (Reference Pezzi, Accorsi and Vigo117) found that hepatic D1 activity is the lowest during early lactation and suggested a possible role of fatty liver in the decreased enzyme activity and the consequently decreased hepatic thyroid hormone activation. However, decreased feed (and consequently carbohydrate) intake can also be acting in the background of decreased thyroid hormone activation since T4-to-T3 conversion is a carbohydrate-dependent process(Reference Danforth and Burger141) and glucose directly triggers hepatic D1 expression(Reference Brown, Maloney and Kinlaw143).
As mentioned above, hypothalamic NPY/AgRP neurons are in a special position to trigger food intake, and both leptin and ghrelin are able to influence the activity of these cells. Coppola et al. (Reference Coppola, Liu and Andrews161) clearly demonstrated that arcuate nucleus NPY/AgRP neurons are supplied with T3 by hypothalamic tanycytes, and that T3, imported from tanycytes, can activate intracellular mechanisms in NPY/AgRP cells to increase neuronal firing and, consequentially, to initiate food intake. These observations imply a possible interaction between the three hormones discussed so far, in the regulation of NPY/AgRP neuronal activity. However, according to Ishii et al. (Reference Ishii, Kamegai and Tamura162), subcutaneously injected T3 results in hyperphagia via hypothalamic adenosine monophosphate activated protein kinase (AMPK) phosphorylation, without affecting local (hypothalamic) NPY or POMC mRNA expression levels, suggesting that additional mechanisms may also be involved in the hypothalamic regulation of food intake.
Oestrogen
Traditionally, oestrogens (mostly 17β-oestradiol; E2) have been regarded exclusively as sex hormones, and, indeed, many of their direct actions are exerted on reproductive organs and tissues. Oestrogens are primarily synthesised within the developing follicles of the ovaries and exert an influence on the female reproductive system. However, other sites of biosynthesis are present throughout the body, including the adipose tissue and the brain itself. Adipose tissue contributes significantly to the maintenance of plasma concentration of E2. Besides the adipose tissue, aromatase (oestrogen synthase) expression is also found in muscle, skin fibroblasts, osteoblasts and chondrocytes of bones, vascular endothelial and aortic smooth muscle cells, Leydig cells, as well as a number of sites in the brain including the medial preoptic/anterior hypothalamus, the mediobasal hypothalamus and the amygdala(Reference Nelson and Bulun163, Reference Sharpe164). Oestrogens exert an effect on almost every cell type, regulating an eclectic assortment of functions.
In males, regulation of E2 mainly occurs through the conversion of testosterone using aromatase enzyme throughout the body, including the adipose tissue that represents a major source of ovary-independent production(Reference Sharpe164). A positive relationship was described between BMI and plasma E2 levels in men and postmenopausal women, and circulating levels of E2 decreased in response to fasting or energy restriction in fertile women(Reference Mystkowski and Schwartz165). Rats lacking oestrogen receptor α (ERα) or aromatase have increased fat mass and hyperlipidaemia(Reference Meli, Pacilio and Raso166). This suggests that E2 has a role in signalling the central nervous system about the status of peripheral energy stores.
It has long been known that E2 modulates hunger(Reference Meli, Pacilio and Raso166–Reference Shimomura, Shimizu and Kobayashi168). The question, however, of how much is the share of E2 alone, besides other relevant hormones, in the regulation of food intake is not fully known. Apart from the actual initiation of food intake, effects on energy expenditure highly influence evolving hunger signals. Therefore, E2 can modulate feedback hunger signals through its central and peripheral regulatory role in the initiation of heat production(Reference Uchida, Kano and Yasuhara169). The homeostatic functions of E2, such as reproduction, stress responses, feeding, sleep cycles and temperature regulation were originally thought to be mediated through E2's classical intracellular/nuclear receptors (oestrogen receptors) ERα and oestrogen receptor β (Erβ), which act as transcription factors, binding to the oestrogen-responsive elements (ERE)(Reference Qiu, Bosch and Tobias170, Reference Qiu, Bosch and Tobias171) of respective genes. Keeping with the general context of the present review, a recent study reported that elimination of ERα (ERαKO) from the hypothalamic ventromedial nucleus in rats leads to the development of the metabolic syndrome, with a significant deficit in energy expenditure(Reference Musatov, Chen and Pfaff172). In addition to nuclear oestrogen receptors, there are other extranuclear pathways activated after ligands bind to the oestrogen receptor in the plasma membrane(Reference Wong, Le and Zsarnovszky173, Reference Belcher and Zsarnovszky174), or activate intracellular proteins directly responsible for the regulation of cellular energy levels, such as UCP(Reference Horvath, Warden and Hajos175) or ectonucleotidases(Reference Kiss, Zsarnovszky and Horvath176). Oestrogens can also initiate cellular actions through membrane-bound E2-sensitive molecular complexes, such as G-protein-coupled receptor 30, ion channels and receptors for other ligands(Reference Ropero, Alonso-Magdalena and Quesada177). An interesting mechanism of oestrogenic effects is the synaptogenic influence of E2 on hypothalamic neuronal connectivity. It is now well established that E2 induces synaptic reorganisation in the hypothalamus to regulate the secretion and release of gonadotropin-releasing hormone and, consequentially, pituitary luteinising hormone release(Reference Naftolin, Garcia-Segura and Horvath178). Synaptic plasticity plays a crucial role in the hypothalamic mediation of leptin and ghrelin effects as well(Reference Horvath179), and overall synaptic reorganisation in the hypothalamus seems to be shaped by all three hormones (E2, leptin and ghrelin). However, E2 appears to exert its own synaptogenic influence in the hypothalamus, since apart from the ovarian cycle, hunger is also modulated by the actual levels of E2(Reference Wade, Gray and Bartness167, Reference Shimomura, Shimizu and Kobayashi168). It is now well established that oestrogens (E2 above all) are trophic hormones. As such, a plethora of studies reported that E2 interacts with other trophic hormones, including the ones discussed in the present review, to regulate developmental events and energy metabolism (food intake and energy expenditure)(Reference Kalsbeek, Fliers and Hofman180–Reference Olofsson, Pierce and Xu183). A relevant selection of these interactions will be discussed below. At this point, we only note that E2, while targeting hypothalamic neurons, is able to influence the function of NPY/AgRP-containing neurons of the arcuate nucleus, the major mediators–initiators of food intake, and thus play a significant role in shaping the overall outcome of NPY/AgRP neuronal activity(Reference Horvath179, Reference Gao and Horvath182). The latter role includes a blunting effect after fasting-induced activation of these neurons(Reference Olofsson, Pierce and Xu183). Interestingly, however, E2 not only attenuates hunger by down-regulating relevant hypothalamic ‘hunger cell’ activity (NPY/AgRP neurons), but also modulates neurotransmission on POMC neurons (satiety cells) to attenuate the cannabinoid-induced changes in appetite(Reference Kellert, Nguyen and Nguyen184).
