Introduction
Magnetic nanocomposites (MNCPs) have attracted widespread attention in analytical and biomedical fields, especially in sample pretreatment and drug-delivery systems, because of their magnetic properties, large specific surface areas, large pore volumes, rapid adsorption kinetics, and adjustable pore sizes (He et al., Reference He, Yi, Zhang, Zhao and Yu2021; Shende & Shah, Reference Shende and Shah2021; Zhu et al., Reference Zhu, Wei, Chen, Gu, Rapole, Pallavkar, Ho, Hopper and Guo2013). In particular, the discovery of these nanomaterials has bestowed a tremendous impact on the development of analytical chemistry (Di et al., Reference Di, Ning, Yu, Chen, Yu, Wang, Yang and Zhu2020). Generally, magnetic nanocomposites are base nanomaterials with embedded magnetic particles, which are oxides of metals such as Fe, Co, Ni, and Cu (Kharissova et al., Reference Kharissova, Dias and Kharisov2015).Their superparamagnetism makes them ideally suited to overcome the challenges of time consumption of traditional solid-phase extraction (SPE) systems and to simplify dramatically the pretreatment procedure and improve the extraction efficiency by employing magnetic separation (Yu et al., Reference Yu, Wang, Hu, Li, Luo and Mei2019). Moreover, MNCPs have been developed to avoid the limitation of specific surface area and drawbacks of agglomeration which result in weakened and leaky magnetism of magnetic nanoparticles (MNPs) such as iron oxides when they have been used as sole particles (Rios et al., Reference Rios, Zougagh and Bouri2013).
Research has continued apace to search for and develop novel MNCPs as adsorbents which have large adsorption capacities. Carbon-based materials or porous materials with various morphologies have been embedded with MNPs to form MNCPs, e.g. graphene oxide (GO) (Farooq & Jalees, Reference Farooq and Jalees2020), carbon nanotubes (CNTs) (Gao et al., Reference Gao, Li, Morimoto, Nagaoka and Maekawa2006), and metal–organic frameworks (MOFs) (Kurmoo, Reference Kurmoo2009). All these nanocomposites not only have superparamagnetic properties, large specific surface areas, and large adsorption capacities but also enhanced recovery from the reaction medium (Farooq & Jalees, Reference Farooq and Jalees2020). However, their cost and toxicity are major concerns. To be specific, GO and CNTs should not be applied without carboxylation, and their significant toxicity could be addressed by biocompatible polymer modification (Itatahine et al., Reference Itatahine, Mehdi, Fizir, Qi, Dramou and He2017). In addition, the aforementioned nanomaterials are not available in nature, which means they are produced using specialized experimental protocols which are laborious and time consuming. To overcome these problems, researchers have sought to develop alternative low-cost nanomaterials that possess comparable capacities to CNTs or GO. Recently, as a low-cost and naturally available biocompatible material, halloysite nanotubes (HNTs) have attracted rapidly growing interest (Li et al., Reference Li, Yuan, Jiang, Dai, Zhao, Guan, Bai and Wang2022).
Nanosized tubular halloysites are naturally hollow, multi-walled tubular structures. Transmission electron microscopy (TEM) analysis showed that the length of HNTs is within the micrometer range (0.4–1 μm), the inner lumen diameters are 10–70 nm, and the outer diameter is 20–200 nm (Yu et al., Reference Yu, Wang, Zhang, Zhang and Liu2016). The unitary cell formula is Al2Si2O5(OH)4.nH2O. HNTs have large porosity, enlarged volume and surface area, and plenty of hydroxyl groups available for modification (Zhang et al., Reference Zhang, Tang, Yang and Ouyang2016). In addition, HNTs are available and abundant in nature, non-toxic, biocompatible, and cheap (Yendluri et al., Reference Yendluri, Lvov, de Villiers, Vinokurov, Naumenko, Tarasova and Fakhrullin2017). HNTs are composed of a gibbsite-like array of aluminol (Al–OH) groups in the inner space of the lumen, and siloxane (Si–O–Si) groups on the outer surface (Fig. 1a) (Fizir et al., Reference Fizir, Dramou, Nasiru Sintali, Ruya, Huang and He2018a). This distinctive structural composition offers HNTs a negatively charged outer surface and a positively charged inner lumen, over a broad range of pH values (Joussein et al., Reference Joussein, Petit, Churchman, Theng, Righi and Delvaux2005; Lvov et al., Reference Lvov, Shchukin, Mohwald and Price2008). The unique surface chemistry of HNTs can be similarly anchored with MNPs such as Fe3O4 to yield a promising framework for magnetic nanoparticles (Duan et al., Reference Duan, Liu, Chen, Zhang and Liu2012). In recent years, magnetic halloysite nanotubes (MHNTs), derivative nanocomposites from low-cost halloysites, have shown promise for future use in magnetic solid-phase extraction for the enrichment of various analytes from environmental, biological, and food samples. For example, coating HNTs with MNPs enhanced the removal capacity of heavy metals such as arsenic, cadmium, and lead (Maziarz & Matusik, Reference Maziarz and Matusik2017). The trend in terms of published articles about MHNTs since their discovery is shown in Fig. 1b. Most of the cited articles have been published since 2018. Obviously, research on MHNTs is still in its infancy and there seem to be no specialized reviews about the methods of preparation of MHNTs or their analytical applications. Therefore, a summary review on magnetic halloysite nanotubes from preparation to application is of importance in this field.

Fig. 1 a Schematic illustration of the crystalline structure of HNTs, and b Growth trend of number of articles on MHNTs published during 2010–2022 (data analysis done in September 2022)
Indeed, many similar reviews have been published on the subject of the properties and application of halloysite in various fields including analytical chemistry and in environmental and biomedical applications (Fakhrullin & Lvov, Reference Fakhrullin and Lvov2016; Fizir et al., Reference Fizir, Dramou, Nasiru Sintali, Ruya, Huang and He2018a, Reference Fizir, Richa, He, Touil, Brada and Fizir2020; Lvov et al., Reference Lvov, DeVilliers and Fakhrullin2016; Naumenko et al., Reference Naumenko, Guryanov, Yendluri, Lvov and Fakhrullin2016), where the common methods of preparation of magnetic halloysite nanotubes were highlighted. However, detailed synthesis strategies, functionalization, and application of HNTs combined with magnetic nanoparticles have not been reviewed. The present review starts with the synthetic strategies of MHNTs. Coating HNTs with MNPs can be categorized into in situ growth, nano-encapsulation, and direct-mixing methods. Some novel methods are presented for the first time. The advantages and limitations of each approach are summarized critically and, based on the authors’ understanding of the subject, some solutions for preparing MHNTs with superior characteristics are recommended. Then, the structural characteristics of synthesized MHNT nanocomposites are described briefly. Furthermore, functionalization approaches of MHNTs with various kinds of organic and inorganic molecules such as polymers, silane agents, and ionic liquids are highlighted. After that, the analytical applications of designed MHNT nanocomposites are summarized. In this part, the focus is on the application of composites as magnetic adsorbents in magnetic solid phase extraction (MSPE) for the separation of antibiotics, pesticides, proteins, carcinogens such as polycyclic aromatic hydrocarbons (PAHs), dyes, radioactive ions, and heavy-metal ions such as Cu(II), Cd(II), Pb(II), Hg(II), Cr(III), and Cr(VI) in complex matrices (Fig. 2a). Finally, current challenges and speculation about the potential ongoing exploration of MHNT nanocomposites for analytical achievements are discussed.

Fig. 2 a Preparation, properties, and functionalization of MHNT composites and their analytical applications, and b summary of the synthesis methods for MHNT composites
Preparation methods of MHNTS
The magnetic nanoparticles used most commonly for the preparation of MHNTs are metals, metal oxides, or ferrites such as ferromagnetic or superparamagnetic iron oxides [e.g. magnetite (Fe3O4) and ferric oxide (γ-Fe2O3)] (Majidi et al., Reference Majidi, Zeinali Sehrig, Farkhani, Soleymani Goloujeh and Akbarzadeh2016). MHNT particles are produced mainly by chemical synthesis which includes various processes such as co-precipitation, in situ precipitation, wet impregnation, solvothermal, egg-white solution, nano-encapsulation, and direct-mixing methods (Fig. 2b ). All the above-mentioned methods except loading and direct-mixing approaches are in situ growth methods because the formation of MNPs is carried out in the presence of the HNTs. Among them, chemical co-precipitation is the most commonly employed method, and its synthetic methods are steerable under mild conditions (Chen et al., Reference Chen, Zhou, Fiore, Tong, Zhang, Li, Ji and Yu2016).
Chemical Co-precipitation Method
Co-precipitation is a simple, controllable, and low-cost technique (He et al., Reference He, Yi, Zhang, Zhao and Yu2021). The method consists of dissolving a stoichiometric mixture of iron salts (e.g. FeCl3.6H2O and FeSO4.7H2O or FeCl2.4H2O) in deionized water, introducing a pre-treated HNT dispersion, and adjusting the dispersion medium in the pH range of 9–13 with base (Eq. 1) in the presence of nitrogen gas to prevent the critical oxidation of Fe3O4 into γ-Fe2O3 (Eq. 2) (Fizir et al., Reference Fizir, Dramou, Nasiru Sintali, Ruya, Huang and He2018a; Konnova et al., Reference Konnova, Lvov and Fakhrullin2016). In this method, the nucleation and growth of magnetic nanoparticle nuclei occur in situ in an aqueous dispersion of HNTs (López-López et al., Reference López-López, Durán, Delgado and González-Caballero2005). Thus, the iron oxide nanoparticles precipitate with HNT particles and form Fe3O4-HNTs nanocomposites. The mechanism of Fe3O4-HNT formation is based on the electrostatic interaction between MNPs and HNTs where the cationic-metal ion precursors of Fe3O4 nanoparticles become attached to the negatively charged HNTs (Abhinayaa et al., Reference Abhinayaa, Jeevitha, Mangalaraj, Ponpandian, Vidhya and Angayarkanni2018). Another explanation is that the metal ions could be adsorbed and retained in the large pore volume of HNTs (Afzali & Fayazi, Reference Afzali and Fayazi2016). By using the co-precipitation method, a high yield of MHNTs could be obtained. However, the main drawbacks of this method are the uncontrollable size distribution and the possibility of leakage of MNPs, which are challenging issues (Chen et al., Reference Chen, Zhou, Fiore, Tong, Zhang, Li, Ji and Yu2016). For example, MHNTs have been prepared with MNPs ranging in size from 10 to 16 nm (Fizir et al., Reference Fizir, Dramou, Zhang, Sun, Pham-Huy and He2017, Reference Fizir, Wei, Muchuan, Itatahine, mehdi, He and Dramou2018b; Li et al., Reference Li, Zha, Niu, Hu, Hui, Tang, Fizir and He2018a). In a study conducted by Liang et al. (Reference Liang, Chen, Xian, Hu, Hou, Wang, Wu and Wang2021), the size of Fe3O4 attached to HNTs nanoparticles was ~ 20–50 nm where a smaller subunits comprising MNPs of ~ 10 nm were found by Xie et al. (Reference Xie, Qian, Wu and Ma2011). These different MNP sizes can be explained as follows: nuclei should be isolated during the period of growth to obtain a very narrow size distribution of the monodispersed NPs produced. Evidently, in the system containing MNP and HNT dispersions, the two phases cannot be separated easily. Worth mentioning is that for MSPE application, the size of MNPs attached to HNTs is not very important as in pharmaceutical (drug-delivery system) or catalysis applications where the size of the metal NPs should be controlled precisely (Gao et al., Reference Gao, Lyu and Yin2020). Consequently, the method of choice may depend on the target application of the nanocomposites. Another point that researchers should be aware of during synthesis of MHNTs is the mass ratio of the total iron salts (Fe2+: Fe3+) to HNTs. A large amount of Fe3O4 particles could aggregate together when the mass ratio is > 3:1. In addition, when the ratio 2:1 or 3:1 is used, a unique cactus-like Fe3O4-HNT is produced. The acicular Fe3O4 on HNTs can develop into granular Fe3O4 as the time is extended to 24 h rather than 4 h (Song et al., Reference Song, Zhou, Zhang, Chen and Yang2019). In addition, the high ratio can have a negative effect on the adsorption of analytes (Wan et al., Reference Wan, Zhan, Long, Zeng and He2017). In order to satisfy the goal of magnetic separation without affecting the adsorption capacity of the nanocomposites, a ratio in the range of 1:1 to 3:1 and 4 h of agitation to prepare MHNTs is recommended. Agitation speed is also an important parameter that can affect the final size of MNPs and needs to be controlled during the preparation of MHNTs.


In Situ reduction-precipitation Method
The MHNTs are synthesized via in situ reduction of metal precursors on the surface of HNTs (Jia et al., Reference Jia, Ma, Rahman and Ma2011; Kadam et al., Reference Kadam, Shinde, Ghodake, Saratale, Saratale, Sharma, Hyun and Sung2020b; Lee et al., Reference Lee, Lee, Jee, Sung and Kadam2019). For magnetic materials, metal precursors, e.g. FeCl3, bind with HNT surfaces for electrostatic interactions between positive charges of metal ions and external negatively charged HNT. With the help of reductants such as Na2SO3 and NaBH4, magnetic nanoparticles were synthesized on HNT surfaces in situ (Jee et al., Reference Jee, Kim, Shinde, Ghodake, Sung and Kadam2020; Jiang et al., Reference Jiang, Zhang, Wei, Tjiu, Pan, Chen and Liu2014b; Kim et al., Reference Kim, Jee, Sung and Kadam2018; Lee et al., Reference Lee, Lee, Jee, Sung and Kadam2019). The formation strategy was that some Fe3+ ions in the solution were reduced to Fe2+ by Na2SO3 or NaBH4. Finally, the iron oxide nanoparticles were formed homogeneously with the addition of base (NaOH). This method is simpler, faster, and cheaper (Jee et al., Reference Jee, Kim, Shinde, Ghodake, Sung and Kadam2020; Jiang et al., Reference Jiang, Zhang, Wei, Tjiu, Pan, Chen and Liu2014b; Kim et al., Reference Kim, Jee, Sung and Kadam2018; Lee et al., Reference Lee, Lee, Jee, Sung and Kadam2019). Note that the sizes of MNPs prepared by this method were difficult to control (Table 3) (Li et al., Reference Li, Huang, Huang, Lin and Huang2020a) and that the use of reductants may increase the cost of the method.
Wet Impregnation Method
Wet impregnation, employing a physical mixture of the HNTs in a solid state and the MNPs dissolved in a liquid solution, is the simplest method for loading a given porous support as HNT (Tušar et al., Reference Tušar, Kaucic, Logar and Suib2013). This method consists of a dispersion of a known amount of HNT with a metal ion precursor (e.g. Fe(NO3)3·9H2O) in the solvent. After drying and removing the excess solvent, e.g. ethanol or methanol, the solid powder obtained is impregnated with ethylene glycol and subjected to thermal treatment (calcination) under a nitrogen atmosphere (Cheng et al., Reference Cheng, Dai, Chen, Cui, Qiang, Sun and Dai2019; Hang et al., Reference Hang, Li, Pan, Li, Dai, Dai, Yu and Feng2013; Ma et al., Reference Ma, Zhou, Dai, Qin, Ye, Chen, Xie, Yan and Li2016a; Tsoufis et al., Reference Tsoufis, Katsaros, Kooi, Bletsa, Papageorgiou, Deligiannakis and Panagiotopoulos2017). The formation process is that the positive Fe2+ and Fe3+ ions are introduced onto HNT surfaces through complexation reactions under agitation and Fe3O4 nanoparticles (or Cu/Co Fe2O4 NPs) are loaded into HNTs after high-temperature calcination (Hang et al., Reference Hang, Li, Pan, Li, Dai, Dai, Yu and Feng2013; Maleki et al., Reference Maleki, Hajizadeh and Salehi2019; Pan et al., Reference Pan, Hang, Dai, Dai, Huo and Yan2012a; Zhang et al., Reference Zhang, Lai, Wu, Li and Hu2020). The average size of the Fe3O4 NPs ranged from 5 to 36 nm (Table 1) (Dai et al., Reference Dai, Wei, Cao, Zhou, Yu, Pan, Zou, Li and Yan2014; Gan et al., Reference Gan, Huang, Zhang, Pan, Shi and Yan2015; He et al., Reference He, Zou, Chen, Dai, Xie, Zhou and Yan2016a, Reference He, Chen, Zhang, Hu, Wang and Wang2016b; Tsoufis et al., Reference Tsoufis, Katsaros, Kooi, Bletsa, Papageorgiou, Deligiannakis and Panagiotopoulos2017; Zeng et al., Reference Zeng, Sun, Wang, Wang and Yang2016).
