- CHr
reticulocyte Hb content
- IDA
Fe-deficiency anaemia
- IDE
Fe-deficient erythropoiesis
- MCV
mean corpuscular volume
- NPNL
non-pregnant non-lactating
- RCF
erythrocyte folate
- sTfR
soluble transferrin receptor
- TC
transcobalamin
- tHcy
total homocysteine
- ZnPP
zinc protoporphyrin
Adequate micronutrient nutrition is essential during pregnancy to ensure optimal fetal growth(Reference Fall, Yajnik, Rao, Davies, Brown and Farrant1). Whilst a wide range of micronutrient deficiencies is commonly found in developing countries(2), concern in countries such as the UK is focused on a few micronutrients such as folate, vitamin B12, Fe and vitamin D(Reference Relton, Pearce and Parker3–Reference Javaid, Crozier, Harvey, Gale, Dennison, Boucher, Arden, Godfrey and Cooper6).
Pregnancy is a time of huge changes in maternal physiology, several of which may influence micronutrient concentrations and biomarkers to an extent not seen in non-pregnant non-lactating (NPNL) women(Reference Morkbak, Hvas, Milman and Nexo7, Reference Milman, Bergholt, Byg, Eriksen and Hvas8). Ethical constraints often prevent indicators of micronutrient status from being rigorously assessed in pregnant women because of the invasive nature of most gold standard methods. In the absence of specific validation studies there remains a danger that normal assessment methods and reference ranges will be assumed to be valid and that interpretation will not account for the adaptive maternal, placental and fetal responses to increased micronutrient demands during pregnancy. The present review discusses the assessment of Fe, folate, and vitamin B12 status in pregnant women and describes the methods used to screen routinely for maternal anaemia caused by prolonged deficiency of any of these micronutrients.
Routine antenatal screening for anaemia caused by micronutrient deficiency
Anaemia can be defined as an insufficient erythrocyte mass to deliver adequate amounts of O2 to peripheral tissues(Reference Greer, Foerster, Lukens, Rodgers, Paraskevas and Glader9). Maintenance of the erythrocyte mass is limited by the ability of maturing erythroblasts to acquire sufficient amounts of Fe, folate and vitamin B12 for the synthesis of DNA and haem(Reference Koury and Ponka10). These micronutrients can be mobilised from body stores, absorbed from the diet or recycled from senescent erythrocytes(Reference Koury and Ponka10, Reference Knutson and Wessling-Resnick11). During pregnancy the demand for micronutrients, especially Fe and folate, is greatly increased and maternal body stores and dietary intake may be insufficient to meet demand. Inadequate supply of these micronutrients to the bone marrow results in dysfunctional erythropoiesis and ultimately anaemia.
In many industrialised countries routine antenatal care involves screening for anaemia at the initial booking appointment, normally between 10 weeks and 20 weeks of gestation, and at 28 weeks of gestation(12). In the UK screening relies heavily on the full blood count, an array of haematological indices that provides information on the number, size and Hb content of the erythrocyte population. An abnormal result or suspicion of increased risk justifies the use of more precise methods to identify underlying problems. Most patients, however, are assessed using only full blood count data.
Mean corpuscular volume: the multi-purpose screening tool
The mean corpuscular volume (MCV) is used to screen for folate, vitamin B12 and Fe deficiency and is measured as part of the full blood count. Poor folate or vitamin B12 status increases MCV by impairing DNA synthesis and cell division, whereas Fe deficiency decreases MCV as a result of impaired Hb production(Reference Greer, Foerster, Lukens, Rodgers, Paraskevas and Glader9). The normal range in NPNL adult women is 80–100 fl, although during pregnancy this level is elevated slightly because of the physiological increase in the proportion of younger larger erythrocytes(Reference Milman, Bergholt, Byg, Eriksen and Hvas8, Reference Lurie13). When screening for Fe-deficiency anaemia (IDA), MCV is considered alongside Hb and packed cell volume.