Insulin
Insulin is secreted by pancreatic islet cells (β-cells) and is known as a major regulator of blood glucose levels. To date, a large body of evidence demonstrates actions of insulin in the hypothalamic feedback loop of energy balance regulation(Reference Schwartz, Figlewicz and Baskin185). The actual concentration of plasma insulin is proportional to body adiposity, although it also depends on food consumption and type of diet. Secretion and synthesis of insulin are affected by a number of genetic and environmental/epigenetic factors. Food intake, diet, fasting and re-feeding influence insulin entry to the brain or its ability to get into the brain tissue(Reference Kaiyala, Prigeon and Kahn186). Eating increases the concentration of insulin in the brain whereas fasting decreases the concentration. Plasma insulin levels directly correlate with those measured in the cerebrospinal fluid. Insulin enters the brain, crossing the blood–brain barrier by a receptor-mediated transport, and stimulates anorexigenic pathways as an adiposity signal leading to reduced food intake. Insulin receptors are expressed in hypothalamic neurons, predominantly in the arcuate nucleus(Reference Pardini, Nguyen and Figlewitz187), a nucleus associated with the regulation of food intake, energy expenditure and food reward and the site of several orexigenic and anorexigenic neural signals(Reference Kalsbeek, Fliers and Hofman180, Reference Gao and Horvath182, Reference Gerozissis188, Reference Figlewicz189). In rats, hyperphagia caused by insulin-deficient type 1 diabetes can be reversed by intracerebroventricular administration of insulin. Thus, insulin interacts with both orexigenic and anorexigenic neuromodulators(Reference Figlewicz189, Reference Air, Benoit and Clegg190).
Reports from the past three decades show that insulin exerts multiple effects in the brain, and its role has been validated by the identification of receptors and transport machinery in the central nervous system. Insulin acts on hypothalamic circuits that regulate food intake, metabolism and other crucial functions, and insulin-responsive neurons include the hypothalamic NPY/AgRP neurons(Reference Pralong191), the latter which may represent a special cell population allowing for a cross-talk between the major hormonal signals discussed in the present review. The effects of insulin leading to decreased food intake are, at least in part, due to the inhibition of NPY expression of neurons of the arcuate nucleus(Reference Frankish, Dryden and Hopkins192). An interesting hypothalamic effect of insulin was observed by Lin et al. (Reference Lin, Plum and Ono193), who found that hypothalamic NPY/AgRP and POMC neurons mediate insulin's central effects to regulate hepatic glucose production.
Part of the endocrine cross-talk appears to manifest in the hormonal regulation of hypothalamic synaptic reorganisation, where the interaction of insulin-like growth factors and their receptors are a well-known episode in the regulation of synaptic plasticity(Reference Naftolin, Garcia-Segura and Horvath178).
Despite the abundance of relevant data, a clearcut borderline between the effects of insulin alone and those exerted through the interactions of insulin with other peripheral trophic hormones in the hypothalamic regulation of food intake is not yet known. Therefore, further effects of insulin on food intake will be discussed below in the context of hormone interactions.
Hormone interactions and combined hormone effects
Interactions of ghrelin and leptin
In mammals, ghrelin stimulates food intake and appears to act as the functional antagonist of leptin(Reference Zigman and Elmquist194). There is a fine balance between the two physiologically antagonistic hormones as demonstrated by Giovambattista et al. (Reference Giovambattista, Piermaria and Suescun195), who detected enhanced leptin secretion after prolonged ghrelin exposure. In turn, moderate hyperleptinaemia/leptin administration prevents the increase of plasma ghrelin during short-term energy restriction(Reference Barazzoni, Zanetti and Stebel196). Ghrelin's hunger signal stimulates the hypothalamic regions responsible for food intake in the lateral hypothalamic nuclei and arcuate nucleus. In contrast to leptin, plasma levels of ghrelin fluctuate in relation to food intake. The low ghrelin levels in obese individuals are associated with low growth hormone concentration and high leptin levels(Reference Tschop, Wawarta and Riepl32, Reference Shiiya, Nakazato and Mizuta197). Rigamonti et al. (Reference Rigamonti, Pincelli and Corra198) found higher concentrations of plasma ghrelin in young patients compared with elderly patients. Thus, it is possible that a high concentration of leptin suppresses ghrelin's plasma level. This idea is supported by observations that plasma leptin concentration correlates with the body fat mass which is high in obesity, decreased in fasting and low in anorexia nervosa.
An interesting addition for ghrelin and leptin regulation is the linkage to sleep–wake behaviour(Reference Motivala, Tomiyama and Ziegler17). It has been demonstrated that inadequate or bad-quality sleep is associated with changed levels of ghrelin and leptin, and is in connection with energy balance disorders leading to weight gain. Normally, ghrelin levels rise at night. In sleep deprivation or insomnia, however, night-time ghrelin levels fall significantly and are followed by increased daytime ghrelin levels. Administration of ghrelin increases the ratio of non-rapid eye movement (REM) sleep in human subjects. The REM phase is thought to be the stage with the highest metabolic rate. Lack of it leads to hormonal changes that increase appetite. It is noteworthy that insufficient sleep can decrease daytime levels of leptin; however, its nocturnal levels do not show significant change between healthy individuals and insomniacs.
The anatomy and synaptology of the hypothalamic leptin/ghrelin-responsive neurons provide an explanation for the aforementioned interactions. In a review, Horvath(Reference Horvath179) pointed to the ability of hypothalamic neuronal circuits to rearrange themselves in response to peripheral and central hormone effects. Earlier, this phenomenon had not been considered as part of the mechanism responsible for the regulation of daily energy metabolism. However, as stressed in that review, experiments on ob/ob mice and transgenic animals provided clearcut evidence for the ability of leptin and ghrelin to induce synaptic reorganisation on hypothalamic neurons that are in a key position (NPY/AgRP and POMC cells) to regulate food intake and other determinants of energy homeostasis. Hypocretin/orexin-containing neurons of the lateral hypothalamus are major targets of gastric ghrelin and, at the same time, these neurons directly innervate raphe serotonergic neurons to regulate sleep–wake behaviour. Elevation of serum ghrelin levels increases the number of excitatory synapses and the ratio of stimulatory/inhibitory synapses on lateral hypothalamic hypocretin/orexin neurons. Since such a ratio in cellular synaptic status is a key factor in the shaping of neuronal activity, the consequence of such a synaptic reorganisation is that the neurons in question, as major sources of the stimulatory innervation of arcuate nucleus NPY/AgRP cells, increase the activity of the latter cell population, thereby initiating/increasing food intake. Such synaptic plasticity occurs simultaneously on POMC neurons as well, where an inhibitory dominance occurs at the time of increased NPY/AgRP cell activity. The described synaptological basis of the mechanism includes the anti-ghrelin-like (antagonistic) effects of leptin, but is also modulated by the synaptogenic effects of E2 and other hormones discussed in the present review.