Table 1 Synthesis conditions, saturation magnetization (Ms), and the size of MNPs of the MHNTs prepared using wet impregnation
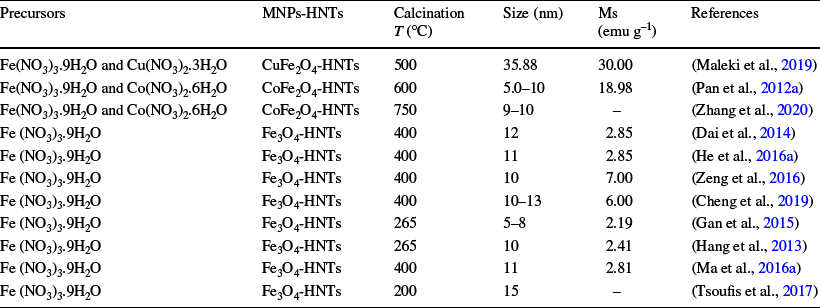
It is widely recognized that the pore size of HNTs decreased with increasing temperature (>300°C) (Mu et al., Reference Mu, Wang, Zhang and Wang2014a). It is difficult, therefore, to insert metals and metal oxides into HNT lumens without damaging the structure of the HNT (Table 3). This drawback may be resolved by decreasing the calcination temperature to <300°C and increasing the calcination time.
Solvothermal Method
The solvothermal process is considered to be among the most promising approaches to producing MHNTs. The thermal decomposition of the precursor is the principle of the method (Fizir et al., Reference Fizir, Dramou, Nasiru Sintali, Ruya, Huang and He2018a). This technique consists of dissolving ethylene glycol (EG) as an organic solvent with a specific percentage of the iron precursor solution (i.e. FeCl2 or FeCl3), dispersing HNTs in this solution, and adjusting the pH through the addition of an alkaline solution. Then, the mixture may undergo crystallization in a high-temperature and pressure reactor (Mirbagheri & Sabbaghi, Reference Mirbagheri and Sabbaghi2018; Pan et al., Reference Pan, Yao, Xu, Ou, Huo, Li and Yan2011, Reference Pan, Hang, Dai, Dai, Huo and Yan2012a). In the solvothermal preparation, MHNTs are synthesized via a Teflon-lined stainless steel autoclave under high temperature of 195–200°C and pressure of 3 × 10–9–4 × 10–8 bar (Jia et al., Reference Jia, Zhou, Xu, Li, Dong, Huang and Xu2016, Reference Jia, Zhou, Xu, Li, Xu, Zhang, Guo, Shen and Zhang2017; Tian et al., Reference Tian, Wang, Tian, Zhou, Yang and Komarneni2016; Zhou et al., Reference Zhou, Jia, Luo, Xu, Chen, Ge, Ma, Chen and Zhu2016). The formation mechanism is that Fe3+ or Fe2+ ions are adsorbed on the surface of HNTs through electrostatic attraction and then reduced to Fe3O4 or γ-Fe2O3 by ethylene glycol.
High-temperature conditions are beneficial for improving magnetism, which are the main advantage of this synthesis technique. In addition, the properties of the MHNTs, including size, density, and crystallinity of MNPs, can be changed through modification of the solvothermal reaction conditions such as temperature, time, concentrations, and the ratio of reactants (He et al., Reference He, Yi, Zhang, Zhao and Yu2021). The average size of the γ-Fe2O3 and Fe3O4 NPs attached to HNTs prepared by this method range from 20 to 200 nm (Table 2) (Mirbagheri & Sabbaghi, Reference Mirbagheri and Sabbaghi2018; Pan et al., Reference Pan, Wang, Dai, Dai, Hang, Ou and Yan2012b). Although this method forms a tunable morphology of MNPs attached to HNTs with high magnetism, it is expensive (Table 3).
Table 2 Synthesis conditions, saturation magnetization (Ms), and the size of MNPs of the MHNTs prepared by the solvothermal method
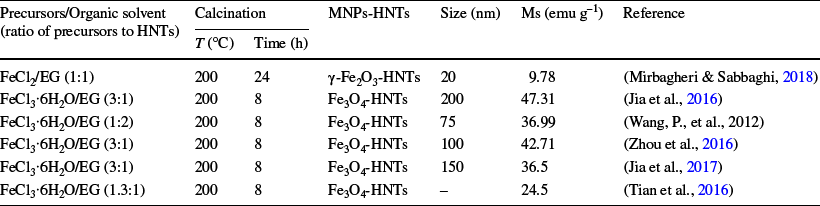
Table 3 Summary of the characteristics of common synthetic strategies of MHNTs (Chen et al., Reference Chen, Zhou, Fiore, Tong, Zhang, Li, Ji and Yu2016; Li et al., Reference Li, Huang, Huang, Lin and Huang2020a; Mu et al., Reference Mu, Wang, Zhang and Wang2014a)
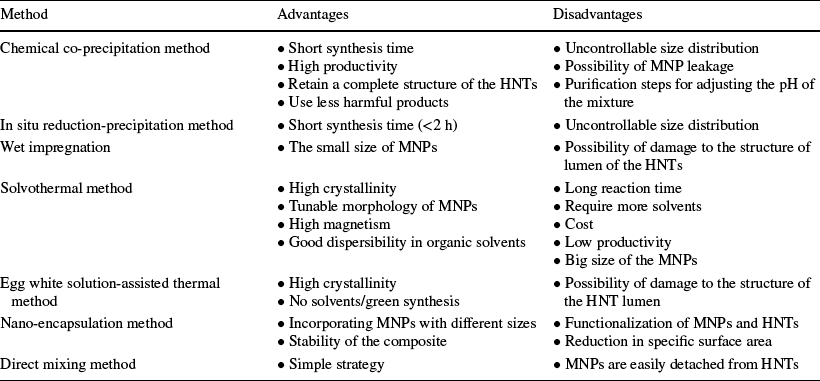
Egg-White Solution-assisted Thermal Method
A cost-effective method is to use egg white solutions to synthesize NiFe2O4-HNTs nanoparticles. This process consists of dispersing Ni(NO3)2.6H2O, Fe(NO3)0.9H2O, and freshly extracted egg white (ovalbumin) as precursors and HNTs in an aqueous medium. Afterward, the resulting dried precursors were calcined at 700°C to form desired crystalline NPs (Zare Pirhaji et al., Reference Zare Pirhaji, Moeinpour, Mirhoseini Dehabadi and Yasini Ardakani2020a, Reference Zare Pirhaji, Moeinpour, Mirhoseini Dehabadi and Yasini Ardakani2020b). Egg white is used due to its aqueous solubility and its ability to bind with metal ions (Gabal, Reference Gabal2010). Therefore, egg-white solution acts as a good surfactant to reduce the impurities in the prepared composites (Gabal et al., Reference Gabal, El-Shishtawy and Al Angari2012; Maensiri et al., Reference Maensiri, Masingboon, Boonchom and Seraphin2007). There are two main limitations of the aforementioned methods based on in situ growth of MNPs: one is that the selected HNTs must remain stable under the precursor mixture of Fe3O4 nanoparticles; the other is that it is possible to generate free MNPs in solution (Table 3).
Nano-Encapsulation Method
Coating MNPs onto the mesoporous HNTs could also be achieved by a nano-encapsulation-based approach. This method uses mainly functionalization of the inner lumen of HNT to promote the growth of MNPs into the inner channels to prepare MHNTs (first approach) or loading modified MNPs by a vacuum method (second approach). In the first approach, HNTs are modified to make the alumina core hydrophobic. Metal oleate (Zhang et al., Reference Zhang, Liu, Yan, Ye and Chen2014), tetradecylphosphonic acid (Hamza et al., Reference Hamza, Ferretti, Innocenti, Fidecka, Licandro, Sangregorio and Maggioni2020), and urease (Zheng et al., Reference Zheng, Du and Ma2015) are used for hydrophobic modification. Once the iron ions diffuse into HNTs, Fe3O4 grows in the HNTs to obtain Fe3O4-HNTs nano-encapsulates. Metal oleate is used as a ferrite precursor and for the hydrophobic modification of HNTs also. By using this strategy, the metal oleate complex entered into the inner space of the HNTs, and ferrite nanoparticles are then formed in situ during the subsequent heat treatment (Zhang et al., Reference Zhang, Liu, Yan, Ye and Chen2014). HNTs are loaded with urease and impregnated metal ion precursor with urea solution. The loaded urease hydrolyzed urea to produce NH3 and iron ions are grown in situ (Fig. 3a) (Zheng et al., Reference Zheng, Du and Ma2015).
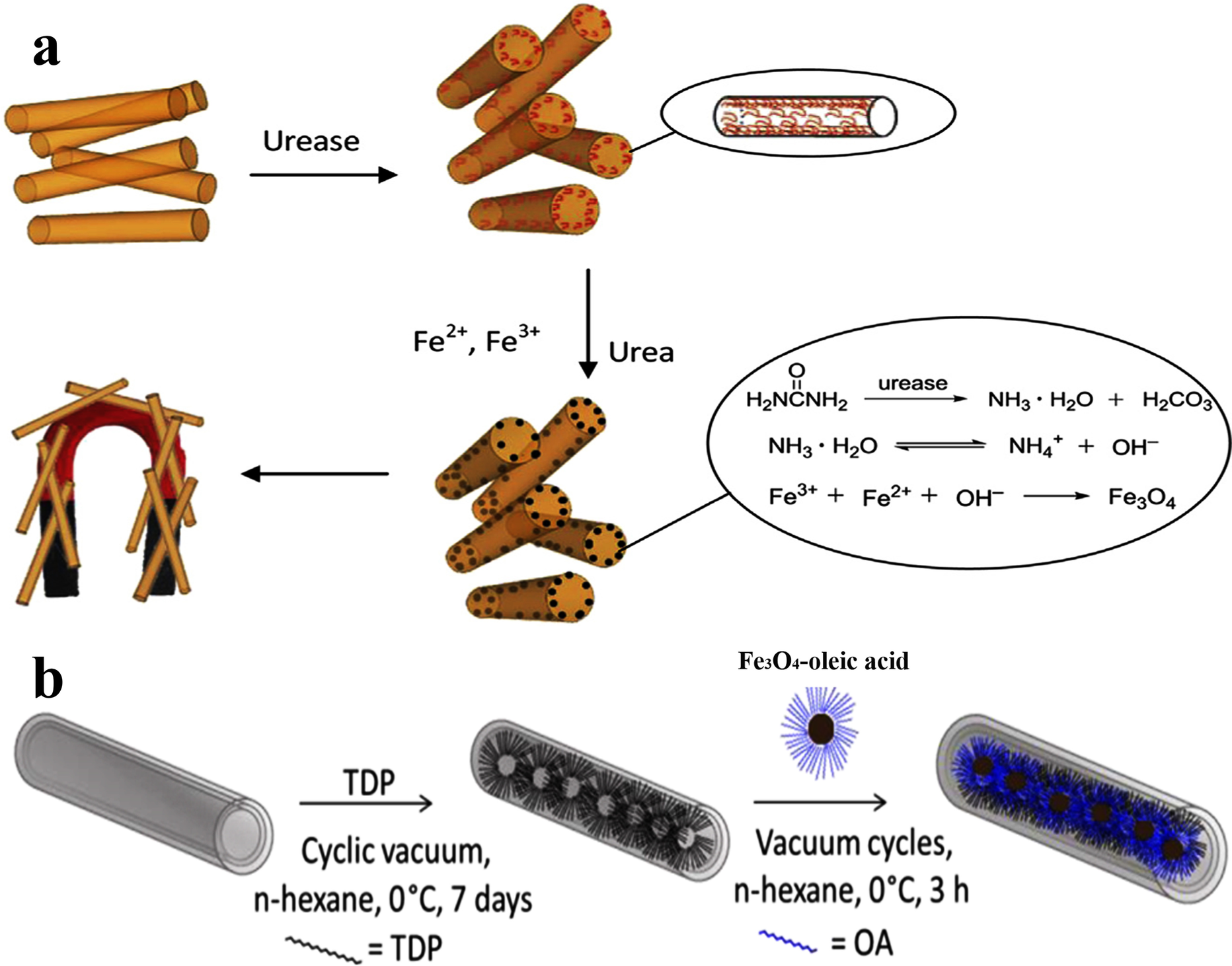
Fig. 3 Encapsulation approaches of Fe3O4 into the lumens of HNTs: a urease immobilization and Fe3O4 formation in the lumens of HNTs (reproduced from Zheng et al., (Reference Zheng, Du and Ma2015) with the permission of Elsevier), and b selective loading of Fe3O4 in the inner part of HNTs (reproduced from Hamza et al.(Reference Hamza, Ferretti, Innocenti, Fidecka, Licandro, Sangregorio and Maggioni2020) with permission of the ACS)
In the second approach, the prepared MNPs of diameter ∼6 nm are functionalized and subsequently added to the modified HNTs by using repeated vacuum/N2 cycles while stirring. By using this strategy, all MNPs can be loaded into the lumen of HNTs without any relevant interaction with the HNT outer surface (Fig. 3b) (Hamza et al., Reference Hamza, Ferretti, Innocenti, Fidecka, Licandro, Sangregorio and Maggioni2020). This method not only has the unique advantage of incorporating MNPs with various sizes but also enables the construction of MNCPs in a controllable manner, and their migration could be suppressed significantly due to the spatial confinement which accounts for high composite stability. The main drawback of this approach is the time consumed in the functionalization of both guests – HNTs and MNPs (Table 3).
Direct Mixing Method (self-assembly)
Mixing HNTs and MNPs directly is a simple strategy to design MHNTs which is completed by the self-assembly of MNPs on the surfaces of HNTs (Khunová et al., Reference Khunová, Šafařík, Škrátek, Kelnar and Tomanová2016, Reference Khunová, Pavliňáková, Škrátek, Šafařík and Pavliňák2018) or vice versa, which means the attachment of HNTs on the surface of iron oxide microspheres depends on the size of the MNPs (Duan et al., Reference Duan, Yuan, Guo, Niu, He and Xia2021). Uniform mixing of previously functionalized or prepared MNPs and HNTs is carried out under ultrasonication or stirring (Duan et al., Reference Duan, Yuan, Guo, Niu, He and Xia2021; Pan et al., Reference Pan, Zhu, Dai, Yan, Gan, Li, Hang and Yan2014).The preparation of MHNTs by direct mixing relies on electrostatic interactions and covalent bonding. Based on this, a halloysite-encapsulated magnetic microsphere composite is prepared by ultrasonification of the mixture of Fe3O4-SiO2 and Cu-HNTs (Fig. 4a). The silica layer is employed commonly to coat MNPs in the mixing method, which can greatly improve the reactivity of MNPs and the stability of MHNTs (Duan et al., Reference Duan, Yuan, Guo, Niu, He and Xia2021). The self-assembly of Lys-Fe3O4 on the surface of Ag/HNTs is carried out by a simple mixing technique. Lys-Fe3O4 nanoparticles with the protonated amino groups are easily embedded in the surface of Ag/HNTs via electrostatic interaction (Fig. 4b). Ag/HNTs/Fe3O4 nanocomposites show high saturation magnetization values of 24.27 emu g–1 (Mu et al., Reference Mu, Wang, Zhang and Wang2014a). The same strategies are followed to prepare Au/HNTs/Fe3O4 (Mu et al., Reference Mu, Zhang and Wang2014b). In other research, poly glycidyl methacrylate-grafted MNPs (MNPs-g-PGMA) are deposited on the surfaces of HNT-NH2 by a simple mixing technique. The formation mechanism is based on the chemical reaction between epoxy rings of the PGMA chains and amino groups of the HNTs (Nguyen et al., Reference Nguyen, Cao, Park and Lim2017). The method of direct mixing of Fe3O4 NPs and hydrothermally carbonized glucose on HNTs (HNTs-Glu) was developed recently. HNTs-Glu-Fe showed high superparamagnetic properties of 50.7 emu g–1 (Sadjadi et al., Reference Sadjadi, Lazzara, Heravi and Cavallaro2019).