The detection of anaemia by MCV tends to occur only after functional deficiency lasting several weeks or more, since it takes time to repopulate the erythrocyte mass with enough cells of abnormal size to affect the mean markedly. Sensitivity is further reduced during pregnancy since the physiological increase in MCV can potentially mask the early stages of microcytosis(Reference Milman, Bergholt, Byg, Eriksen and Hvas8). It is therefore most useful at the initial booking appointment since it can identify pre-existing micronutrient deficiency that can be treated in time to prevent further deterioration(14–Reference Nybo, Friis-Hansen, Felding and Milman16). During late pregnancy, however, it may fail to detect individuals with recently-developed deficiency brought about by the high demand for micronutrients(Reference Rusia, Flowers, Madan, Agarwal, Sood and Sikka17, Reference van den Broek, Letsky, White and Shenkin18).
Hb: the maternal oxygen-carrying capacity
The conceptus is totally dependent on the maternal supply of O2 for its own respiration and this supply is delivered to the placenta, bound to Hb, at a rate governed by several factors including cardiac output, ventilation rate, uterine blood flow, smoking, altitude and packed cell volume(Reference Carter19). Insufficient supply of Hb to the placenta caused by maternal anaemia can potentially result in fetal hypoxia with serious adverse consequences for fetal development(Reference Mostello, Chalk, Khoury, Mack, Siddiqi and Clark20).
Impaired Hb production during pregnancy is most often caused by poor Fe status, and therefore measurement of Hb serves as a useful screening method for the detection of IDA. Assessment is complicated by the physiological expansion of the plasma volume during early pregnancy and the subsequent increase in erythrocyte mass(Reference Lund and Donovan21, Reference Orsi and Tribe22). The net result of these haematological changes is a 5–15% dilution of the maternal blood supply, which reduces blood viscosity and improves substrate delivery to the placenta(Reference Rasmussen23, Reference Chesley24). This process is called the ‘physiological anaemia of pregnancy’ and does not reflect any deterioration in maternal Fe status or O2-carrying capacity.
There is considerable debate as to what constitutes the optimal Hb range during pregnancy. The US Centre for Disease Control has classified maternal anaemia based on data from healthy Fe-supplemented mostly-Scandinavian pregnant women(Reference Svanberg, Arvidsson, Norrby, Rybo and Solvell25–Reference Sjostedt, Manner, Nummi and Ekenved28). Using the 5th percentiles from these groups to define the limit of the normal range, the Centre for Disease Control has defined anaemia as Hb <110 g/l during the 1st and 3rd trimesters and <105 g/l during the 2nd trimester. Similarly-derived cut-off points have also been established for packed cell volumes. A more recent dataset of several hundred healthy pregnant Danish women has found similar results. Normal ranges (g/l), defined as means±1·96 sd, were: 118 (105–132) at 18 weeks of gestation, 118 (103–134) at 32 weeks of gestation, and 124 (108–140) at 39 weeks of gestation(Reference Milman, Bergholt, Byg, Eriksen and Hvas8). These values are assumed to represent a healthy range of values within these populations, since no clinically-significant adverse pregnancy outcomes were recorded. Hb concentrations below these lower cut-off points are considered likely to reflect maternal IDA to some extent.
The effects of uncomplicated IDA in human pregnancy are difficult to ascertain since ethical considerations often prevent controlled studies from being carried out. One alternative is to observe the relationships between IDA and pregnancy outcomes in free-living populations. Unfortunately, such studies are confounded by factors such as inadequate haemodilution(Reference Hytten29, Reference Salas, Marshall, Gutierrez and Rosso30), smoking(Reference Leifert31), chronic inflammation(Reference van den Broek and Letsky32), infection(Reference Muhangi, Woodburn, Omara, Omoding, Kizito, Mpairwe, Nabulime, Ameke, Morison and Elliott33, Reference Geelhoed, Agadzi, Visser, Ablordeppey, Asare, O'Rourke, van Leeuwen and van Roosmalen34) and low BMI(Reference Geelhoed, Agadzi, Visser, Ablordeppey, Asare, O'Rourke, van Leeuwen and van Roosmalen34), all of which have strong independent effects on pregnancy outcome. Most studies have reported the optimal Hb range in mid-to-late pregnancy to include mild anaemia as defined by Centre for Disease Control values (Hb 95–110 g/l)(Reference Chang, O'Brien, Nathanson, Mancini and Witter35–Reference Steer38) and a few have even questioned the risks associated with moderate or severe IDA (Hb <95 g/l and <80 g/l respectively), since the numerous observational studies that have examined this issue are highly inconsistent(Reference Rasmussen23).