Interactions of ghrelin and insulin
As mentioned above, ghrelin release is not influenced by simple expansion (mechanical distention) of the stomach; instead, it is highly dependent on the ingredients of the meal. Elevated blood glucose level, after oral or intravenous application, leads to decreased plasma ghrelin(Reference Shiiya, Nakazato and Mizuta197, Reference McCowen, Maykel and Bistrian199, Reference Rosicka, Krsek and Jarkovska200). In turn, ghrelin seems to enhance the degradation/catabolism of carbohydrates and results in an increase in the RQ. According to Ariyasu et al. (Reference Ariyasu, Takaya and Hosoda36), insulin-induced hypoglycaemia facilitates ghrelin secretion in rodents and this can be reversed by glucose injections. Ghrelin levels are increased under lipid-rich diets, but not under low-protein diets(Reference Erdmann, Lippl and Schusdziarra201, Reference Greenman, Golani and Gilad202).
As emphasised in the present review, the arcuate nucleus is a key brain region regulating energy balance. Peripheral hormonal signals converge to influence arcuate nucleus circuits and shape overall energy metabolism. Among these signals are ghrelin and insulin, whose common targets are the arcuate nucleus NPY/AgRP neurons, the ‘hunger cells’ of the hypothalamus. As described above, ghrelin stimulates the activity, while insulin down-regulates NPY secretion of these cells; thus these two hormones antagonise in the regulation of ‘hunger cell’ function. Apart from their hypothalamic effects, parallel brain circuits are also modulated by the two hormones. For example, there is evidence that brainstem regions, such as the ventral tegmental area, are sensitive to leptin, insulin and ghrelin and that the activity of dopaminergic cells of the ventral tegmental area are modulated by the above hormones(Reference Figlewicz, Evans and Murphy203, Reference Guan, Yu and Palyha204). In turn, the ventral tegmental area dopaminergic cells innervate a number of forebrain structures, forming circuits that are commonly associated with the expectation of feeding-associated reward(Reference Wise205–Reference Wise and Rompre207).
It is noteworthy that besides their central effects, there are certain peripheral interactions of ghrelin and insulin that also influence hypothalamic neural mechanisms. For example, a wide range of findings supports roles of ghrelin in the regulation of insulin release. Ghrelin receptors are present on insulin-secreting β-cells(Reference Reimer, Pacini and Ahren21) and ghrelin immunoreactivity is reported in pancreatic islets partly overlapped with glucagon-immunoreactive cells. Data suggest that ghrelin is synthesised and secreted in pancreatic α-cells and feeds back on the same α-cells in an autocrine and/or paracrine manner. Ghrelin concentration in pancreatic veins is higher than in pancreatic arteries, suggesting that pancreatic islets produce ghrelin as well(Reference Date, Nakazato and Hashiguchi208, Reference Kageyama, Funahashi and Hirayama209). In support of the latter, in their review, Dezaki et al. (Reference Dezaki, Sone and Yada210) described that ghrelin is present in pancreatic islet cells and regulates β-cell insulin secretion.
It was recently established that (exogenous) ghrelin administration increases plasma glucose and reduces insulin levels(Reference Yada, Dezaki and Sone211). These results are supported by the finding that ghrelin knock-out mice show elevated insulin production in pancreatic islets, which leads to decreased blood glucose levels(Reference Dezaki, Kakei and Yada212, Reference Dezaki, Hosoda and Kakei213). The main regulator of insulin release seems to be the glucose-induced Ca influx into the β-cells. Dezaki(Reference Dezaki, Hosoda and Kakei213) described the Ca-mediated insulin release that could be attenuated by ghrelin administration. The mechanism underlying this observation involves ghrelin's activating effect on β-cell growth hormone secretagogue receptors (GHS-R) to enhance K+ currents through delayed rectifier K-channels via G-protein activation; this, in turn, attenuates the glucose-induced Ca influx and thereby decreases insulin secretion(Reference Dezaki, Sone and Yada210).
Yet another finding to consider is that ghrelin attenuates the expression of UCP2 in ob/ob mice, which, under normal circumstances, down-regulates glucose-induced ATP production, reducing insulin release from β-cells. In summary, ghrelin inhibits insulin secretion in islet β-cells in two ways: (1) by acutely enhancing Kv channels and suppressing Ca signalling; and (2) by chronically up-regulating UCP2. Consequently, ghrelin inhibits insulin release, thereby increasing glycaemia, and affecting feeding behaviour and adiposity(Reference Dezaki, Sone and Yada210, Reference Yada, Dezaki and Sone211).
Interactions of leptin and thyroid hormones
Both leptin and the thyroid hormones are key regulators of body weight by affecting food intake and energy expenditure(Reference Rogers, Barnes and Hermann214). Leptin decreases food intake and increases energy expenditure, while the thyroid hormones T4 and T3 increase both food intake and energy expenditure. This indicates that thyroid hormones and leptin could play a crucial role in the regulation of the balance between energy intake and energy expenditure, and, consequently, in the regulation of body weight and body fat mass. The correlation between serum leptin and T3 concentrations has been studied extensively. Results so far are conflicting, particularly those from human studies(Reference Zabrocka, Klimek and Swierczynski215).
Study on cultured rat adipocytes demonstrated that leptin mRNA production and thus leptin secretion was inhibited by Triac (triiodothyroacetic acid, a metabolite of T3) and T3 in a dose-dependent manner and with similar potency(Reference Medina-Gomez, Calvo and Obregon216). This was confirmed in an in vivo rat study, in which intraperitoneal administration of different doses of T3 (and consequently increased serum T3) led to a decrease in white adipose leptin mRNA levels and a decrease in serum leptin concentration. This observation indicates that T3 regulates serum leptin through the modulation of leptin gene (ob) expression(Reference Wise205).
Conversely, administration of leptin has been shown to affect serum thyroid hormone levels. When administered to fasted rats, leptin prevented the reduction in thyroid hormone levels by maintaining normal pro-thyrotropin-releasing hormone (precursor to thyrotropin-releasing hormone) mRNA activity in the hypothalamus(Reference Legradi, Emerson and Ahima217). Likewise, leptin enhanced T4-to-T3 conversion(Reference Legradi, Emerson and Ahima217). Leptin also prevents the suppression of TSH in the anterior pituitary after food restriction, thereby maintaining serum T3 and T4 levels. However, this anti-inhibitory effect of TSH also depends on thyroid gland activity, as it was not observed in hypothyroid rats(Reference Seoane, Carro and Tovar218).