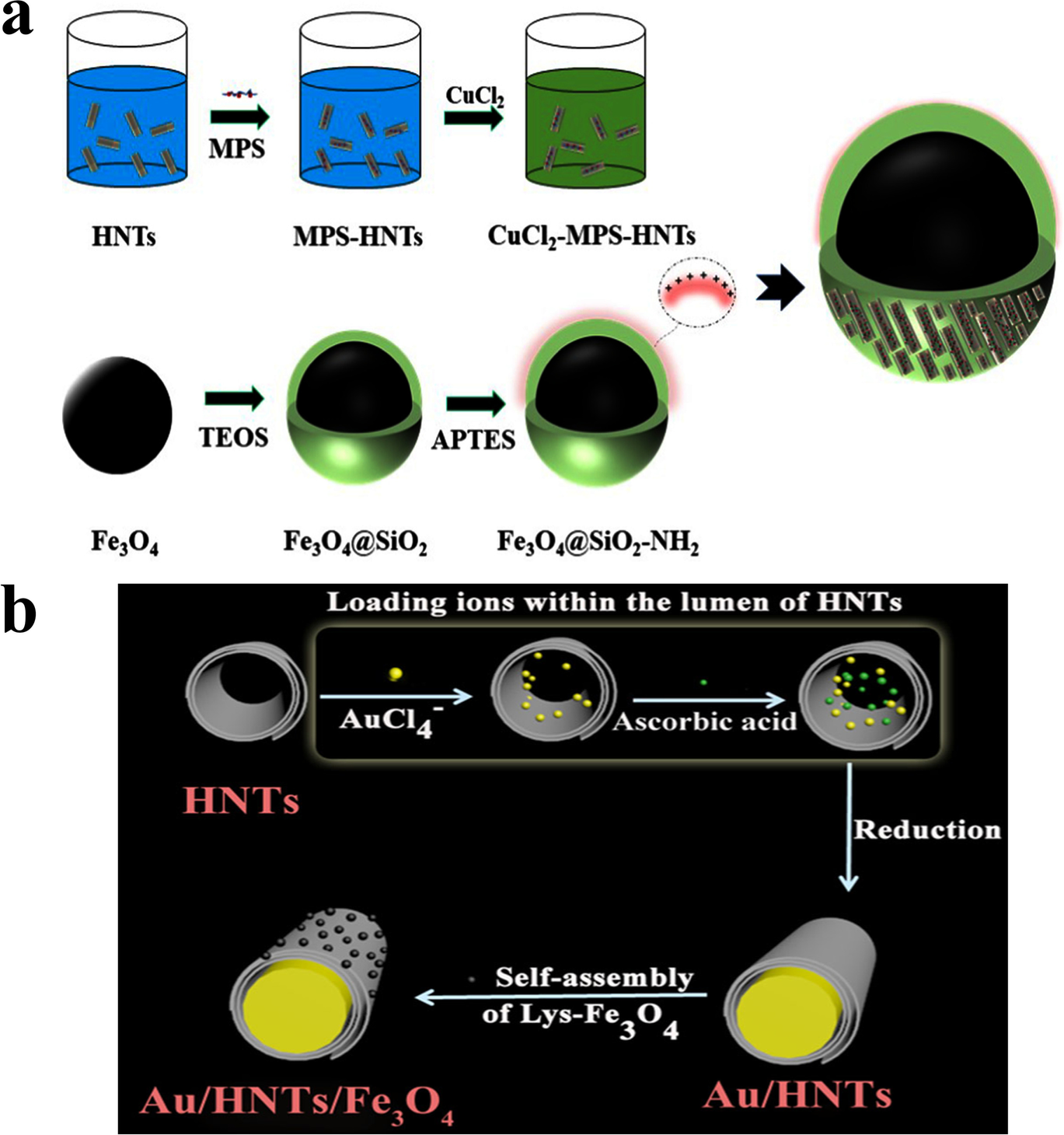
Fig. 4 MHNTs prepared using direct mixing methods: a the preparation process of Fe3O4@SiO2@Cu-MHNTs (reproduced from Duan et al. (Reference Duan, Yuan, Guo, Niu, He and Xia2021) with the permission of Elsevier) and b schematic illustration of the preparation of Au/HNTs/Fe3O4 (reproduced from Mu et al., Reference Mu, Wang, Zhang and Wang2014a with the permission of the Royal Society of Chemistry)
Structural Characterizations of MHNTs
Various physicochemical identification techniques have been used to examine the structural and distinctive features of the synthesized MHNTs, e.g. Fourier-transform infrared spectroscopy (FTIR), Raman spectroscopy, X-ray diffraction (XRD), vibrating sample magnetometry (VSM), energy-dispersive spectrometry (EDS), transmission electron microscopy (TEM), and scanning electron microscopy (SEM). FTIR spectroscopy is used to confirm the presence of expected functional groups. The absorption peak at 1030 cm–1 is from the Si–O groups in MHNTs. The peaks at 536 and 912 cm−1 are characteristic of Al–O–Si and the internal hydroxyl group vibrations, respectively, in magnetic nanotubes. The peak at 3430 cm–1 is due to structural O–H stretching vibrations from iron oxides of MHNTs. The FTIR analyses showed that the bands of the Al–O–Si of HNTs at 536 or 540 cm–1 and characteristic MNP peaks at 580 cm−1 are overlapped in MHNTs (Amjadi et al., Reference Amjadi, Samadi and Manzoori2015a; Fizir et al., Reference Fizir, Dramou, Zhang, Sun, Pham-Huy and He2017; Hajizadehet al., Reference Hajizadeh, Hassanzadeh-Afruzi, Jelodar, Ahghari and Maleki2020a). To identify the crystalline structure of MHNTs, XRD examinations are used. The XRD traces display distinct peaks in the range 30–65°2θ which can be indexed as (220), (311), (400), (422), (511), and (440). These peaks are in accordance with the crystalline cubic system of iron oxide NPs (Bilici et al., Reference Bilici, Badak, Zengin, Suludere and Aktas2020; Maleki et al., Reference Maleki, Hajizadeh and Firouzi-Haji2018; Xie et al., Reference Xie, Qian, Wu and Ma2011). The magnetic behavior of MHNTs is examined using VSM. The saturation magnetization value could be observed through the magnetization of MHNTs as a function of the applied magnetic field (sweeping from –1 to 1 T) curve at room temperature (25°C). The magnetization increases with increase in the magnetic field. MHNTs possess superparamagnetic properties with saturation magnetization ranging from 21 to 50 emu g–1 (Hajizadeh et al., Reference Hajizadeh, Maleki, Rahimi and Eivazzadeh-Keihan2020b; He et al., Reference He, Zou, Chen, Dai, Xie, Zhou and Yan2016a, Reference He, Chen, Zhang, Hu, Wang and Wang2016b; Shi et al., Reference Shi, Pang, Chen, Wu and Zhang2020). Saturation magnetization depends on the synthesis conditions and on the size of the MNPs (He et al., Reference He, Yi, Zhang, Zhao and Yu2021). The chemical composition of MHNTs is investigated using EDS where very strong signals of Fe, O, Al, and Si are observed in the MHNTs (Dramou et al., Reference Dramou, Fizir, Taleb, Itatahine, Dahiru, Mehdi, Wei, Zhang and He2018; Hajizadeh, et al., Reference Hajizadeh, Hassanzadeh-Afruzi, Jelodar, Ahghari and Maleki2020a; Shi et al., Reference Shi, Pang, Chen, Wu and Zhang2020). The TEM and SEM analyses are used to examine the morphological structure and diameter of MHNTs. The incorporation of MNPs does not affect or modify the morphology of the HNTs (Zhu et al., Reference Zhu, Fan, Wang, Zhai, Hu, Li and Jiang2021). Typically, the diameter of MNPs and their surface morphology depend on the design approach and experimental conditions. In the present review, the morphology of the prepared MHNTs by different synthesis methods are discussed. For example, in the MHNTs prepared by co-precipitation methods, the MNPs were deposited and attached to the external surface of HNTs with diameters ranging from 5 to 50 nm. As shown in Fig. 5a, the size of iron oxide particles is ~16 nm (Fizir et al., Reference Fizir, Dramou, Zhang, Sun, Pham-Huy and He2017; Li et al., Reference Li, Wang, Lv, Liu, Zhang and Shao2018b). The same morphology was observed for MHNTs prepared by solvothermal methods (Fig. 5b) (Pan et al., Reference Pan, Yao, Xu, Ou, Huo, Li and Yan2011) and direct mixing methods (Fig. 5c) (Mu et al., Reference Mu, Zhang and Wang2014b). However, the size of MNPs formed by thermal decomposition (range 75 to 200 nm) is larger than that from co-precipitation (Fig. 5d). For MHNTs prepared by the wet impregnation technique, it was noted that MNPs were distributed on the inside surfaces of HNTs with diameters ranging from 5 to 10 nm (Fig. 5e) (Pan et al., Reference Pan, Hang, Dai, Dai, Huo and Yan2012a). As for the morphology of MHNTs synthesized by the encapsulation method, functionalized MNPs of diameter ∼6 nm were loaded in their entirety into the lumens of HNTs (Fig. 5f) (Hamza et al., Reference Hamza, Ferretti, Innocenti, Fidecka, Licandro, Sangregorio and Maggioni2020).
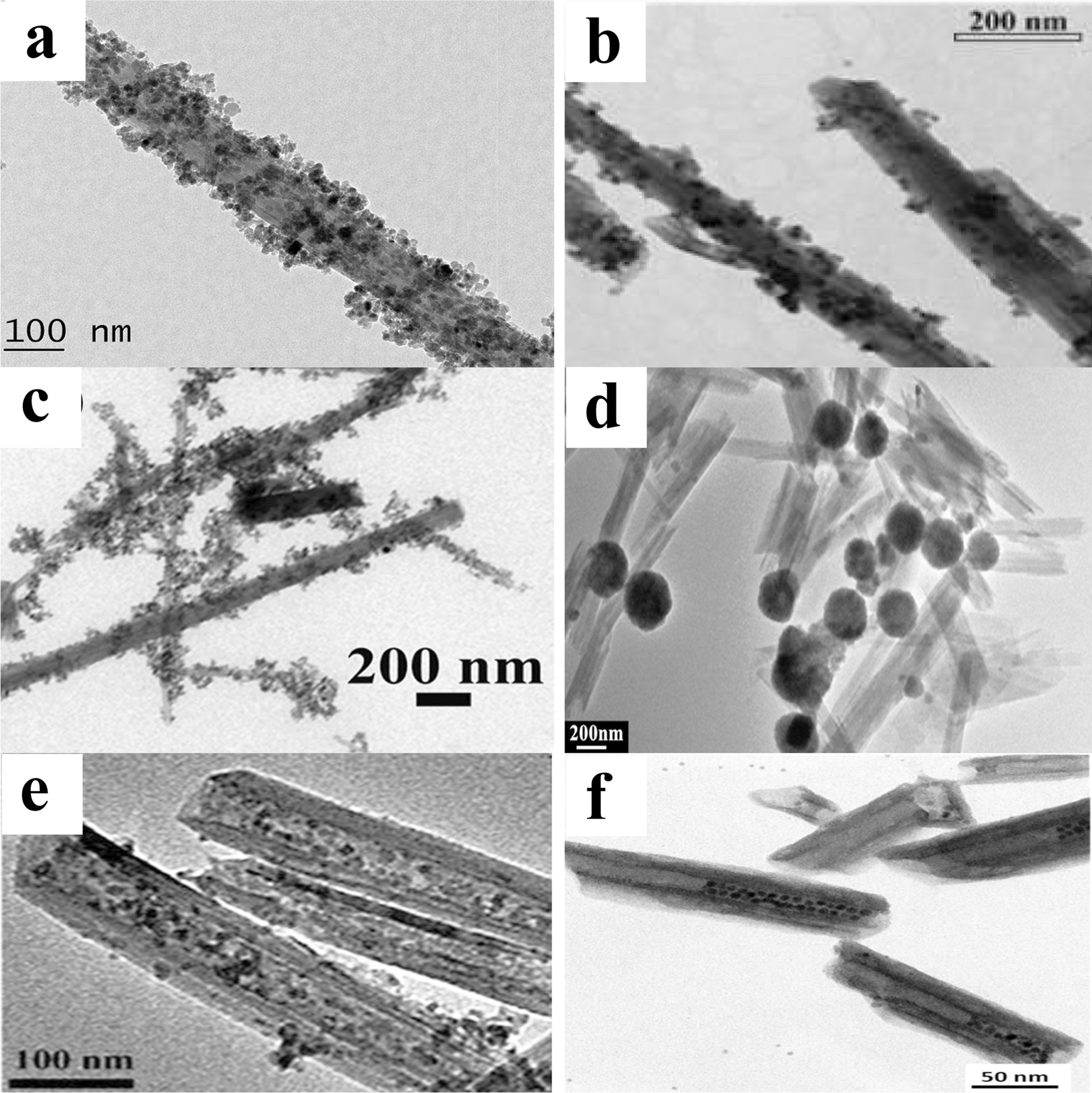
Fig. 5 TEM images of MHNTs prepared by: a co-precipitation (reproduced from Fizir et al. (Reference Fizir, Dramou, Zhang, Sun, Pham-Huy and He2017); Li et al. (Reference Li, Zha, Niu, Hu, Hui, Tang, Fizir and He2018a) with the permission of Elsevier), b, d solvothermal (reproduced from Pan et al. (Reference Pan, Yao, Xu, Ou, Huo, Li and Yan2011) with permission of the American Chemical Society), c direct mixing (reproduced from Mu et al., Reference Mu, Zhang and Wang2014b with permission of Springer), e wet impregnation (reproduced from Pan et al., (Reference Pan, Hang, Dai, Dai, Huo and Yan2012a) with the permission of Elsevier), and f encapsulation methods (reproduced from Hamza et al. (Reference Hamza, Ferretti, Innocenti, Fidecka, Licandro, Sangregorio and Maggioni2020) with the permission of the American Chemical Society)
Surface Functional Modification of MHNTs
When MHNTs are used as adsorbents for pollutants or other analytes, MHNTs show only a weak affinity (ion exchange, hydrogen bonding, and van der Waals forces) for the guest. To enhance the performance of MHNTs in the above-mentioned area, surface functionalization of MHNTs (Fig. 6), normally site-specific and sometimes selective, is extremely advantageous.
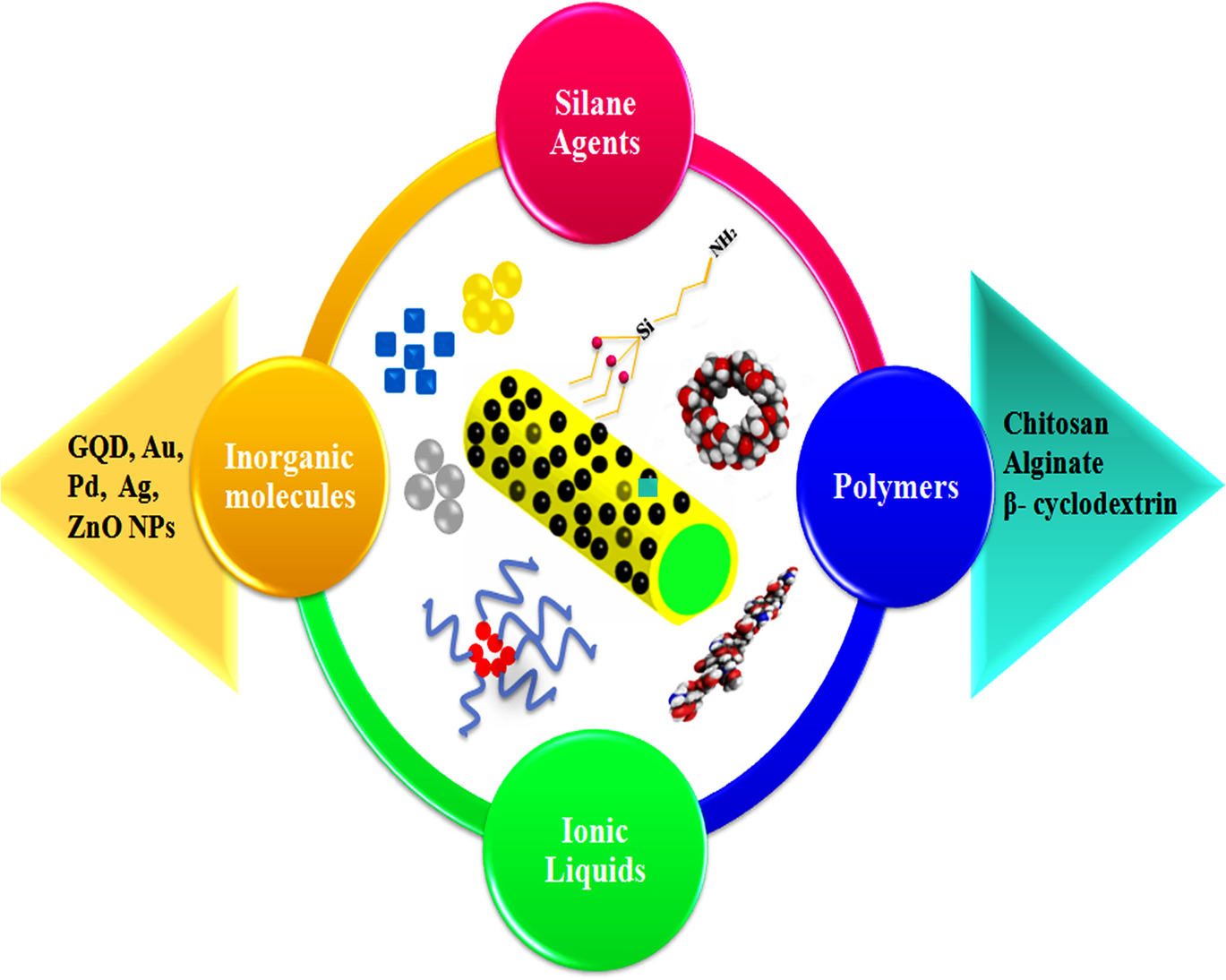
Fig. 6 Surface functional modification of MHNTs with various organic and inorganic molecules
Functionalization of MHNTs-Based Silane Coupling Agent
Modification of inorganic nanomaterials using a silane agent is a chemical surface modification approach that has attracted much interest to control dispersion and aggregation of nanoparticles (Ahangaran & Navarchian, Reference Ahangaran and Navarchian2020). Silane modifiers are one of the most significant bifunctional modifiers that are used commonly for the surface functionalization of HNTs or MHNTs (Fig. 7). The most important characteristic of silane modifiers (Table 4) is their capability to create a stable chemical bond between organic and inorganic MHNTs. Typically, silane coupling agents are grafted on MHNTs via covalent modification. The reaction mechanism of the grafting process is the condensation between the hydrolyzed silanes and the hydroxyl groups located on the external surfaces of the MHNTs (Liu et al., Reference Liu, Jia, Jia and Zhou2014). External surface modification can improve the adsorption efficiency of MHNTs (Zhu et al., Reference Zhu, Duan, Wang, Gao, Jia, Ma and Wang2017) and is considered as a vital step to prepare polymer composites because silane coupling improves the bonding performance of organic polymers (Foroughirad et al., Reference Foroughirad, Haddadi-Asl, Khosravi and Salami-Kalajahi2020a; Hajizadeh & Maleki, Reference Hajizadeh and Maleki2018; Zhu et al., Reference Zhu, Fan, Wang, Zhai, Hu, Li and Jiang2020). Generally, dispersions of the MHNTs and silane agents may undergo condensation reactions in toluene (Kadam et al., Reference Kadam, Jang and Lee2017), acetic acid/alcohol (Sillu & Agnihotri, Reference Sillu and Agnihotri2019), water/alcohol (Hang et al., Reference Hang, Li, Pan, Li, Dai, Dai, Yu and Feng2013),or ethyl alcohol (Zhu et al., Reference Zhu, Duan, Wang, Gao, Jia, Ma and Wang2017). All reactions were kept under reflux conditions (60°C) for 24 h or more. MHNTs may also be modified by 3-amino-npropyltriethoxysilane (APTES), through which the ending amine group is anchored onto the MHNT’s surface to initiate the polymerization process (Foroughirad et al., Reference Foroughirad, Haddadi-Asl, Khosravi and Salami-Kalajahi2020a). Reportedly, the uniform silanol (Si − OH) sites over MHNTs can be transformed into much stronger siloxane (Si − O − Si) bonds through amino silanization by APTES, causing orientation of amine groups away from the surface (Sillu & Agnihotri, Reference Sillu and Agnihotri2019).
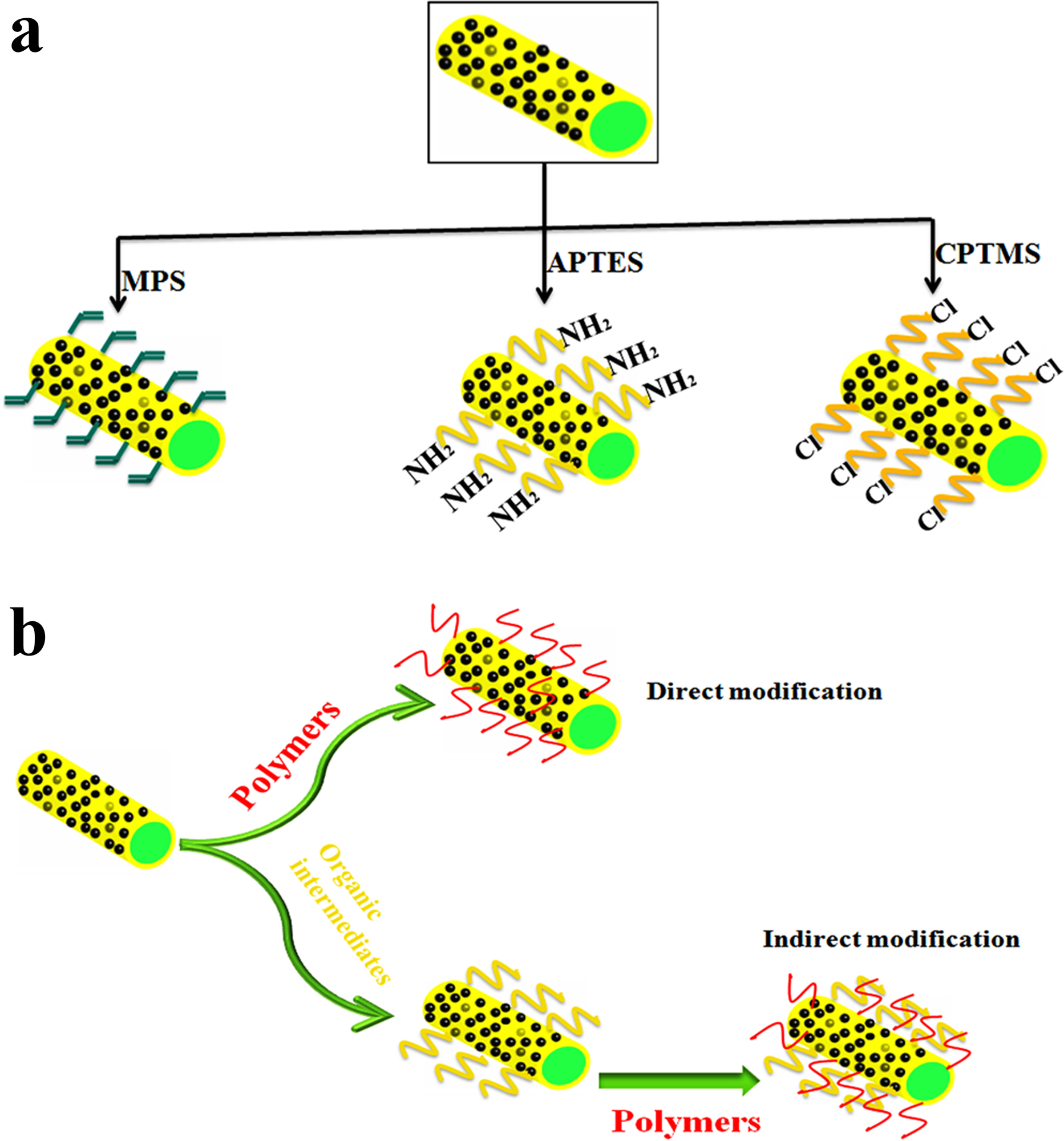
Fig. 7 a Functionalization of MHNT-based silane coupling agents and b polymer modification approaches
Table 4 Silane coupling agents used for modification of MHNTs
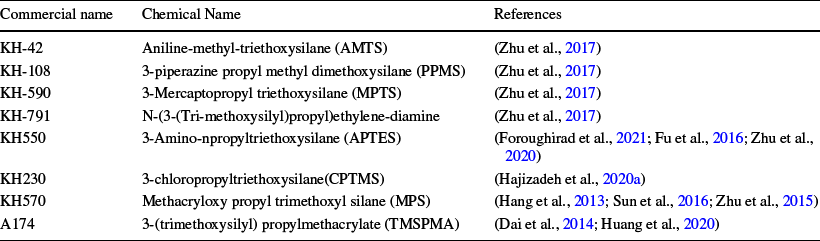
Recently, surface vinyl functionalization of the MHNTs by methacryloxy propyl trimethoxyl silane was developed to introduce polymerizable double bonds (Fizir et al., Reference Fizir, Wei, Muchuan, Itatahine, mehdi, He and Dramou2018b). Thermogravimetric analysis (TGA) showed that the grafting ratio was 9.60% (relative to MHNTs) (Hang et al., Reference Hang, Li, Pan, Li, Dai, Dai, Yu and Feng2013; Sun et al., Reference Sun, Chen, Li, Li, Zhu, Hu, Huang, Li and Zhong2016). Silane coupling agents including anilino-methyl-triethoxysilane and 3-piperazinepro pylmethyldi-methoxysilane were grafted on MHNTs to increase the adsorption capacity of magnetic nanotubes. The grafting reaction was performed in ethyl ethanol solution. Analysis by TEM showed a thin layer of membrane coated on the surface of MHNTs. The specific surface area of the modified MHNTs decreased because the micro-pores of the HNTs were partially plugged by the silane (Zhu et al., Reference Zhu, Duan, Wang, Gao, Jia, Ma and Wang2017).
Silane grating methods can be employed further as a pretreatment before additional surface modifications. For example, to prepare MHNTs-Ag, MHNTs was modified with APTES where the amino groups of APTES were complexed with silver ions and formed large clusters on the surface of MHNTs (Fu et al., Reference Fu, Liao, Li and Chen2016). In another study, to synthesize MHNTs-COOH, MHNTs were first functionalized with amino groups by grating APTES. Subsequently, the MHNTs-NH2 product was mixed and stirred with succinic anhydride in dimethyl formamide, generating the MHNTs-COOH (Pan et al., Reference Pan, Hang, Dai, Dai, Huo and Yan2012a).
Functionalization-based Polymers
The preparation of MHNTs with high sorption efficiency has recently become a new research hotspot. The MNPs introduced on magnetic nanotube surfaces cause adverse defects such as lower uptake capacity, which restricts their large-scale application, however. Fortunately, the aforementioned drawback has been solved successfully via the selective modification of MHNTs with natural and synthetic polymers (Yang et al., Reference Yang, Zong, Hu, Sheng, Wang and Wang2013a). As expected, the nano-composites obtained exhibited outstanding utility in the removal of heavy metal ions, dyes, and organic contaminants. The functionalization of MHNTs with the polymer layer increases not only the binding capacity but also the mechanical and thermal stability, thereby resulting in an adsorbent with superior properties compared to those of either the magnetic nanotubes themselves or the polymer (Khunová et al., Reference Khunová, Šafařík, Škrátek, Kelnar and Tomanová2016; Liu et al., Reference Liu, Jia, Jia and Zhou2014; Vahidhabanu et al., Reference Vahidhabanu, Adeogun and Babu2019). The external and internal surfaces of MHNTs are both polar due to the silica and alumina species which provide sufficient hydrophilicity, and, consequently, good dispersion in polymers such as polyethyleneimine (PEI) (Hajizadeh & Maleki, Reference Hajizadeh and Maleki2018; Zhou et al., Reference Zhou, Jia, Luo, Xu, Chen, Ge, Ma, Chen and Zhu2016), polyacrylates (Foroughirad et al., Reference Foroughirad, Haddadi-Asl, Khosravi and Salami-Kalajahi2020b), and biopolymers such as chitosan (CS) (Dramou et al., Reference Dramou, Fizir, Taleb, Itatahine, Dahiru, Mehdi, Wei, Zhang and He2018; Kadam et al., Reference Kadam, Jang, Jee, Sung and Lee2018; Kim et al., Reference Kim, Jee, Sung and Kadam2018; Lee et al., Reference Lee, Lee, Jee, Sung and Kadam2019; Vahidhabanu et al., Reference Vahidhabanu, Adeogun and Babu2019), alginate (Polat & Açıkel, Reference Polat and Açιkel2019), and cyclodextrin (β-CD) (Li et al., Reference Li, Chen, Liu, Deng, Li, Ren, Huang, Chen, Yang and Zhong2019; Yang et al., Reference Yang, Zong, Hu, Sheng, Wang and Wang2013a).
Many publications exist on the functionalization of MHNTs with chitosan (Dramou et al., Reference Dramou, Fizir, Taleb, Itatahine, Dahiru, Mehdi, Wei, Zhang and He2018; Kadam et al., Reference Kadam, Jang, Jee, Sung and Lee2018; Kim et al., Reference Kim, Jee, Sung and Kadam2018; Lee et al., Reference Lee, Lee, Jee, Sung and Kadam2019; Vahidhabanu et al., Reference Vahidhabanu, Adeogun and Babu2019). The hybridization is achieved by simple solution mixing of MHNTs with CS solution in a suitable solvent with vigorous stirring or ultrasonic treatment in the presence of glutaraldehyde as a cross-linker (Kadamet al., Reference Kadam, Sharma, Shinde, Ghodake, Saratale, Saratale, Kim and Sung2020a; Türkeş & Açıkel, Reference Türkeş and Açιkel2020). MHNTs can interact with cationic polymers, such as CS and PEI, via electrostatic attraction (Kadam et al., Reference Kadam, Shinde, Ghodake, Saratale, Saratale, Sharma, Hyun and Sung2020b). MHNTs were modified with chitosan oligosaccharides (COS) via a simple assembling method with a 5.9% grafting ratio. The cationic amine group of COS interacts with the negatively charged outer surface of MHNTs through electrostatic attraction. Moreover, the Si–O groups of MHNTs can interact with the amine and hydroxyl groups of chitosan oligosaccharides via hydrogen bonding (Dramou et al., Reference Dramou, Fizir, Taleb, Itatahine, Dahiru, Mehdi, Wei, Zhang and He2018).
Water-soluble polymers such as PEI can also be mixed directly with magnetic halloysite. In a study by Zhu et al. (Reference Zhu, Fan, Wang, Zhai, Hu, Li and Jiang2020), branched PEI with sufficient amino groups was used to cross-link to MHNTs-APTES using glutaraldehyde. PEI has shown effective binding with MHNTs; its intrinsic toxicity problem needs to addressed, however. MHNT-alginate nanocomposite gel beads can be prepared by mixing the MHNTs in water and crosslinking them using calcium ions (Polat & Açıkel, Reference Polat and Açιkel2019).
In measurement with MHNT-polymer composites, MHNTs can be modified by cyclodextrin via covalent functionalization where amide bonds are formed between carboxylated β-CD and the primary amines on MHNTs (Li et al., Reference Li, Chen, Liu, Deng, Li, Ren, Huang, Chen, Yang and Zhong2019).
Apart from being used to prepare MHNT-polymer nanocomposites, these interactions can also be employed for the surface treatment of MHNTs for further modification such as enzyme immobilization for catalytic purposes (Kadamet al., Reference Kadam, Shinde, Ghodake, Saratale, Saratale, Sharma, Hyun and Sung2020b; Zhu et al., Reference Zhu, Fan, Wang, Zhai, Hu, Li and Jiang2020). Note that enzyme immobilization can also be carried out without polymer modification as reported by Sillu and Agnihotri. (Reference Sillu and Agnihotri2019), in which cellulase was immobilized directly on the amine-modified MHNTs using aminosilane surface functional chemistry.