In the brain, T4-to-T3 conversion is catalysed by D2. According to Coppola et al. (Reference Coppola, Liu and Andrews161), in the arcuate nucleus, D2 activity takes place in tanycytes, and resulting T3 is then transferred to NPY/AgRP neurons. T3 in the latter cell population triggers a series of events including the up-regulation of UCP2 and NPY, eventually leading to mitochondrial proliferation and increased neuronal activity. The physiological outcome of these cellular events manifests in the initiation of or in an increase in food intake. As previously mentioned, leptin up-regulates uncoupling in UCP2-expressing hypothalamic neurons. This might be, at least in part, due to leptin's ability to increase T3 levels by acting centrally on D2 activity(Reference Cettour-Rose, Burger and Meier219). Interestingly, however, as an anorexigenic hormone, leptin down-regulates NPY/AgRP neuronal functions. Therefore, in terms of food intake, it seems likely that T3's and leptin's opposing physiological effects on NPY neurons are mediated by different signalling modalities with possible cross-talk at the level of UCP2. UCP2, and other uncoupling enzymes elsewhere in the body, may be common targets of T3 and leptin in their regulatory actions in heat production. The latter regulatory process probably involves parallel neural circuits. For example, the expression level of UCP1 in brown adipose tissue is a marker of sympathetic nervous system activity. It has been shown that D2 activity, in brown adipose tissue in particular, can be stimulated by the sympathetic nervous system(Reference Cettour-Rose, Burger and Meier219) and, not surprisingly, central leptin administration increases UCP1 expression in brown adipose tissue.
In sum, T3 regulates serum leptin levels by inhibiting leptin gene expression, but leptin maintains normal thyroid hormone levels in food-restricted animals by preventing the suppression of thyrotropin-releasing hormone and TSH(Reference Ghamari-Langroudi, Vella and Srisai220). Leptin also increases D1 activity peripherally, and D2 activity centrally in food-restricted animals, resulting in an increase in T3 as long as sufficient T4 is available.
Interactions of oestrogen and leptin
The interactions of E2 and leptin are particularly interesting and deserve more attention, since the two hormones have similar effects on food intake and energy homeostasis. For example, leptin limits food intake, i.e. decreased leptin levels result in hyperphagia. Oestrogen also mimics this effect: ovariectomy leads to increased food intake that can be reversed by E2 replacement.
Noteworthy among their interactions are the two hormone's effects on the regulation of each other's plasma levels. Such effects are exerted through indirect and direct pathways. Leptin influences E2 levels indirectly by modulating the regulation of the reproductive axis. It is well known that undernutrition inhibits female reproductive functions and lowers ovarian E2 in favour of self-maintenance and thermoregulation(Reference Otukonyong, Okutani and Takahashi221–Reference Brecchia, Bonanno and Galeati223). Low levels of ovarian E2 during fasting can be increased by eliminating leptin deficiency. Similarly, low E2 levels in ob/ob mice can also be increased by leptin(Reference Mystkowski and Schwartz165). Furthermore, leptin administration increases basal luteinising hormone levels, ovarian uterine weights and restores fertility in ob/ob mice(Reference Otukonyong, Okutani and Takahashi221). This suggests that leptin could be the adiposity signal to the reproductive axis signalling the body's current nutritional status, allowing reproduction to continue if significant adipose tissue is available and inhibiting it if body fat stores are depleted(Reference Barash, Cheung and Weigle224). The inhibiting effect of leptin deficiency on reproduction is mediated through the down-regulation of gonadotropin-releasing hormone(Reference Mystkowski and Schwartz165, Reference Bronson225).
Although gonadotropin-releasing hormone secretion appears as an important platform of leptin influence on oestrogenic effects, leptin may also influence cellular E2 availability directly. Leptin stimulates aromatase activity in adipose tissue and the presence of leptin receptors (Ob-R) in the ovary implies that leptin may have direct influence on ovarian E2 production and/or oestrogenic effects(Reference Mystkowski and Schwartz165).
There is evidence suggesting that leptin production is directly affected by E2. Ovariectomy or deletion of ERα increases serum leptin levels and leads to an increase of body fat mass(Reference Meli, Pacilio and Raso166). Plasma leptin levels are higher in women than in men with an equivalent fat mass and leptin secretion is higher in isolated female omental tissue than male donors, suggesting that sex steroids exert effects on leptin production. Women have higher subcutaneous tissue fat and less intra-abdominal fat than men. Therefore, this sex-related difference in fat distribution may account for these sex differences in leptin levels. Conversely, administration of E2 to cultured rat adipocytes induces leptin secretion and E2 receptors have been found in adipose tissue, suggesting that E2 exerts its effects over leptin secretion directly on adipocytes(Reference Mystkowski and Schwartz165).
In ovariectomised rats, the minimum effective leptin dose resulting in weight loss is greater than in controls, implying that E2 deficiency may attenuate leptin's effects(Reference Mystkowski and Schwartz165). A number of further studies confirm this attenuating effect of E2 over leptin and provide more insight into the potential mechanism. Meli et al. (Reference Meli, Pacilio and Raso166) found that changes in body weight and fat content caused by ovariectomy are accompanied by alterations in serum leptin concentration. Serum leptin levels are higher in ovariectomised than sham controls, and are reversed by E2 treatment. Increased serum leptin levels after E2 withdrawal are secondary to the increase in fat mass. Mid- or long-term hypo-oestrogenism causes a lower expression of Ob-R in adipose tissue and the hypothalamus compared with sham-operated rats. Lack of E2 leads to hypothalamic NPY overproduction and impairs central leptin sensitivity. The decrease in Ob-R and the associated leptinaemia support central leptin insensitivity. Oestrogen status, therefore, modulates serum leptin levels, as well as hypothalamic and white adipose Ob-Rb expression, thereby implying close cross-talk between central and peripheral leptin sensors in the regulation of body fat mass.
The above phenomenon was observed outside of the hypothalamus as well: Chen et al. (Reference Chen, Fan and Cui226) found a relationship between E2 levels and Ob-R expression in the dorsal root ganglion. Oestrogen was found to increase the expression of Ob-R protein and mRNA in ovariectomised rats. As a result of co-labelling for Ob-R and ERα/ERβ, 100 % co-localisation of ERα with Ob-R occurred, while ERβ–Ob-R co-localisation occurred in only 15 % of dorsal root ganglion neurons. The authors concluded that E2 may up-regulate the expression of Ob-R protein and mRNA in an ERα-dependent manner.
A study by Ainslie et al. (Reference Ainslie, Morris and Wittert227) confirmed that E2-deficient rats may be leptin insensitive. The authors showed that the effect of central leptin administration on food intake and physical activity in ovariectomised rats is significantly less than in sham controls. However, they did not find differences in Ob-Rb mRNA expression between sham and ovariectomised rats, suggesting that central leptin insensitivity may occur due to a defect at, or downstream to, the hypothalamic Ob-Rb itself.
Once leptin binds to Ob-Rb, the associated Janus kinase 2 tyrosine kinase is activated, followed by the activation of signal transducer and activator of transcription 3 protein (STAT3), STAT5, phospatidylinositol 3′-kinase and MAPK pathways. STAT3 is a crucial member of these pathways, as ablation of Ob-Rb–STAT3 signalling/neuronal STAT3/STAT3 in Ob-Rb neurons mimics the obesity, hyperphagia and decreased energy expenditure of leptin-deficient db/db mice. Following phosphorylation of STAT3 by Janus kinase 2 and the subsequent dimerisation and nuclear transport, STAT3 alters cell transcription and function. It was proposed that E2 may interact with the leptin pathway at the level of STAT3. STAT3 has been reported as a target of E2 signalling in cells. In a neural STAT3 knock-out murine model, mice exhibited obesity and infertility which E2 administration failed to correct(Reference Gao and Horvath182).