MHNTs have also been used as support to initiate surface polymerization of various kinds of monomers such as methacrylic acid, acrylamide, acrylic acid, etc. For example, novel MHNTs grafted polymer using vinyl monomers has been prepared for a sustained-release drug-delivery system (Fizir et al., Reference Fizir, Dramou, Zhang, Sun, Pham-Huy and He2017). Similarly, [2-(acryloyloxy) ethyl]trimethylammonium chloride (AETAC) along with APTES has been used as monomers and grafted on the surface of MHNTs via co-precipitation polymerization (Foroughirad et al., Reference Foroughirad, Haddadi-Asl, Khosravi and Salami-Kalajahi2020b).
From the studies above, polymer functionalization of MHNTs can be achieved by direct or indirect approaches. Direct polymer functionalization is carried out directly on the surface of MHNTs whereas, indirect functionalization is achieved by surface modification of MHNTs with organic intermediates (bridge) such as APTES or glutaraldehyde to adjust the compatibility between the inorganic cores (MHNTs) and the polymer shell. Despite the direct method being simple, the indirect method is the more convenient pathway and leads to a higher graft ratio of polymer (Gao, Reference Gao2019). Polymer modification approaches are simplified and depicted in Fig. 7b.
Functionalization-based Inorganic Materials
Anchoring small inorganic molecules such as graphene quantum dots (GQD), gold (Au) (Jia et al., Reference Jia, Zhou, Xu, Li, Dong, Huang and Xu2016), palladium (Pd) (Jia et al., Reference Jia, Zhou, Xu, Li, Xu, Zhang, Guo, Shen and Zhang2017), silver (Ag) (Gan et al., Reference Gan, Huang, Zhang, Pan, Shi and Yan2015; Rouhi et al., Reference Rouhi, Babamoradi, Hajizadeh, Maleki and Maleki2020), and zinc oxide (ZnO) NPs (Jee et al., Reference Jee, Kim, Shinde, Ghodake, Sung and Kadam2020), etc., on the surface of MHNTs is the easiest functionalization approach. There are two strategies of modification. The first consists of adding one, two, or more small inorganic molecular materials into a solution containing magnetic HNTs. The small inorganic molecules interact with each other and condense on the surface of MHNTs. As proof of concept, MHNTs have been modified successfully with inorganic antimicrobial zinc oxide (ZnO) NPs in two steps: first, the Zn2+ ions were attached tightly to the negative surface of the MHNTs; and second, the calcination treatment led to the nucleation of Zn2+ to form the ZnO on the surface MHNTs (Jee et al., Reference Jee, Kim, Shinde, Ghodake, Sung and Kadam2020).
Ag nanoparticles with a mean diameter of 20 nm were embedded on the external surfaces of MHNTs via the chemical-reduction method where AgNO3 was used as a precursor and mixed with a MHNTs dispersion. The TEM results showed that Ag NPs were spherical in shape (Gan et al., Reference Gan, Huang, Zhang, Pan, Shi and Yan2015). MHNT-AuPd was synthesized by mixing MHNT solution with the NPs precursor’s solution (HAuCl4 and K2PdCl4) at ambient temperature. The TEM results showed that AuPd NPs with diameters of <5 nm were formed and deposited on the surface of MHNTs (Jia et al., Reference Jia, Zhou, Xu, Li, Xu, Zhang, Guo, Shen and Zhang2017).
The second strategy consists of immobilization or self-assembly of the inorganic metal compound directly on the surface of the magnetic HNTs. This strategy was exploited to prepare MHNT-Au and MHNT-Au-Ni where Au or Au-Ni NPs arranged on the external wall of MHNTs by simply blending the Au or Au-Ni NPs with thiol-group-modified MHNTs via metal-S bonds (Jia et al., Reference Jia, Zhou, Xu, Li, Dong, Huang and Xu2016). The outer surfaces of APTES-modified MHNTs was decorated with AuNPs via electrostatic interaction (Fig. 8) (Zhang et al., Reference Zhang, Lai, Wu, Li and Hu2020).
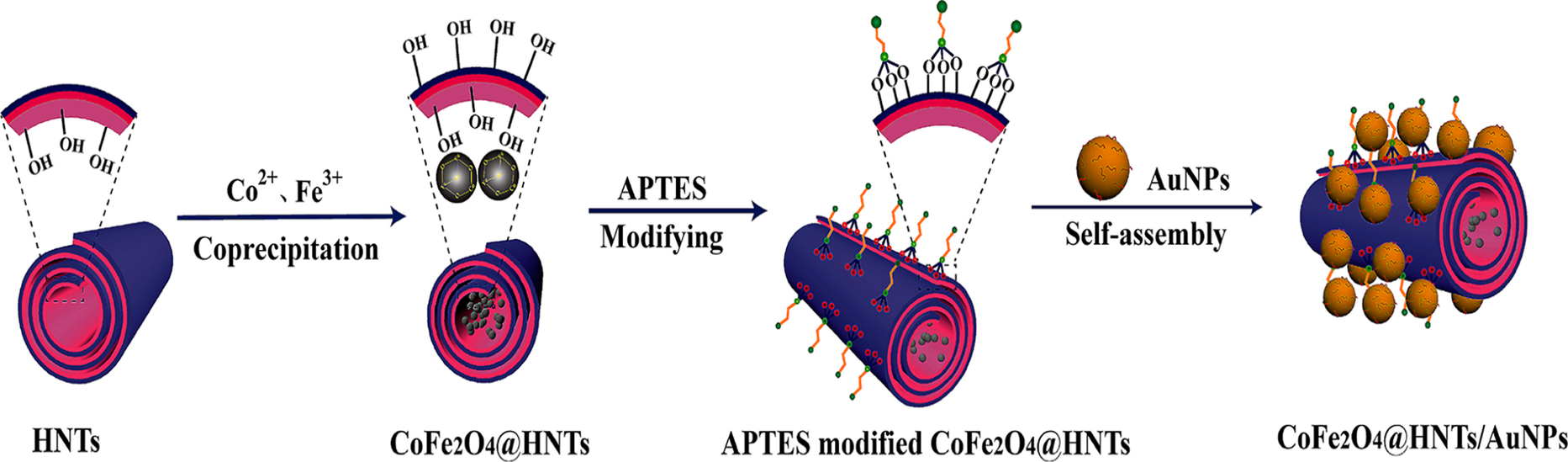
Fig. 8 Synthesis process of MHNT/AuNP composites (reproduced from Zhang et al. (Reference Zhang, Lai, Wu, Li and Hu2020) with the permission of the American Chemical Society)
Similarly, a graphene quantum dot with excellent electrical properties was anchored on the external surface of MHNTs through covalent immobilization. The GQD/MHNTs can be synthesized by thermal decomposition of citric acid to produce GQD and subsequently form amide bonds between carboxylated GQD and amine-modified MHNTs via the EDC/NHS reaction (Zare Pirhaji et al., Reference Zare Pirhaji, Moeinpour, Mirhoseini Dehabadi and Yasini Ardakani2020a; Zare Pirhaji et al., Reference Zare Pirhaji, Moeinpour, Mirhoseini Dehabadi and Yasini Ardakani2020a, Reference Zare Pirhaji, Moeinpour, Mirhoseini Dehabadi and Yasini Ardakani2020b). The same strategy was used to prepare functionalized MHNTs with N-doped graphene quantum dots (Ganganboina et al., Reference Ganganboina, Chowdhury and Doong2017). The uniform dispersion of GQD with a mean diameter of 7 nm on the MHNT surfaces was confirmed by TEM analysis. The nanocomposites (NiFe2O4/HNTs/GQDs) prepared showed significant superparamagnetic features (60.82 emu g–1).
Functionalization-based Ionic Liquids
Ionic liquids (ILs), defined as low melting-point organic salts, are a novel class of compounds with unique properties and a combinatorially broad chemical diversity (Ghandi, Reference Ghandi2014). The surface functionalization of nanoparticles by ionic liquids attracts significant research attention. Ionic liquids such as cetyltrimethyl ammonium bromide (CTAB), sodium dodecyl sulfate (SDS), or 1-hexadecyl-3-methylimidazolium bromide (C16mimBr) were adsorbed on the surfaces of various nanomaterials such as CNTs (Bai et al., Reference Bai, Park, Lee, Bae, Watari, Uo and Lee2011), GO (Das et al., Reference Das, Maiti, Ghosh, Mandal and Das2013), and HNTs (Abhinayaa et al., Reference Abhinayaa, Jeevitha, Mangalaraj, Ponpandian and Meena2019) to enhance their stability and loading capacity. More recently, C16mimBr has been coated, in non-covalent fashion, on the surfaces of MHNTs by means of a simple sonication treatment. The formation mechanism consists mainly of electrostatic interaction between the C16mim+ and negatively charged MHNTs. The formation of C16mimBr with mixed hemimicelles on the surfaces of MHNTs led to the retention of analytes by strong hydrophobic, π–π, and electrostatic interactions which led to an increase in the uptake capacity of the MHNTs (Liu, et al., Reference Liu, Fizir, Hu, Li, Hui, Zha and He2018a). Based on the encouraging results obtained from the nanomaterials above, novel mixed hemimicelles on the surfaces of MHNTs have been developed using two types of ionic surfactants (non-ionic surfactant (TX100) and 1-cetyl-3-methylimidazolium bromide (C16mimBr)) to endow the mixed hemimicelles with more hydrophobic properties. The ratio of TX100/C16mimBr 0.64 showed significant recovery of the extracted analytes whereas increasing the amount of TX100 resulted in a poor extraction rate due to the saturation of the MHNT-C16mimBr (Liu et al., Reference Liu, Wang, Hu, Wu, Huang, Fizir and He2018b). The analytical achievement of the above-functionalized MHNTs will be discussed in the next section.
Analytical and Environmental Applications of MHNTs and MHNT Nanocomposites as Magnetic Adsorbents
The removal of emerging contaminants, e.g. dyes, heavy metal ions, radioactive ions, pesticides, etc., by adsorption, offers numerous advantages such as low cost, biocompatibility, and removal efficiency over other cleanup methods. The key player in applying MHNT nanocomposites as adsorbents is a special tubular structure that does not exist in other nanomaterials and is ideally suited to the task of contaminant destruction and elimination. MHNT nanocomposites are not limited to separation of contaminants from environmental wastewaters; they can also be used in monitoring and detection of various therapeutic agents, proteins, and mycotoxins in biological and food samples. The analytical application of MHNTs has gained significant attention (Fig. 9) where the removal of dyes and heavy metal ions is the main requirement.
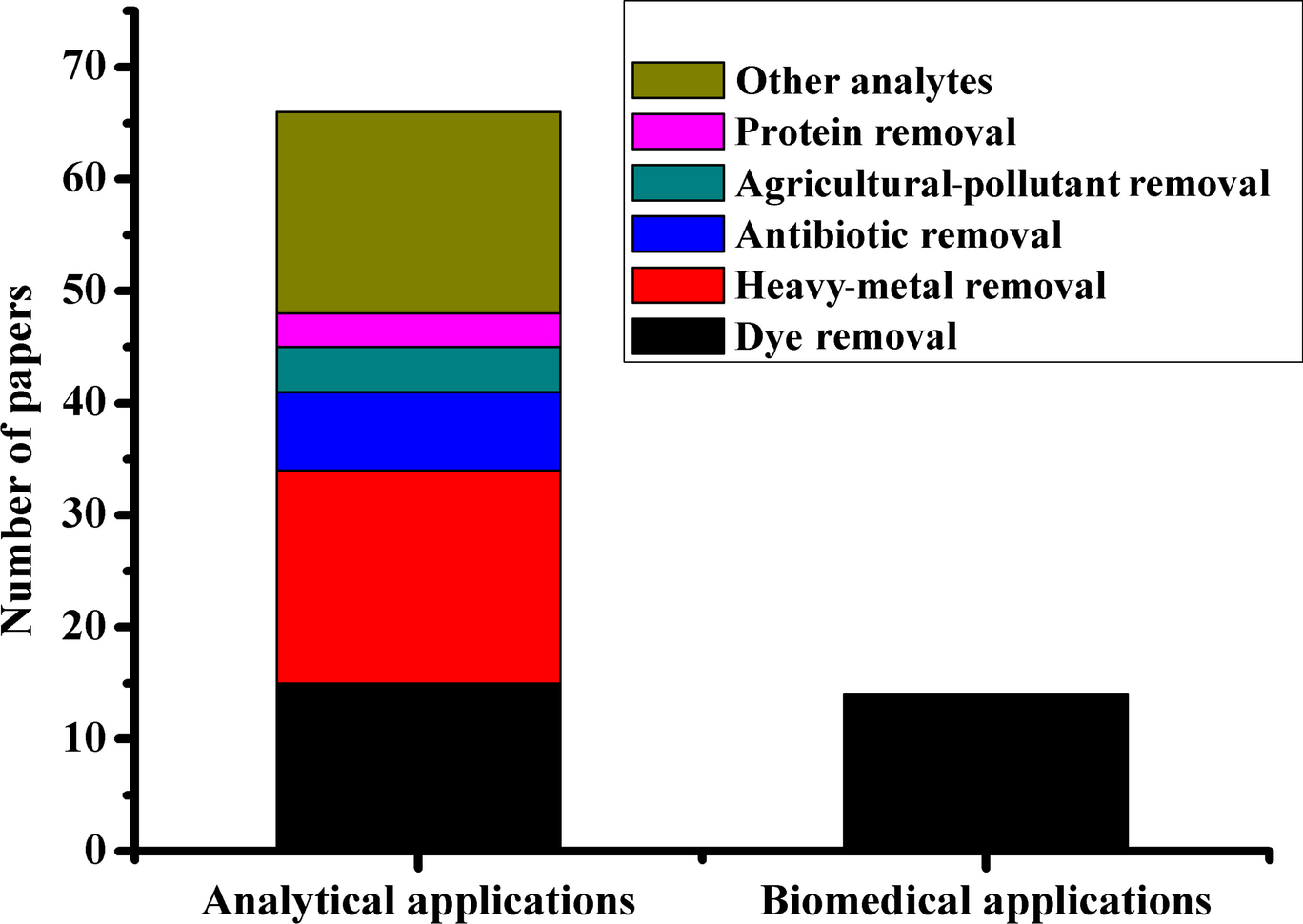
Fig. 9 Number of papers published relating to fields of application of MHNTs and various adsorbed analytes
MHNTs and MHNT Nanocomposites for Dye Removal
Wastewaters containing dyes are hazardous and carcinogenic to human beings (Zhou et al., Reference Zhou, Lu, Zhou and Liu2019). Various researchers have attempted to develop substitute nanomaterials for dye adsorption. In this section, the dye adsorption and removal capability from aqueous and environmental samples of MHNTs and composites-based MHNTs is compiled. The adsorption of dyes by MHNTs was investigated first by Xie et al. (Reference Xie, Qian, Wu and Ma2011). MHNTs exhibited better adsorption of cationic methylene blue (MB) than neutral red (NR) and methyl orange (MO). However, the adsorption capacity of HNTs was twice as great as that of MHNTs. The comparable results between the three dyes studied are due to the negatively charged surfaces of MHNTs which could adsorb more cationic dyes such as MB (Xie et al., Reference Xie, Qian, Wu and Ma2011). In another work, MHNTs showed a maximum adsorption capacity of 11.10 mg g–1 for 100 mg L–1 naphthalene green (Riahi-Madvaar et al., Reference Riahi-Madvaar, Taher and Fazelirad2017). An increase in the MB adsorption capacity of 57.13 mg g–1 was observed using HNT/Fe3O4/C as an adsorbent (Jiang et al., Reference Jiang, Zhang, Wei, Tjiu, Pan, Chen and Liu2014a).
In measurements involving dye removal, the mechanical strength of supramolecular gels was improved by introducing MHNTs in their structures and it is used for dye adsorption. However, the resulting adsorption capacity was very small (Zeng et al., Reference Zeng, Sun, Wang, Wang and Yang2016) and was similar to unmodified MHNTs (Xie et al., Reference Xie, Qian, Wu and Ma2011). Consequently, this multistep-prepared supramolecular gel is not recommended for dye adsorption. MHNTs were functionalized by various kinds of polymers (i.e. polydopamine and chitosan) and ionic liquids to enhance their dye-adsorption capacity. An excellent MB-removal capacity, up to 714.29 mg g−1, was obtained using the core-double-shell structured MHNTs/poly(dopamine + APTES) nano-hybrids as adsorbents. The removal efficiency reached 89% for MB after five adsorption–desorption cycles, which means that the nanohybrids possess an outstanding regeneration capacity. The excellent removal efficiency is attributed to the fact that MHNTs/poly(dopamine + APTES) possessed a large number of negatively charged amine groups and catechol groups under an alkaline medium, which could react with MB through the electrostatic interaction and π–π stacking interaction (Wan et al., Reference Wan, Zhan, Long, Zeng and He2017). The aforementioned material was used in the polyvinylidene fluoride (PVDF) membrane to enhance its hydrophilicity and water flux. These composites showed a good MB removal rate of ~97.2% (Zhang et al., Reference Zhang, Zhu, Liu, Cai, Han, Zhang and Li2019).
Similarly, 2-(acryloyloxy)ethyl-trimethylammonium chloride (AETAC) and APTES as cationic and co-monomers, respectively, were polymerized on the surface of MHNTs and used as adsorbents of sunset yellow. The composites showed a large and quick adsorption capacity of 33.8 µmol g–1 in only15 min. The dominant adsorption mechanism was the electrostatic interactions between the anionic sulfonic acid groups of the dye and the cationic functional groups of the monomer AETAC (Foroughirad et al., Reference Foroughirad, Haddadi-Asl, Khosravi and Salami-Kalajahi2020b).
More recently, chitosan-modified MHNTs as adsorbents for dye removal have attracted significant research attention. For example, sponge-like MHNT/CS composite synthesized by combining solution-mixing and freeze-drying showed a maximum Congo red dye adsorption capacity of 54.49 mg g−1, which was greater than that of HNTs/CS (41.54 mg g–1) due to the additional surface charge provided by MNPs. Electrostatic interactions play an important role in the binding between dye and MHNTs/CS (Vahidhabanu et al., Reference Vahidhabanu, Adeogun and Babu2019). The aforementioned adsorbent also showed a good MB adsorption ability of 50.37 mg g–1 (Türkeş & Açıkel, Reference Türkeş and Açιkel2020).
The uptake capacity of MO has been improved by using mixed hemimicelles-based MHNTs as low-cost adsorbents. The outcomes indicate that MHNTs-C16mimBr could adsorb ~150 mg of MO and 90 mg of methyl red (MR) per gram of MHNTs. This good adsorption efficiency may be due to the following: (1) dyes could interact with adsorbents through electrostatic, π–π, and hydrophobic interactions provided by the micelles adsorbed on the surfaces of MHNTs; and (2) the lumen of HNTs could retain the dyes through the electrostatic interaction between OH2+ of the aluminum group (Al–OH) and negative charge of MO and MR. This adsorbent showed significant rates of recovery, 85–87% for MR and 89–93% for MO from tap and lake waters, and low detection limits, 0.042 µg L−1 for MR and 0.050 µg L−1 for MO (Liu et al., Reference Liu, Fizir, Hu, Li, Hui, Zha and He2018a).
The reviewed literature shows that polydopamine-grafted MHNTs and MHNTs-C16mimBr are the best substitute nanomaterials for MB and MO removal from environmental wastewaters, respectively. However, further research is needed for the application of polydopamine-grafted MHNTs in real samples. In addition, the selectivity of the aforementioned materials should be enhanced by grafting new elements on the surfaces of MHNT-based composites. The application of MHNT nanocomposites to different kinds of dyes is summarized in Table 5.
Table 5 Summary of the application of MHNT nanocomposites to various kinds of dyes
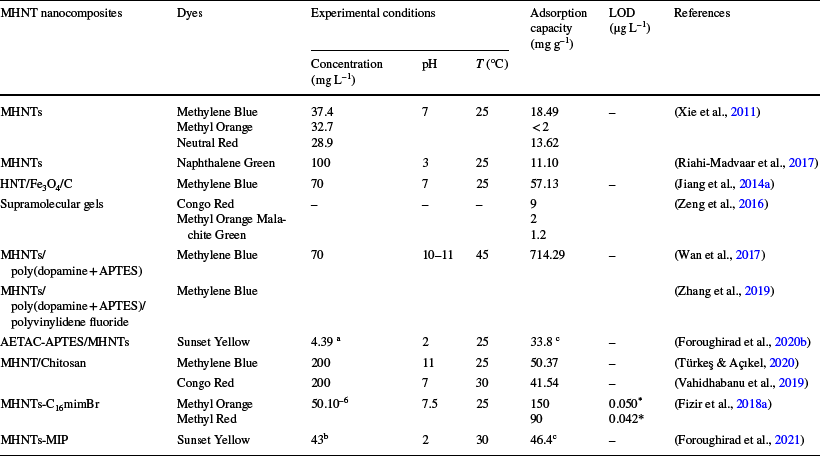
aµM/mL, bμmol/L, and cμmol/g
* Detection technique: HPLC–UV
MHNTs and MHNTs Nanocomposites for Heavy Metal Removal
Heavy metal ions pose a tremendous threat to ecosystems and living creatures due to their high toxicity (Siddeeg et al., Reference Siddeeg, Tahoon, Alsaiari, Shabbir and Rebah2021). MHNT nanocomposite materials have a special macro and mesoporous structure and excellent adsorption performance which can be applied to adsorption and removal of heavy metal ions. In this section, the role and potential of MHNTs in heavy-metal ion elimination from real samples will be reviewed.
Monovalent Heavy Metal Ions
Magnetic HNTs were employed as adsorbents to study the adsorption characteristics of some monovalent metal ions such as Ag(I) and Tl(I). The quantification of these metal ions using MHNTs will be summarized in this section.
Thallium (Tl) is among the most significant toxic metal ions in drinking water. However, it has been studied only rarely (Shah et al., Reference Shah, Nisar, Khan, Nisar, Niaz, Ashiq and Akhter2019). A new nanocomposite consisting of magnetite halloysite nanotubes and dibenzo-18-crown-6 was developed for the preconcentration of Tl(I) via ultrasound-assisted solid-phase extraction combined with electrothermal atomic absorption spectrometry. Dibenzo-18-crown-6 was used due to its selectivity for Tl(I) ions. The methods demonstrated significant recovery of Tl(I) from real samples such as tap water and human hair, up to 104% and the detection limit was 1.8 ng L–1 (Ashrafzadeh Afshar et al., Reference Ashrafzadeh Afshar, Taher and Fazelirad2017).
Magnetic halloysite nanotubes modified with 5-(p-dimethylaminobenzylidene) rhodanine, a silver-specific dye, has been investigated as a selective adsorbent for the removal of Ag(I) from environmental samples. The adsorbent possessed a fast adsorption capacity of 16.2 mg g–1 in 45 min. In the concentration range from 4.0 to 200 µg L−1of Ag+, the limit of detection was 1.6 µg L–1. The recovery of silver ions from the soil sample was up to 102.0% and no interferences were detected (Amjadi et al., Reference Amjadi, Samadi and Manzoori2015a).
Divalent Heavy Metal Ions
Several types of divalent metal ions such as Cu(II), Pb(II), Cd(II), and Hg(II) have been adsorbed by MHNTs. The adsorption capacity of MHNTs for cadmium (Cd2+) in aqueous solution was 11.4 mg g−1 and the recovery in spiked waters, nail, and hair samples by MHNTs coupled with a flame atomic absorption spectrometry method ranged from 96.7 to 104.2% with a detection limit of 0.27 µg L–1 (Amjadi et al., Reference Amjadi, Samadi and Manzoori2015a). These results are comparable to other nanomaterials studied such as functionalized magnetic CNTs (Taghizadeh et al., Reference Taghizadeh, Asgharinezhad, Samkhaniany, Tadjarodi, Abbaszadeh and Pooladi2014) and MOFs (Sohrabi et al., Reference Sohrabi, Matbouie, Asgharinezhad and Dehghani2013) where they showed excellent adsorption performances (>185 mg g–1) and low detection limits (<0.20 µg L–1). However, low-cost MHNTs without functionalization still showed acceptable results for Cd2+ removal and analysis whereas the other nanomaterials need labor-intensive preparation steps. In an attempt to enhance the removal capacity of MHNTs, GQD was immobilized onto the external surface of MHNTs to remove Cd2+ and Pb2+ from water. The adsorbent showed an improved uptake capacity of 34.72 and 42.02 mg g–1 in <60 min. The removal of metal ions is based on the construction of complexes (bidentate and monodentate) between the carboxyl and hydroxyl species on the surfaces of MHNTs/GQDs and the metal ions (Zare Pirhaji et al., Reference Zare Pirhaji, Moeinpour, Mirhoseini Dehabadi and Yasini Ardakani2020a, Reference Zare Pirhaji, Moeinpour, Mirhoseini Dehabadi and Yasini Ardakani2020b). Polyethyleneoxide/chitosan (PEO/CS) nanofibers were used to immobilize MNPs and HNTs via electrospinning. The nanofibrous adsorbents possessed a maximum adsorption capacity of ~120, 160, and 150 mg g–1 under an initial concentration of 100 mg L−1 at 45°C for Cd(II), Pb (II), and Cu(II), respectively (Li et al., Reference Li, Wang, Lv, Liu, Zhang and Shao2018b).
Several research projects reported the elimination of Pb2+ from wastewaters (Alguacil et al., Reference Alguacil, Alcaraz, García-Díaz and López2018; Nonkumwong et al., Reference Nonkumwong, Ananta and Srisombat2016). This metal ion belongs to the hazardous heavy metal class. Pb2+ is very stable in nature and if its concentration in the body exceeds 0.1 mg L–1, it can cause anemia and damage the nervous system. MHNT-manganese oxides (MHNTs-MnO2) were applied successfully to the rapid removal of Pb2+ from an aqueous solution. MnO2 was used due to its greater affinity for many heavy metal ions. The uptake capacity of Pb2+ by MHNTs-MnO2 ( ̴ 60 mg g–1) was about three times greater than that of MHNTs (̴ 20 mg g–1) (Afzali & Fayazi, Reference Afzali and Fayazi2016). In other research, polyamide-amine (PAMAM) has been loaded on the surfaces of MHNTs to provide a large amine-group density. The maximum adsorption capacity of this material for Pb(II) was up to 194.4 mg g−1. After six cycles, MHNTs-PAMAM still retained 90% of the maximum adsorption capacity. The adsorption mechanism consists of the coordination between the internal tertiary amines and the sulfhydryl groups with the Pb cation and, when pH > 4, the adsorption capacity increased due to the reducing species of H3O+ in water (Cheng et al., Reference Cheng, Dai, Chen, Cui, Qiang, Sun and Dai2019). Similarly, MHNTs-alginate beads with a 1:2 ratio possess a large adsorption capacity for Pb(II) which was up to 125 mg g−1 (Polat & Açıkel, Reference Polat and Açιkel2019).
Mercury (Hg2+) is one of the most widely studied pollutants and its discovery in environmental samples is attracting significant research attention. Exposure to Hg2+ can damage the central nervous system and vital body organs (Selvaraj et al., Reference Selvaraj, Rajalakshmi, Ahn, Yoon, Nam, Lee, Xu, Song and Lee2021). A simple magnetic electrochemical sensing protocol using MHNTs-MnO2 and magnetic carbon paste electrodes where the Hg2+ can be absorbed by MHNT composites was reported by Fayazi et al. (Reference Fayazi, Taher, Afzali and Mostafavi2016). The MHNTs composites-Hg2+ was brought to the surface of the electrode where the ions are detected electrochemically by applying differential pulse voltammetry. The recovery of metal ions from real water samples was up to 102.7%. In the concentration range 0.5–150 µg L–1 Hg(II), the detection limit was very small, 0.2 µg L−1. The results were comparable to other previously modified electrochemical methods such as carbon paste electrode modified magnetic nickel zinc ferrite nanocomposites (8.0 µg L−1) (Afkhami et al., Reference Afkhami, Sayari, Soltani-Felehgari and Madrakian2015) and glassy carbon electrode modified ion imprinted polymeric-carbon nanotubes (1 µg L−1) (Rajabi et al., Reference Rajabi, Roushani and Shamsipur2013).
Variable-Valence Heavy Metal Ions
For the environmental samples mentioned above, the variable-valence heavy metal ions adsorbed by MHNT nanocomposites are chromium (Cr(III) and Cr(VI)), antimony(Sb(V) and Sb(III)), and arsenic (As(III) and As(V)).
Exposure of the human body to a low level of arsenic (i.e. drinking water containing As) can lead to serious health problems such as chronic poisoning and cancer because arsenic can combine with sulfhydryl groups and hydroxyl groups in the molecular structure of enzyme proteins and thus affect directly the body’s physiological function (Jomova et al., Reference Jomova, Jenisova, Feszterova, Baros, Liska, Hudecova, Rhodes and Valko2011). Magnetic HNTs showed a high adsorption capacity of 408.71 and 427.72 mg g–1 for As(III) and As(V), respectively (Song et al., Reference Song, Zhou, Zhang, Chen and Yang2019). The elimination pathways of As are attributed to the inner-sphere complex formation and As(V) reduction with simultaneous Fe(II) oxidation (Maziarz et al., Reference Maziarz, Matusik, Leiviskä, Strączek, Kapusta, Woch, Tokarz and Gorniak2019).
A porous adsorbent with HNTs, Fe3O4, and carbon was produced to enhance the removal efficiency of arsenic by using polyurethane foam waste as the carbon source and structure template for HNTs dispersing and MNPs loading. The adsorbent showed an excellent uptake capacity of 1491.72 mg g− 1 toward As(V) due to the porous structure of HNTs/C/Fe3O4 and the good dispersion of HNTs and iron oxide, which facilitate the transmitting of arsenic ions and provide more binding sites (Song et al., Reference Song, Wang, Zhou, Luo and Liu2021).
The permitted level of Cr(VI) in environmental wastewaters is limited to 200 µg L–1 due to its potential carcinogenicity (Tian et al., Reference Tian, Wang, Tian, Zhou, Yang and Komarneni2016; Zhitkovich, Reference Zhitkovich2011). Cr(VI) is more harmful to the environment than Cr(III). Typically, the removal of this toxic element consists of reducing the most toxic Cr(VI) species and immobilizing the resulting low-toxicity Cr(III) moieties (Xia et al., Reference Xia, Song, Jeyakumar, Shaheen, Rinklebe, Ok, Bolan and Wang2019). For example, oxygen-containing organic groups and iron oxide nanoparticles were coated on the surface of HNTs for the removal of Cr(VI). The Fe3O4/HNTs-C produced exhibited a maximum adsorption capacity of 132 mg g–1, which is ~100 times greater than that of HNTs alone (<10 mg g–1). The adsorption mechanism was explained as follows: (1) MNPs and organic carbon species reduce part of the Cr(VI); (2) the resulting Cr(III) can be attracted to the adsorbent surface by electrostatic attraction and anchored on the surface of halloysite by the chelation effect of organic functional groups (i.e. carboxyl, aldehyde, and hydroxyl); and (3) the remaining negatively charged Cr(VI) ions could be attached to the positively charged surface of adsorbent by electrostatic attraction and ion exchange (Tian et al., Reference Tian, Wang, Tian, Zhou, Yang and Komarneni2016).
Silane-modified MHNTs were also reported as having an acceptable uptake capacity for Cr(VI) in an aqueous solution which was up to 59.90 mg g–1 (Table 6 ) (Zhu et al., Reference Zhu, Duan, Wang, Gao, Jia, Ma and Wang2017). However, the removal ability of this adsorbent was smaller than those found for Fe3O4/HNTs-C (Tian et al., Reference Tian, Wang, Tian, Zhou, Yang and Komarneni2016) and electrospun membrane-based MHNTs (77.10 mg g–1) (Li et al., Reference Li, Wang, Lv, Liu, Zhang and Shao2018b).