Oestrogen receptors are functionally linked to STAT3: they have a direct physical coupling and involve co-regulators such as cAMP-response element binding protein. STAT3 also enhances the transactivational function of oestrogen receptors, which suggests that oestrogen receptors and STAT3 act mutually as co-activators to facilitate signalling(Reference Gao and Horvath182).
Conversely, Chen & Heiman(Reference Chen and Heiman228) sought to determine whether ovariectomy leads to leptin resistance. Ovariectomy caused hyperphagia and increased fat mass. Leptin treatment increased fat utilisation and prevented reduction in energy expenditure. Withdrawal of leptin stimulated hyperphagia. The authors concluded that loss of ovarian function in rats is not associated with a change in leptin sensitivity.
It is well established that the hypothalamus plays an important role in the regulation of food intake and body weight since chemical or physical lesions of these regions cause hyperphagia and obesity(Reference Mystkowski and Schwartz165, Reference Gao and Horvath182). It seems clear at this point that the combined effects of leptin and E2 are exerted by their actions on the same population of hypothalamic neurons(Reference Chakraborty, Sachdev and Salton229). Evidence indicating that E2 and leptin may cooperate in the regulation of energy metabolism and reproduction is provided by the co-localisation of E2 and leptin receptors in hypothalamic neurons. Diano et al. (Reference Diano, Kalra and Sakamoto230) reported co-localisation of leptin receptors with ERα in the medial preoptic area, arcuate nucleus, ventromedial nucleus and paraventricular nuclei in female rats. The authors suggested that this extensive co-localisation of receptors in neuronal perikarya of the hypothalamus indicates a functional link between these hormones and the regulation of a variety of behavioural and neuroendocrine mechanisms. In a previous study, Meli et al. (Reference Meli, Pacilio and Raso166) also found considerable co-localisation of oestrogen receptors and Ob-R in hypothalamic nuclei.
The possible mechanisms through which E2 and leptin may cooperate in the brain include interrelated modifications of hypothalamic orexigenic and anorexigenic neuropeptides. In the hypothalamus, anorexigenic arcuate nucleus POMC neurons inhibit food intake through the release of the cleavage product melanocyte-stimulating hormone, while the orexigenic NPY neurons act against this to promote feeding(Reference Mystkowski and Schwartz165). When leptin signalling is diminished, or during fasting, POMC expression is reduced, while increasing levels of leptin stimulate POMC transcription through STAT3 activation(Reference Mystkowski and Schwartz165, Reference Gao and Horvath182). Mystkowski & Schwartz(Reference Mystkowski and Schwartz165) found that ovariectomy in rats leads to increased POMC expression in the arcuate nucleus, implying an adaptive response of POMC neurons to attenuate increases in body weight. Furthermore, decreased body weight following the administration of supraphysiological amounts of E2 in male rats is followed by the induction of arcuate nucleus POMC gene expression. Oestrogen-induced weight loss elicits a compensatory reduction of POMC gene expression. Therefore, increased melanocortin signalling does not mediate E2 effects on energy homeostasis.
Gao & Horvath(Reference Gao and Horvath182) claim, however, that E2 exhibits molecular and cellular effects on POMC similar to leptin in the arcuate nucleus. They state that both leptin and E2 alter the synaptic input on hypothalamic melanocortin neurons, inducing an increased POMC tone and decreased food intake and adiposity, allowing for the assumption that E2 and leptin regulate POMC expression by the induction of synaptic remodelling.
According to Hirosawa et al. (Reference Hirosawa, Minata and Harada231), the increased serum leptin levels did not up-regulate POMC expression in the hypothalamus of ERα-null mice, while ablation of E2 by ovariectomy and the accompanying higher serum leptin levels up-regulated POMC expression, indicating that ERα is essential for the up-regulation of POMC and that this pathway is probably mediated in an E2-independent manner. ERα can be transcriptionally activated by ligand-independent activation. The authors suggest that leptin may activate ERα through the MAPK pathway via Janus kinase 2. Others, however, suggest that E2 influences the distribution of body fat and adipose tissue metabolism via both ERβ and ERα: according to Shin et al. (Reference Shin, Hur and Seo232), obesity and blood leptin levels are influenced by the ERβ:ERα ratio.
It may be possible that E2 exerts its effects over POMC via a putative membrane-associated oestrogen receptor that is distinct from ERα and ERβ. Qiu et al. (Reference Qiu, Bosch and Tobias171) identified this receptor in dopamine-containing and POMC hypothalamic neurons; its activation leads to desensitisation of γ-aminobutyric acid (GABAB) receptors in POMC neurons of the arcuate nucleus by the phospholipase C, protein kinase C, protein kinase A pathway, facilitating the formation of synaptic contacts with hypothalamic areas controlling appetite by POMC neurons.
Interactions between insulin and leptin, oestrogen or thyroid hormones
Leptin, like insulin, plays an important and key role in food reward. The mechanisms are unclear but are being intensely investigated(Reference Konner, Klockener and Bruning233). Insulin is a major modulator of leptin levels(Reference Carvalheira, Siloto and Ignacchitti234–Reference Orosco, Rouch and Gerozissis239), in a dose-dependent manner(Reference Buyse, Viengchareun and Bado240–Reference Saladin, De Vos and Guerre-Millo242). In diabetic rats, insulin administration reverses the previously reduced leptin level(Reference Sivitz, Walsh and Morgan243). Hypothalamic neurons (predominantly in the arcuate nucleus) that express leptin receptors(Reference Fei, Okano and Li82) and insulin receptors(Reference Havrankova, Roth and Brownstein244–Reference Marks, Porte and Stahl246) widely overlap. In these neurons, the signalling pathways of leptin and insulin converge on PI3 kinase whose product, PIP3, regulates excitability of ATP-dependent K (KATP) channels, thereby controlling food intake, energy expenditure and glucose metabolism(Reference Shyng and Nichols247). The activation of KATP channels results in hyperpolarisation and thereby inactivation of glucose-responsive/insulin-sensitive neurons. It has been shown that the inhibition of PI3 kinase blocks the anorexigenic effects of both leptin and insulin in rats(Reference Gerozissis188). Insulin and leptin both target arcuate nucleus NPY neurons, where PI3 kinase-mediated signals seem to be shared and are common regulators in the two hormones' activating effects(Reference Yang, Wang and Wang248). Insulin, in interaction with serotonin and leptin, affects the activity of POMC neurons, inducing α-MSH release, which leads to a negative regulation of energy balance.
It has been reported that both ERα knock-out and aromatase knock-out mice are obese(Reference Heine, Taylor and Iwamoto249, Reference Takeda, Toda and Saibara250), but ovariectomised ERα knock-out mice display decreases in body weight, fat-pad weight, adipocyte size and improved insulin and glucose metabolism indicative of ERβ-mediated activity on adipose tissue which are opposite to those of ERα(Reference Naaz, Zakroczymski and Heine251). ERα plays a role in the development of hepatic insulin sensitivity(Reference Clegg, Brown and Woods252, Reference Geer and Shen253) since the absence of ERα in the ventromedial nucleus leads to weight gain and insulin resistance, adiposity, hyperphagia and hyperglycaemia(Reference Musatov, Chen and Pfaff172). It is well known that the insulin-activated GLUT-4 transporter is the main carrier for glucose into cells. In ERα knock-out mice, the activity of these transporters is substantially reduced; ERβ knock-out mice do not show this deficiency(Reference Barros, Machado and Warner254). Insulin–E2 interactions are also obvious in certain physiological or pathological conditions, such as pregnancy, menopause or diabetes. In pregnant rats, sexual steroid levels are increased, resulting in elevated insulin levels. Likewise, E2 administration in menopausal women corrects insulin secretion and decreases insulin resistance(Reference Brussaard, Gevers Leuven and Frolich255–Reference Ding, Song and Manson257). Cellular investigations demonstrate that ERα is also present in pancreatic β-cells, which is responsible for the short-term insulinotropic effect of E2 via inhibition of KATP channels.
A hypothesis has arisen that partially explains the synergistic interrelationship of insulin and T3 underlying mechanisms involved in glucose and lipid metabolism. According to this, T3 plays a more significant role in glucose metabolism and regulation than previously thought. T3 regulates glucose uptake, storage, glycolysis, as well as lipid oxidation and uptake in insulin-sensitive cells. Disturbances in T3 levels cause insulin resistance and hyperglycaemia, which is indicative of its contribution to deficiencies/pathogenesis leading to type 2 diabetes. T3 increases glucose uptake directly. Expression of (regulatory) glucose transport proteins, such as GLUT-1, GLUT-2 and GLUT-4 are regulated by both insulin and T3. A family of membrane-associated proteins regulated by T3, named β3-adrenergic receptors, plays a role in NEFA oxidation and insulin secretion. T3 and TAG in fat cells regulate insulin secretion and insulin sensitivity by an as yet unknown mechanism(Reference Groot, Jameson and DeGroot258–Reference Ohara, Kobayashi and Shiraishi266). Additionally, T3 regulates the expression of transmembrane enzymes K+/Na+ and Ca2+ ATP-ase, involved in driving membrane-bound protein pumps, which transport molecules such as glucose and amino acids into the cells(Reference Kyte267). Thyroid hormones reduce β-cell mass, the main source of insulin production, although several studies have demonstrated that thyroid hormones have cytotoxic effects on β-cells in vivo and in vitro. In fact, thyroid hormones induce apoptotic cell death in insulin-producing cells(Reference Lenzen and Bailey268–Reference Ximenes, Lortz and Jorns272).
Interactions between oestrogen and thyroid hormones
Although clinical reports point to a relationship between thyroid disorders and insulin sensitivity particularly prevalent in older populations of females more than males, the mechanisms which link sex steroids, thyroid hormones and metabolic homeostasis in both sexes are not well known(Reference Wu273). In postmenopausal women receiving E2 treatment, lower levels of free T3 and free T4 have been observed although there was no rise in serum TSH(Reference Franklyn, Sheppard and Ramsden274). Although E2 treatment did not alter the serum concentrations of TSH in euthyroid or untreated hypothyroid rats, it increased the T3 effect in serum TSH in hypothyroid rats. Oestrogen treatment increased thyroid hormone receptor expression in the pituitary, suggesting that an increase in thyroid receptors may be induced by E2 in rats(Reference Franklyn, Wood and Balfour275). During development, treatment with testosterone and E2 had similar effects in both sexes, producing lower levels of α and β subunit mRNA in exogenous sex steroid-treated hypothyroid rats compared with hypothyroids not receiving exogenous sex-steroid treatment. These data suggest that gonadal steroids render the pituitary thyrotroph cells more sensitive to thyroid hormones(Reference Ahlquist, Franklyn and Wood276). This mechanism between oestrogen receptor and thyroid hormone receptor interaction will be explored further, keeping in mind the relationship as it pertains to feeding, appetite, metabolism and energy balance modulated by sex hormones, particularly E2 in women.
Thyroid hormones affect reproductive behaviours of both males and females in addition to playing a permissive role in the photoperiodicity of both sexes(Reference Fitts, Klein and Powers277–Reference Dahl, Evans and Moenter279). Both thyroid hormones and sex steroids modulate metabolic homeostasis and oxidative metabolism at the tissue level via their respective non-genomic membrane and genomic nuclear receptors, sometimes acting synergistically between genomic and non-genomic mechanisms(Reference Vasudevan, Zhu and Daniel280–Reference Alaynick, Way and Wilson286) as described above. These mechanisms, in turn, feed back into the regulation of the hypothalamo–pituitary–adrenal axis, hypothalamo–pituitary–gonad axis, as well as the ‘hypothalamus–gut’ circuits(Reference Konturek, Konturek and Pawlik287–Reference Suzuki, Simpson and Minnion289). Additionally, thyroid hormones contribute to the maintenance of non-reproductive conditions, homeostasis in both male and female birds and mammals, for example, intracellular sites of activity including mitochondria, endoplasmic reticulum, plasma membrane, synapse(Reference Zhang and Lazar124, Reference Lazar290), lipogenesis, lipolysis, thermogenesis, growth, development, differentiation(Reference Zhang and Lazar124, Reference Oppenheimer and Schwartz291, Reference Oppenheimer, Schwartz and Lane292), mylenation of axons(Reference Farsetti, Mitsuhashi and Desvergne293) via nuclear recruitment of co-activators and co-repressors by ligand-bound nuclear receptors(Reference Vasudevan, Ogawa and Pfaff294). Mood and cognition in women are also affected by E2 and thyroid hormones – signals that underlie appetitive behaviour at converging pathways in the hypothalamic nuclei, brain stem and target organs of humoral and efferent signals(Reference Klieverik, Janssen and van Riel283–Reference Peppa, Koliaki and Nikolopoulos285, Reference Konturek, Konturek and Pawlik287, Reference Bauer and Whybrow295–Reference Scott, Wu-Peng and Yen297). Such mechanisms conduct cross-talk among isoforms of nuclear oestrogen receptors and thyroid hormone receptors in diverse tissues and in terms of genomic expression(Reference Vasudevan, Zhu and Daniel280, Reference Lazar290, Reference Schwartz, Lazar and Oppenheimer298–Reference Vasudevan, Davidkova and Zhu300). While it is tempting to envision an overlapping function between oestrogen receptor and thyroid hormone receptor subtypes and isoforms, different co-localisation studies indicate distinct non-overlapping functions(Reference Lazar290). In support of this idea, the phenotypes of the specific knock-out models suggest functions unique to specific isoforms(Reference Vasudevan, Ogawa and Pfaff294). On the other hand, the modulation of the signals arising from E2 and thyroid hormones converging must influence underlying behaviour, such as appetite, feeding, homeostasis and metabolism, concentrated in hypothalamic nuclei.