Table 6 Summary of the application of MHNT nanocomposites to various heavy metal ions
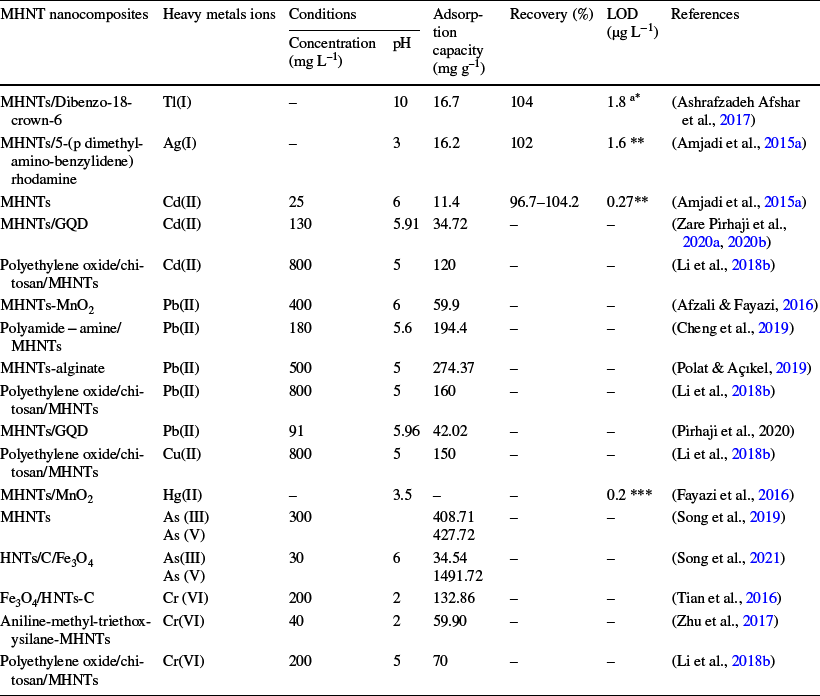
ang L−1
Detection techniques: *ETAAS; **flame atomic absorption spectrometry (FAAS) and differential pulse voltammetry (DPV)
The co-adsorption feasibility of Cr(VI) and Sb(V) was tested using silane-modified MHNTs where the adsorption mechanism was explained as follows: (1) Cr(VI) ions are attached to adsorbent surfaces through anion–π, functional-group interactions and electrostatic interaction; (2) Sb(V) can be adsorbed through Cr(VI)–O–Sb(V) interaction on the modified MHNTs surface, and (3) Cr(VI) and Sb(V) could form Cr (VI)–O–Sb(V) complexes in solution, which are subsequently adsorbed onto the adsorbent by complexation reactions. Therefore, the coexistence of Cr(VI) could enhance Sb(V) adsorption where the maximum Sb(V) adsorption capacity was found to be 30.49 and 53.06 mg g–1(Table 6) in the absence and the presence of Cr(VI), respectively (Zhu et al., Reference Zhu, Duan, Wang, Gao, Jia, Ma and Wang2017).
Application of MHNT and MHNT Nanocomposites to Radioactive Ions
The use of oil and fossil fuels leads to the pollution of water by the release of a large quantity of radioactive materials into nature. Radionuclides, such as uranium (U), are very toxic (Todorov & Ilieva, Reference Todorov and Ilieva2006). Therefore, U(VI)-bearing effluents must be treated effectively before being discharged into the environment. Adsorption of U(VI) onto MHNTs was studied by He et al. (Reference He, Chen, Zhang, Hu, Wang and Wang2015).The experimental results indicated that MHNTs had the largest adsorption capacity of 88.32 mg g–1 for UO2 2+. The removal capacity was achieved by ion exchange and surface complexation (He et al., Reference He, Chen, Zhang, Hu, Wang and Wang2015). ß-cyclodextrin was grafted onto MHNTs and batch adsorption techniques for U were performed (Yang et al., Reference Yang, Jiao, Zhou, Chen and Jiang2013b). The removal percentage by CD/HNT/iron oxide of uranium ions in simulated wastewater was 92% at neutral pH, showing that CD/MHNT is a promising adsorbent of uranium. The large sorption capacity of uranium was attributed mainly to the multiple hydroxyl sites of surface-grafted ß-CD (Yang et al., Reference Yang, Zong, Hu, Sheng, Wang and Wang2013a). The elimination of other toxic radioactive ions, such as europium (Eu) and iodine(I) by MHNT composites warrants further research.
MHNT and MHNT Nanocomposites for Extraction and Detection of Pharmaceuticals, Agricultural Pollutants, Proteins, and Mycotoxins
Bioactive pollutants including pesticides and other pharmaceutically active compounds such as antibiotics are the cause of major concern. These chemicals have been blamed for contamination of freshwater and food sources, threatening water and food security. Pollution by these pollutants has hit various countries harder as a consequence of less stringent legislation on waste discharge from agricultural and pharmaceutical industries (Aylaz et al., Reference Aylaz, Kuhn, Lau, Yeung, Roy, Duman and Yiu2021). On the other hand, therapeutic monitoring of drugs in biological fluids using a sensitive technique is necessary for controlling its efficacy in patients (Dramou et al., Reference Dramou, Zuo, He, Pham-Huy, Zou, Xiao and Pham-Huy2013).
Determination of any drug or contaminant in complex matrices by chromatographic or spectrophotometric methods needs sample pre-treatment to eliminate any potential interference. Currently, magnetic solid-phase extraction is a practice used commonly for sample clean-up because of its properties such as simplicity, the fact that it takes relatively little time, requires just a small amount of solvent, and is relatively cheap (Wu et al., Reference Wu, Zhao, Wang, Tang, Zhao, Niu, Yu, Yang, Fang, Lv and Liu2021). Consequently, non-selective and selective nanocomposite-based MHNTs have been developed for the separation of various kinds of pharmaceuticals, agricultural pollutants (e.g. antibiotics, flavonoids, and pesticides), mycotoxins, and biomolecules, e.g. proteins.
Non-Selective Nanocomposite-based MHNTs
Several composite-based MHNTs have been tested to assess their adsorption performance for various types of analytes. For example, MHNTs appeared to have a relatively good removal capacity for tetracycline from an aqueous solution. In contrast, chitosan-modified MHNTs showed low uptake capacity (Guan et al., Reference Guan, Wang, Pan, Lei, Zhou, Lu and Yan2012; Ma et al., Reference Ma, Dai, Dai, Da and Yan2016b). Thus, chitosan modification is not recommended for the removal of tetracycline. In addition, the extraction of tetracycline from areal matrix by the nanomaterials above was not evaluated.
Recently, a MHNT/AuNPs substrate for rapid and efficient MSPE surface-enhanced Raman scattering (SERS) on-site detection of nitrofuran antibiotics in real samples was developed. MHNT was used because of its good dispersibility and rapid enrichment. The substrate exhibited excellent SERS activity and maximum extraction ability, within 5 min. The detection limit of nitrofuran in aquatic samples was 0.014 mg L–1 and the recovery was up to 116.3% (Zhang et al., Reference Zhang, Lai, Wu, Li and Hu2020). In work by Dramou et al. (Reference Dramou, Wang, Sun, Zhang, Yang, Liu and He2022), a novel composite magnetic graphene oxide-MHNT (Fig. 10) was used for the extraction of rutin from Swanson health products due to its large adsorption uptake, up to 50 mg g–1. This was greater than the update for either MGO or MHNTs when they were used as single adsorbents, as their dispersion characteristics could be enhanced by the composite because the introduction of MHNTs can expand the lamellar structure and reduce the aggregation of MGO efficiently. On the other hand, the MGO boosts the MHNTs adsorption capacity. The LOD (limit of detection) and LOQ (limit of quantification) of rutin using the MGO-MHNTs-HPLC method were 0.0325 and 0.0975 μg mL–1, respectively. In fact, wastewaters or biological media are complex and contain interferences. Developing highly stable and selective adsorbents for contaminant removal and drug detection from the aforementioned samples has always been the motivation in the field of MSPE.

Fig. 10 Synthesis of MGO-MHNTs (reproduced from Dramou et al. (Reference Dramou, Wang, Sun, Zhang, Yang, Liu and He2022) with the permission of Elsevier)
Selective Nanocomposites: MHNT-Imprinted Polymers
Molecularly imprinted polymers (MIPs) are considered to be smart polymers which have been proven an effective approach for clean-up and preconcentration of various analytes from complex matrices (Guć et al., Reference Guć, Messyasz and Schroeder2021). MIPs can uptake selectively the target molecule from a complex medium due to the recognition sites and imprinted cavity distributed in a three-dimensional network (Li et al., Reference Li, Zha, Niu, Hu, Hui, Tang, Fizir and He2018a). To attain the recognition sites, the target analyte as the template molecule with functional monomers and crosslinker agent participates in the reaction. Based on the lock-key concept, an imprinted cavity and recognized sites were obtained after eliminating the template molecule from the designed polymer matrix, and they can select and extract the target analyte (Fizir et al., Reference Fizir, Dahiru, Cui, Zhi, Dramou and He2021).
HNTs possess superior characteristics compared to other non-support materials used such as CNTs or GO (Dramou et al., Reference Dramou, Fizir, Taleb, Itatahine, Dahiru, Mehdi, Wei, Zhang and He2018). For example, modification of CNTs is necessary prior to use because they show poor dispersibility in the organic phase or aqueous phase. By contrast, HNTs disperse well in water (Fizir et al., Reference Fizir, Richa, He, Touil, Brada and Fizir2020). What is more, a HNT can load molecules into its lumen which can improve the loading capacity of the MHNT composites (Fizir et al., Reference Fizir, Richa, He, Touil, Brada and Fizir2020; Yamina et al., Reference Yamina, Fizir, Itatahine, He and Dramou2018). Given that HNTs possess these excellent performances, magnetic HNTs can be a good candidate and suitable alternative support or matrix for MIPs (Li et al., Reference Li, Zha, Niu, Hu, Hui, Tang, Fizir and He2018a).
One of the most significant merits of the coating of MHNTs with MIPs (MHNTs-MIPs) is their dramatically enhanced selectivity and adsorption capacity. Many drawbacks of traditional MIPs such as poor site accessibility for templates, slow mass transfer, laborious centrifugation or filtration, and template leakage could be solved by applying MHNTs-MIPs where the separation and recovery of absorbents are facilitated by using an external magnetic field (Fizir et al., Reference Fizir, Richa, He, Touil, Brada and Fizir2020). The preparation methods of MHNTs-MIPs were discussed in a previous review (Fizir et al., Reference Fizir, Richa, He, Touil, Brada and Fizir2020).
MHNTs-MIPs have been used as solid-phase extraction adsorbents for the separation of pharmaceutically active compounds, agricultural contaminants, mycotoxins, and proteins. In this section, the selectivity and adsorption capacity of these nanomaterials are discussed and highlighted.
Separation of Pharmaceutical Active Compounds
A series of novel selective imprinted polymer-based MHNTs was designed for the adsorption and detection of tetracycline, norfloxacin, chloramphenicol, sulfamethazine, metoclopramide, and quercetin from environmental wastewaters and biological samples, e.g. serum and urines. Methacrylic acid and EGDMA were used as a monomer and a cross-linker, respectively, to prepare MHNTs-MIPs through the co-precipitation process for tetracycline adsorption where imprinted polymers with 35 nm of shell thickness showed the greatest and fastest adsorption capacity of 21.50 mg g–1 (Table 7) in 10 min due to the ultrathin imprinted polymer shell. MHNTs-MIPs showed significant stability and continuous superior performance for adsorbing tetracycline even after eight adsorption/desorption cycles (Dai et al., Reference Dai, Wei, Cao, Zhou, Yu, Pan, Zou, Li and Yan2014). Reportedly, MHNTs-MIPs prepared with the template molecule and the methacrylic acid (MAA) monomer at a ratio of 1:6 exhibited an excellent selective adsorption capacity of chloramphenicol (24.44 mg g–1), excellent regeneration property, and stability. The dominant adsorption mechanism is hydrogen bonds between carboxylic acid groups of monomer and chloramphenicol (He et al., Reference He, Zou, Chen, Dai, Xie, Zhou and Yan2016a, Reference He, Chen, Zhang, Hu, Wang and Wang2016b). Ma et al. (Reference Ma, Zhou, Dai, Qin, Ye, Chen, Xie, Yan and Li2016a, Reference Ma, Dai, Dai, Da and Yan2016b) synthesized a novel biomimetic Setaria viridis-inspired-hydrophilic magnetic surface molecularly imprinted core–shell nanorods (denoted as HMMINs). Using MHNTs as carriers enhanced significantly the specific binding of as-prepared MIPs toward sulfamethazine, 10.47 mg g–1 (Table 7). However, the preparation includes many steps which are time-consuming (Ma et al. Reference Ma, Zhou, Dai, Qin, Ye, Chen, Xie, Yan and Li2016a, Reference Ma, Dai, Dai, Da and Yan2016b).
Table 7 Experimental conditions for detection and extraction of various kinds of analytes by selective and non-selective MHNT composites and their analytical performance
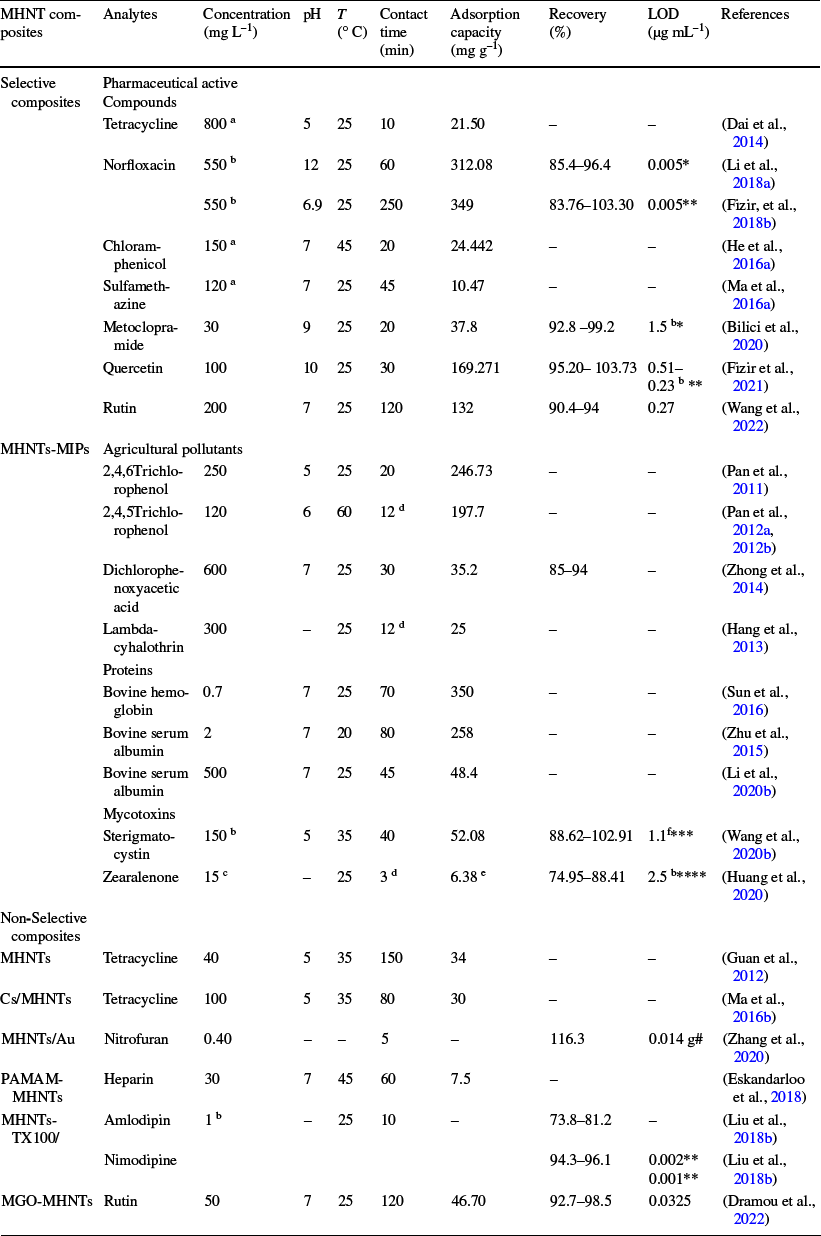
aµmol L−1; bµg mL–1; cng mL–1;dhours; eng/mg; fμg kg–1; gmg L–1. Detection techniques: *UV–Vis, ** HPLC/UV–Vis, ***HPLC–DAD, ****HPLC-FLD and # Surface-enhanced Raman scattering
With the enhancement of research on pharmaceutically active compounds, in addition to the adsorption performance of the materials in question, it is still important to have stable adsorption performance in a complex matrix. Hence, MHNTs-MIPs combined with high-performance liquid chromatography (HPLC–UV) has been applied for solid-phase extraction of norfloxacin in serum and lake water samples. Besides the excellent removal capacity and selectivity of prepared MIPs, the method provided good sensitivity (the LOD was <0.0006 µg L–1 in the real samples studied) and excellent recoveries of ~83% (Fizir et al., Reference Fizir, Wei, Muchuan, Itatahine, mehdi, He and Dramou2018b). To simplify the imprinting process and avoid the vinyl modification of MHNTs, a sol–gel method was used to prepare MHNTs-MIPs for extraction of norfloxacin. Similar findings (to those in the research mentioned above) were made using the MHNTs-MIPs-UV method (Li et al., Reference Li, Zha, Niu, Hu, Hui, Tang, Fizir and He2018a). Subsequently, the application of the above-produced MHNTs-MIPs was extended to the separation and detection of quercetin from serum and urine samples. APTES and TEOS were used as monomer and cross-linker agents, respectively. The MHNTs-MIPs-HPLC method showed a high sensitivity for quercetin; the limits of detection were 0.51 ng mL−1 in serum and 0.23 ng mL−1 in urine (Fizir et al., Reference Fizir, Dahiru, Cui, Zhi, Dramou and He2021).