Two genes, Thrα and Thrβ, encode the mammalian nuclear receptors for T3, confirming that T3 acts directly on transcription. TRα1 (and TRα2)(Reference Oppenheimer and Schwartz291, Reference Vasudevan, Davidkova and Zhu300) is encoded by the Thrα gene, and TRβ1 and TRβ2 are encoded by the Thrβ gene; another TRβ3 isoform that may be rat specific(Reference Williams301). These receptors have a similar, if not identical, structure, containing a central DNA-binding domain and a C-terminus domain, which activates transcription upon ligand binding(Reference Flamant, Gauthier and Samarut302). Both oestrogen receptors and thyroid hormone receptors are ligand-dependent nuclear transcription factors that can bind to an identical half-site, AGGTCA, of their cognate hormone response elements, although generalisations of receptor cross-activity are not easily made. For example, co-transfection of a mutated TRα1 also inhibited the E2 effect without binding to the AGGTCA sequence(Reference Zhu, Yen and Chin303). Studies of inhibitory effects of liganded TRα1 on E2 induction suggest that oestrogen receptors and thyroid hormone receptors may interact to modulate E2-sensitive gene expression, in a ligand-dependent and isoform-specific manner such as for the hypothalamic preproenkephalin promoter(Reference Zhu, Yen and Chin303). However, in contrast to TRα, the isoforms TRβ1 and TRβ2 failed to inhibit E2-induced activation of the consensus ERE but, rather, attenuated E2-mediated transcriptional activation(Reference Scott, Wu-Peng and Yen297). Thus, oestrogen receptor and thyroid hormone receptor isoforms have modular protein structures with high homology in the central DNA-binding domain where they bind common enhancer elements (ERE) within the promoter sequence modulating gene transcription(Reference Vasudevan, Zhu and Daniel280, Reference Scott, Wu-Peng and Yen297, Reference Evans304–Reference Zhao, Lorenc and Stephenson309).
Thyroid hormone receptors and oestrogen receptors are members of the nuclear receptor superfamily, composed of forty-eight ligand-activated Zn-finger transcription factors involved in important physiological functions such as metabolism and energy homeostasis, carbohydrate and lipid metabolism in addition to development, reproduction, etc(Reference Evans304, Reference Mangelsdorf, Thummel and Beato305, Reference Sonodo, Pei and Evans310, Reference Jeong and Mangelsdorf311). Briefly, members of the superfamily of nuclear receptors reside in either the cytoplasm or nucleus of the cell. When ligands or small molecules bind to the cytoplasmic nuclear receptor complex, it undergoes conformational change, dimerisation and translocation to the nucleus where it binds to a sequence of DNA called the hormone response element. There the nuclear receptor complex then co-recruits or co-represses other proteins downstream at the promoter site where transcription factors are activated and changes in cell function ensue.
Specifically, half of the T3 consensus response element DNA sequence is identical to the oestrogen receptor response element (ERE); therefore thyroid hormone receptors bind to a consensus ERE(Reference Scott, Wu-Peng and Yen297, Reference Vasudevan, Davidkova and Zhu300, Reference Dellovade, Zhu and Krey306, Reference Dellovade, Zhu and Pfaff307, Reference Dellovade, Kia and Zhu312) on several receptor genes in the hypothalamus. Thyroid hormone receptors and oestrogen receptors are both present in rat hypothalamic nuclear extracts and bind to the promoter regions in the hypothalamic gene preproenkephalin, causing interations between liganded thyroid hormone receptors and oestrogen receptors that affect preproenkephalin transcription(Reference Bauer and Whybrow296, Reference Dellovade, Kia and Zhu312). Likewise, the oxytocin gene promoter has a composite hormone response element to which several members of this superfamily of nuclear receptors can bind to whereby thyroid hormones interfere with E2-stimulated oxytocin mRNA reducing E2-stimulated behaviour(Reference Vasudevan, Davidkova and Zhu300, Reference Dellovade, Zhu and Pfaff307). The ERE identified in the promoter region of the progesterone receptor is also bound by thyroid hormone receptors(Reference Scott, Wu-Peng and Yen297). Progesterone, important in reproductive behaviour, correlates with a rise in appetite in cycling women(Reference Asarian and Geary313). Thus, since T3 may reduce E2-dependent sexual behaviour through interactions between thyroid hormone receptors and oestrogen receptors in the nuclei of hypothalamic neurons which underlie sexual behaviour(Reference Zhu, Yen and Chin303), their interaction may modulate eating behaviour in women throughout the cycle by acting at the nuclear receptor superfamily concensus ERE as outlined above. Indeed, cursory searches for feeding and oestrogen receptors, thyroid hormone receptors, progesterone receptor, oxytocin and preproenkephalin return hundreds of papers altogether on feeding and each of the factors referred to above.
Over the past 30 years much has been explored on the interaction of thyroid hormones and E2 at regions of DNA co-activating and co-repressing genomic expression(Reference Zhu, Dellovade and Pfaff314) and the non-genomic membrane-initiated molecular mechanisms of hormone signalling(Reference Zhao, Lorenc and Stephenson309), i.e. the cross-talk that produces a synergy between activation and repression of genomic and non-genomic mechanisms(Reference Sonodo, Pei and Evans310). Using a reproductive behavioural assay of rats, i.e. lordosis, it has been shown that sex hormone-dependent hypothalamic gene expression and behaviour are influenced by synaptic inputs which interfere with oestrogen receptor-dependent neuroendocrine function and behaviour(Reference Zhu, Dellovade and Pfaff314). For example, the two different genes that code for TRα1 and TRβ have opposite effects on female sexual behaviours(Reference Lazar290, Reference Dellovade, Chan and Vennstrom308), whereas hypothalamic neurons that express E2-sensitive genes, for example, the preproenkephalin promoter, are inhibited by TRα1 in the ventromedial hypothalamus (VMH) but not the amygdala or caudate/putamen(Reference Zhu, Yen and Chin303, Reference Dellovade, Zhu and Pfaff307, Reference Sonodo, Pei and Evans310). High doses of thyroid hormones reduce lordosis behaviour of ovariectomised E2-treated female mice(Reference Morgan, Dellovade and Pfaff315) but in total pituitary RNA levels, dependent upon new protein synthesis, E2 induced increases while T3 inhibited E2 induction(Reference Alarid, Preisler-Mashek and Solodin316). Studies of sex specificity between E2 and thyroid hormones in the regulation of pituitary function found that pituitary weight and total cellular RNA levels were significantly decreased following ovariectomy in females but increased following gonadectomy in males without changes in pituitary DNA levels compared with intact rats. Oestrogen treatment produced a significant increase in pituitary RNA in ovariectomised females but not gonadectomised males, although concomitant administration of T3 blocked the increase in pituitary RNA in females(Reference Zhu, Dellovade and Pfaff314). In contrast, thyroid hormone inhibited ERα proteolysis in pituitary lactotropes, did not prevent induction of prolactin gene expression or proliferation and inhibited the ERE reporter gene, suggesting that other endocrine factors can indirectly modulate ERα activity(Reference Alarid, Preisler-Mashek and Solodin316).
Thyroid hormone receptor is known to bind an ERE which inhibits E2-dependent transactivation(Reference Adan, Cox and Van Kats317). Areas of the brain involved in reproductive functions and behaviour may play a role in other appetitive behaviours such as appetite and food seeking, namely the olfactory bulb, hippocampus and granular layer of the cerebellar cortex, where TRα1 and TRα2 transcript levels are highest. Feedback mechanisms common to reproduction and feeding/appetitive/energy homeostasis occur in hypothalamic nuclei. For example, such mechanisms exist in the paraventricular nuclei with hypophysiotrophic neurons projecting to the median eminence, and pre-autonomic neurons controlling autonomic projections to the liver(Reference Konturek, Konturek and Pawlik287, Reference Farsetti, Mitsuhashi and Desvergne293, Reference la Fleur, Kalsbeek and Wortel318, Reference Buijs, la Fleur and Wortel319), arcuate nucleus(Reference Klieverik, Janssen and van Riel283, Reference Scott, Wu-Peng and Yen297), supraoptic nucleus particularly the TRα(Reference Adan, Cox and Van Kats317, Reference Bradley, Young and Weinberger320), and adrenal afferents to the brainstem and suprachiasmatic nucleus via separate sympathetic and parasympathetic neuron populations(Reference Buijs, la Fleur and Wortel319). In addition, cross-talk between E2 and thyroid hormones modulates the activity in the hippocampus and amygdala contributing to cognition and mood, triggers for emotional eating in women(Reference Vasudevan and Pfaff282).
Nuclear receptors such as oestrogen receptors and thyroid hormone receptors bind low-molecular-weight ligands of E2, selective oestrogen response modulators, T3 and T4(Reference Fitts, Klein and Powers278, Reference DuSell, Umetani and Shaul321), where they co-activate and co-repress transcription factors at targeted genomic regions(Reference Alaynick, Way and Wilson286, Reference Evans304, Reference Mangelsdorf, Thummel and Beato305, Reference DuSell, Umetani and Shaul321–Reference Davis, Leonard and Davis323). However, E2 and thyroid hormones also bind to plasma membrane receptor sites initiating non-genomic, ion and intracellular Ca2+ channels, and G-protein-coupled secondary messenger cascades discussed above(Reference Ropero, Alonso-Magdalena and Quesada177, Reference Vasudevan, Kow and Pfaff281, Reference Vasudevan and Pfaff282, Reference Zhao, Lorenc and Stephenson309, Reference Sonodo, Pei and Evans310, Reference Glass, Holloway and Devary324). Future investigation into the regulation of metabolic homeostasis between the central nervous system and peripheral tissue may incorporate genomic analysis of the superfamily of nuclear receptors (oestrogen receptors and thyroid hormone receptors) in concert with the neuromodulatory factors outlined above.
Conclusion
The goal of the present review was to highlight the role of the complex hormonal interplay in the regulation of food intake and energy homeostasis. The hormones discussed in the present review are crucial in the regulation of food intake and energy homeostasis in that they play the most significant roles in the function of relevant central, mostly hypothalamic, circuits. However, they also act as the peripheral signals/mediators of peripheral tissues to inform hypothalamic circuits of the real-time status of the body's constituent energy level. Thus, it appears that ghrelin, leptin, thyroid hormones, insulin and E2 form a ‘second zone’ in the modulation of energy homeostasis (Fig. 1). This second zone lies between the central ‘hotspot’ of hypothalamic circuits and the peripheral tissues. The peripheral tissues, as appears from our review, represent a third, ‘outer ring/zone’ in this respect, where local interactions take place between the discussed hormones, on the one hand, and between the respective tissues and the trophic hormones, on the other hand. The apparent integrity of the discussed regulatory mechanisms suggests that the interpretation of hormonal effects alone or in simple combinations is not sufficient to understand their true nature, as both individual (feedback signals) and combined effects are necessary in the complex orchestration of energy homeostasis, including the regulation of food intake and energy expenditure. In other words, it seems most likely that the discussed hormones act as feedback signals, functioning as individual hormone signals, while their interactive effects propel and shape the bidirectional message between the central nervous system and the periphery. Our summary, therefore, points to the need for studies involving thorough, multifactorial investigation of the topic, including the analysis of simultaneous changes in the aforementioned variables from the central nervous system, as well as peripheral tissues of the experimental subjects.
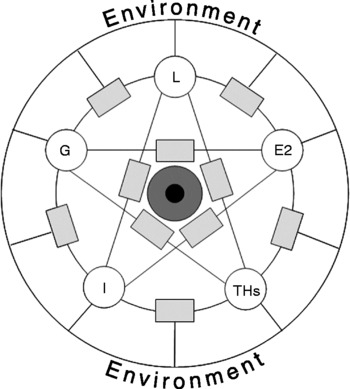
Fig. 1 Simplified scheme of interactions between leptin (L), ghrelin (G), oestrogen (E2), insulin (I) and thyroid hormones (THs). The hypothalamus (■) is in the centre of the neuroendocrine regulation of food intake, while functionally relevant brain regions () influence central hypothalamic functions. The listed hormones, as feedback signals, affect the hypothalamic regulation of their own and each other's secretion, while the secreted hormones regulate the functions of relevant peripheral organs (
) directly and through local hormone interactions as well. Peripheral organs, in their interactions with the inner and outer environment (outermost circle), modulate the humoral feedback factors to tune to adequate hypothalamic regulation. All together, the ‘pool’ of neuromodulators acts to invoke feeding behaviour or to inhibit it, thus maintaining the energy homeostasis of the organism.
Acknowledgements
Work on this project was greatly supported by Országos Tudományos Kutatási Alapprogramok (OTKA; Hungarian Scientific Research Fund) OTKA 72186 and OTKA 81745, Gazdaság és Versenyképesség Operatív Programjának (GVOP; Hungarian Economy and Competitiveness Programme) GVOP-3.2.1.-2004-0225/3.0., and the János Bolyai Research Scholarship of the Hungarian Academy of Sciences.
The authors' contributions were as follows: V. S. reviewed leptin; A. G. reviewed thyroid hormones; T. J. S. reviewed the interactions of oestrogens and thyroid hormones and edited the revised manuscript; D. S. K. reviewed insulin; G. G. reviewed ghrelin; T. B. reviewed the interactions of leptin and thyroid hormones; V. L. F. reviewed the interactions of ghrelin and leptin; A. Z. reviewed oestrogen and the interactions of oestrogen and leptin, and wrote the abstract. The introduction and conclusion were a joint effort by all authors.
The authors declare that they have no conflict of interest with regard to the present article.