MHNTs-MIPs prepared through surface-initiated reversible addition-fragmentation chain transfer polymerization could adsorb 37.8 mg of metoclopramide g–1 of adsorbent with an imprinting factor of 4.51, which means that the imprinted polymers possess excellent selectivity. In the concentration range of 5.0–150.0 ng mL−1 metoclopramide in urine samples, the limit of detection of MHNTs-MIPs coupled UV–Vis was calculated to be 1.5 ng mL−1. The results were comparable with other detection techniques such as HPLC, fluorescence, and UV–Vis where the LOD of the drug was >0.51 μg mL–1 (Bilici et al., Reference Bilici, Badak, Zengin, Suludere and Aktas2020).
In the first attempt to increase further the magnetism and loading capacity of MHNTs-MIP, it was combined with MGO via electrostatic interaction for rutin extraction. As shown in Fig. 11, strong magnetism (26.398 emu g–1), greater selectivity (IF = 2.25), and maximum adsorption capacity were obtained (132 mg g–1). The MGO/MHNTs-MIPs-HPLC method proved its efficiency for rutin detection and extraction from juice samples (Wang et al., Reference Wang, Ni, Zhang, Zhang, Jia, He and Dramou2022).
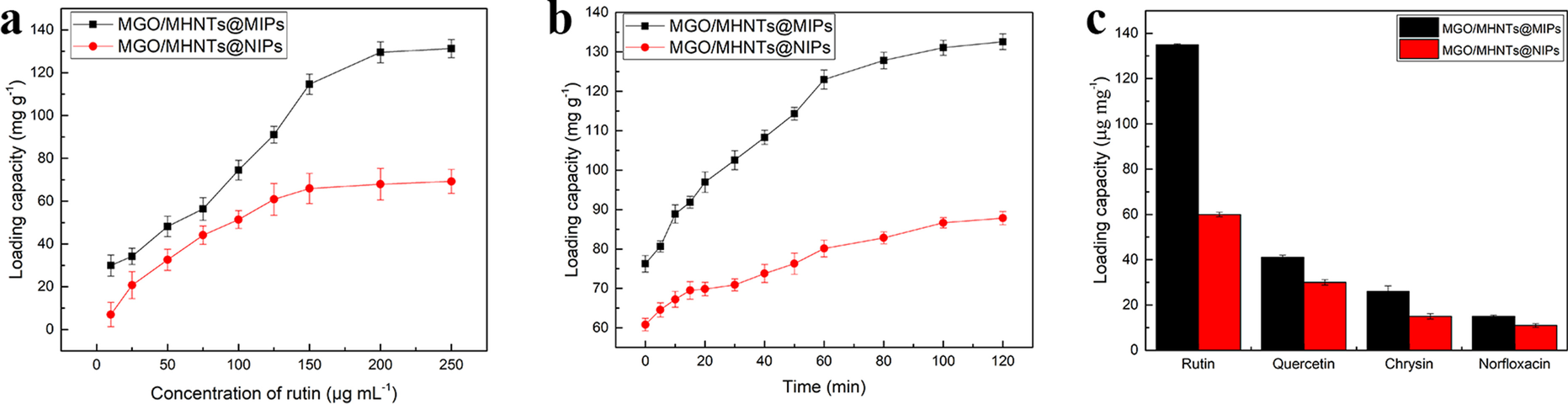
Fig. 11 a The loading isotherm curve of MGO/MHNTs-MIPs and MGO/MHNTs-NIPs; b the kinetic loading curve of MGO/MHNTs-MIPs and MGO/MHNTs-NIPs; c the selectivity of MGO/MHNTs-MIPs and MGO/MHNTs-NIPs (reproduced from Wang et al. (Reference Wang, Ni, Zhang, Zhang, Jia, He and Dramou2022) with the permission of Elsevier)
Separation of Agricultural Pollutants
A great many pesticides, including fungicides, herbicides, and insecticides are applied in agriculture in numerous countries. These agricultural pollutants can pollute water resources with carcinogens and other toxic substances that can affect humans if they are improperly selected and managed (Mateo-Sagasta et al., Reference Mateo-Sagasta, Zadeh, Turral and Burke2017). MHNTs-MIPs were used as adsorbents for the removal of these pollutants, e.g. trichlorophenol, dichlorophenoxyacetic acid, and lambda-cyhalothrin. Imprinted polymer-based MHNTs showed an excellent binding capacity of 246.73 mg g–1 toward trichlorophenol. Hydrogen bonds between trichlorophenol and methacrylic acid were the dominant recognition mechanism (Pan et al., Reference Pan, Yao, Xu, Ou, Huo, Li and Yan2011). In order to monitor the recognition and release of the analyte by imprinted materials, N-isopropylacrylamide (NIPAM) as a temperature-responsive monomer was used to prepare temperature-responsive, molecularly imprinted MHNTs. The maximum adsorption capacity of trichlorophenol was 197.7 mg g–1 at 60°C and its release was at 20°C (Pan et al., Reference Pan, Hang, Dai, Dai, Huo and Yan2012a, Reference Pan, Wang, Dai, Dai, Hang, Ou and Yanb). In work by Zhong et al. (Reference Zhong, Zhou, Zhang, Zhou, Li, Zhu and Wang2014), 4-vinylpyridine was used as a monomer and divinylbenzene as across-linker to prepare MHNTs-MIPs for removal of dichlorophenoxyacetic acid from a real sample. The recovery of analyte in <30 min by the MHNTs-MIPs-HPLC method was ~ 85–94% (Zhong et al., Reference Zhong, Zhou, Zhang, Zhou, Li, Zhu and Wang2014) which was greater than that of CNTs-MIP where the recovery was <81% due to the retaining capacity of analyte in the lumen of HNTs, which does not exist in CNTs (Yang et al., Reference Yang, Jiao, Zhou, Chen and Jiang2013b).
Lambda-cyhalothrin is a non-systemic pyrethroid insecticide and is highly active against a broad spectrum of pests in agriculture. Lambda-cyhalothrin residues in the environment have an adverse effect on human life because they can harm the central nervous system (Garcia et al., Reference Garcia, Scheffczyk, Garcia and Römbke2011). Thus, the detection and removal of these pollutants are very important (Pan et al., Reference Pan, Zhu, Dai, Yan, Gan, Li, Hang and Yan2014). MHNTs-MIPs prepared by Pickering emulsion polymerization using MAA as a monomer showed better specific recognition and selectivity for lambda-cyhalothrin in a mixed solution with a relatively large adsorption capacity of 25 mg g–1 (Hang et al., Reference Hang, Li, Pan, Li, Dai, Dai, Yu and Feng2013). The use of MHNTs-MIPs for solid-phase extraction of pyrethroid in real samples warrants further research.
Separation of Proteins
The extraction of purified proteins has attracted considerable interest because of their increasing significance in various applications ranging from diagnostics to therapeutics (Pei et al., Reference Pei, Wang, Wu, Xuan and Lu2009). The performance of the MHNTs-MIP for protein removal was investigated in various reports. For example, imprinted polydopamine on the surface of hydrophobic MHNTs prepared by Pickering emulsion polymerization showed a greater adsorption capacity (350 mg g–1 at 0.7 mg L–1) of bovine hemoglobin and better affinity than non-imprinted polymers with a high imprinting factor of 3.24. Hydrophobic interaction, electrostatic interaction, π–π bonds, and van der Waals forces are the main interactions between the cavity formed and the target protein (Sun et al., Reference Sun, Chen, Li, Li, Zhu, Hu, Huang, Li and Zhong2016). The adsorption capacity of MHNTs-MIPs is greater than other MIPs prepared by dopamine (Jia et al., Reference Jia, Xu, Wang, Ran, Yang and Zhang2013; Shen et al., Reference Shen, Zhou and Ye2012) which may be attributed to the more available binding site in the lumen of HNTs and magnetic NPs. On the other hand, compared to imprinted polydopamine-MHNTs, bovine serum albumin was retained less by MHNTs-MIPs prepared by N-isopropylacrylamide and methacrylic acid as bifunctional monomers (Zhu et al., Reference Zhu, Li, Zhou and Zhong2015) or by N-isopropylacrylamide (NIPAM), acrylic amide (AAM), and N-(3-aminopropyl) methyl acrylamide hydrochloride (APM) as functional co-monomers (Li et al., Reference Li, Huang, Huang, Lin and Huang2020a), proving that dopamine is a suitable monomer for protein recognition.
Separation of Mycotoxins
Mycotoxins are toxic metabolites produced by fungi on crops such as maize, sorghum, and wheat and they have adverse effects on humans and animals due to their toxicity and carcinogenic effects (Wang et al., Reference Wang, He, Song, Zhang, Xu, Huang, Jin, Ba, Li, You and Zhang2020a). Mycotoxins may result in an economic crisis if they are not detected and removed from food samples (Zain, Reference Zain2011).
Recently, MHNTs-MIPs were used successfully for the detection and extraction of some mycotoxins, e.g. sterigmatocystin and zearalenone from food samples. As proof of concept, the limit of detection of zearalenone in maize samples using pseudo-hollow MHNTs-MIPs-coupled HPLC with a fluorescence detector (HPLC-FLD) method was 2.5 ng mL−1 with satisfactory recoveries ranging from 74.95 to 88.41%. The chromatogram of the real sample after zearalenone removal, using the aforementioned method, contained some impurities which did not exist in the chromatogram of the immunoaffinity column (IAC). However, the retention time of analyte is not affected (Huang et al., Reference Huang, He, Li, Zhang, Wang, Zhang, Li, You and Zhang2020). In addition, a one-time use column and high cost are considered as drawbacks which limit IAC applications (Wang et al., Reference Wang, He, Song, Zhang, Xu, Huang, Jin, Ba, Li, You and Zhang2020a). Consequently, MHNTs-MIPs used as stable, low-cost, and simple methods proved to be promising techniques for myctoxins removal from food samples.
Thermo-responsive MHNTs-MIP and MCNTs-MIP showed greater affinity for sterigmatocystin than MCNTs-MIPs which may be attributed to the possibility of destroying the structures as well as the mechanical properties of the MCNTs after carboxylation modification, which affects the adsorption property of MCNTs-MIPs. Consequently, HNTs are a good alternative to CNTs. Furthermore, HNTs are cheap and readily available without complex fabrication, unlike CNTs (Wang et al., Reference Wang, Wu, Cui, Fizir, Shi and He2020b).
MHNT and MHNT Nanocomposites for Extraction and Detection of Phenol and Polycyclic Aromatic Hydrocarbons
Organic pollutants, such as polycyclic aromatic hydrocarbons (PAHs) and phenolic compounds, are listed as priority contaminants in wastewater, food, and soil with toxic effects on both plants and animals. The remediation of these toxic compounds has been an active area of research in the field of environmental science (Zango et al., Reference Zango, Sambudi, Jumbri, Ramli, Abu Bakar, Saad, Rozaini, Isiyaka, Osman and Sulieman2020). Various studies have investigated the application of MHNT nanocomposites as potential adsorbents for these pollutants. γ-Fe2O3/HNTs removed phenol efficiently from aqueous solutions with a relatively acceptable adsorption capacity of 24.33 mg g–1 and high regeneration ability where the composite could remove ~80% of phenol even after five adsorption/desorption cycles (Mirbagheri & Sabbaghi, Reference Mirbagheri and Sabbaghi2018). A hybrid nanocomposite with MHNTs, polyaniline, and copper (MHNT-PANI-Cu) exhibited a better removal capacity of nitrated polycyclic aromatic hydrocarbons (nitrophenanthrenes) than MHNTs; this was attributed to the following: (1) PANI can interact with analytes via π–π and hydrophobic interactions; and (2) transference between π electrons of nitrophenanthrenes and copper. The detection limit of MHNT-PANI-Cu-coupled Gas Chromatography-Mass Spectrometry (GC-MS) was 0.25 ng L−1 in soil and wastewater samples after <1 min of extraction (Darvishnejad & Ebrahimzadeh, Reference Darvishnejad and Ebrahimzadeh2018) which is more sensitive than the MHNT-PANI-GC/MS method whereby the LODs of polycyclic aromatic hydrocarbons in beer sample were in the range 1.64–14.20 ng L−1 (Shi et al., Reference Shi, Pang, Chen, Wu and Zhang2020). In conclusion, modifying MHNTs with PANI-Cu composites plays a vital role in the enhancement of extraction efficiency of organic pollutants.
Conclusions and Future Perspectives
This review presents a snapshot of the current state of research on MHNTs and the efforts that govern their development in analytical fields. Firstly, the design strategies of MHNTs were clarified, including in situ growth, nano-encapsulation, and direct mixing methods. Meanwhile, the synthesis conditions, saturation magnetization, and the size of MNP of the MHNTs prepared by various methods have been summarized in the tables, which allows the reader to choose the appropriate synthesis approach based on the experimental demand. In addition, the common identification methods and characteristics of MHNTs have been described briefly, and these can be used to verify the successful synthesis of MHNTs. The surface functionalization of MHNTs is an important step in improving their properties (such as adsorption efficiency, stability, and selectivity) and even endows them with new properties (such as electrochemical properties). Therefore, the surface functional modification from the types of functional agents and their combination modes with MHNTs have been introduced in detail. Finally, the analytical applications of MHNT and MHNT nanocomposites were reviewed. The main analytes extracted (mentioned here) include antibiotics, pesticides, proteins, carcinogens such as polycyclic aromatic hydrocarbons (PAHs), dyes, radioactive ions, and heavy metal ions such as Cu(II), Cd(II), Pb(II), Hg(II), Cr(III), and Cr(VI), found in environmental, biological, and food matrices.
Although research on MHNTs has made great advances, the following problems and challenges in its preparation still need to be resolved.
(1) Shape heterogeneity of MNPs on the surface of HNTs. Fortunately, the present review has shown that by comparing the characteristics of various synthesis approaches, the most appropriate method can be selected to reduce shape heterogeneity. To avoid agglomeration and further reduce heterogeneity, the strong recommendation that emerged is to use a mass ratio of iron salts to HNTs in the range of 1:1 to 3:1 and an agitation time of 4 h in the synthesis.
(2) Excess use of harmful products in the preparation of MHNTs. The review revealed that synthesis strategies can be simplified further and optimized based on the theories of conventional techniques.
(3) The effect of agitation speed on the physicochemical characteristics of MHNTs should be explored further. When the problems above in the preparation of MHNTs are solved, and then they are functionalized to form complexes, better properties and wider applications can be achieved. For example, MHNT composites showed a maximum adsorption capacity and satisfactory extraction performance for the aforementioned analytes. In particular, MHNTs offered promise as an adsorbent for the removal of uranium. Hence, the elimination of other toxic radioactive ions, such as europium and iodine, by MHNTs composites could warrant further research. Compared with conventional SPE adsorbents, the integration of MNPs with HNTs can offer extra advantages such as very stable structural forms and rapid extraction from the sample medium. The modification of MHNTs by imprinted polymers means that this nanomaterial has a high selectivity and plays a vital role in the enhancement of the extraction efficiency of organic pollutants. On the other hand, MHNTs-MIPs bound by magnetic graphene oxide are promising as a potential magnetic solid-phase extraction agent. Thus, further research on the preparation of MGO/MHNTs-MIPs using green monomers and their application for the enrichment and extraction of various kinds of analytes in real samples needs to be explored.
Acknowledgements
The authors acknowledge the financial support of the University Research-Training Projects of Algeria through the Grant No. A16N01UN440120220001.
Funding
Funding sources are as stated in the Acknowledgements.
Declarations
Competing Interest
The authors declare that they have no known competing financial interests or personal relationships that could have appeared to influence the work reported in this paper.