An alternative method is to examine the effects of maternal anaemia in highly-controlled animal models. Most of these studies have used ovine models, although in studies that have been carried out in human subjects using non-invasive techniques the results have generally been in agreement(Reference Mostello, Chalk, Khoury, Mack, Siddiqi and Clark20, Reference Edwards, Novy, Walters and Metcalfe39, Reference Delpapa, Edelstone, Milley and Balsan40). These results show adaptive responses by both mother and fetus to acute falls in maternal packed cell volume that help to prevent maternal and fetal hypoxia. The affinity of Hb for O2 is reduced by increasing erythrocyte concentrations of 2,3-diphosphoglycerate, which allows more efficient uptake of O2 by maternal and fetal tissue(Reference Edwards, Novy, Walters and Metcalfe39, Reference Richardson and Bocking41). Increased maternal cardiac output and utero–placental blood flow maintains the rate of O2 delivery(Reference Mostello, Chalk, Khoury, Mack, Siddiqi and Clark20, Reference Delpapa, Edelstone, Milley and Balsan40) and increased fetal heart rate and blood pressure maintains the distribution of O2 to fetal tissue(Reference Pulgar, Zhang, Massmann and Figueroa42). In addition, both mother and fetus may reduce their rates of physical activity to levels that require less O2. Only when O2 delivery falls to below 50% of normal levels, or when conditions persist for several days, does the rate of fetal O2 consumption fall as a linear function of O2 delivery(Reference Paulone, Edelstone and Shedd43, Reference Bocking, White, Homan and Richardson44). Under such conditions the fetus adapts to the lower supply of O2 by up regulating erythropoiesis and down regulating fetal growth, both of which help to prevent the onset of hypoxia or acidosis in all but the most extreme conditions.
Whilst the effects of mild maternal anaemia remain highly controversial, moderate-to-severe anaemia in both animal models and human observational studies has been consistently associated with fetal growth restriction, probably mediated by chronically-impaired maternal O2-carrying capacity(Reference Mostello, Chalk, Khoury, Mack, Siddiqi and Clark20, Reference Richardson and Bocking41, Reference Mahajan, Aalinkeel, Shah, Singh and Kochupillai45, Reference Spinillo, Capuzzo, Piazzi, Nicola, Colonna and Iasci46). However, it remains unclear whether there is any discernable benefit of Fe therapy in terms of pregnancy outcomes, other than restoration of maternal Fe status(Reference Reveiz, Gyte and Cuervo15). The continuing practice of prophylactic Fe supplementation is based on the general consensus that Fe deficiency is by definition undesirable and that its prevention is more likely to be beneficial than harmful(47). Maternal supplementation during pregnancy is also likely to improve neonatal Fe status and thus protect the infant from IDA, especially if born preterm(47–Reference Singla, Gupta and Agarwal49).
Assessment of maternal body iron stores
Body Fe stores are located mostly in the reticuloendothelial cells of the bone marrow, liver and spleen, as well as in hepatocytes(Reference Andrews50). The largest pool of Fe is contained in the maternal erythrocyte mass, which is constantly turned over and recycled. The most precise methods for the assessment of body Fe stores are quantitative phlebotomy(Reference Haskins, Stevens, Finch and Finch51), liver biopsy(Reference Barry52), MRI(Reference Gandon, Olivie, Guyader, Aubé, Oberti, Sebille and Deugnier53) and bone marrow staining(Reference Finch, Hegsted, Kinney, Thomas, Rath, Haskins, Finch and Fluharty54). However, the routine use of such techniques during pregnancy would be both unethical and expensive.
Fortunately, body Fe stores can be measured quite accurately using serum ferritin. Ferritin serves as the body's main intracellular Fe storage protein. Trace amounts of mostly Fe-free ferritin are secreted into the plasma from reticuloendothelial or parenchymal cells in concentrations that linearly reflect total body Fe stores(Reference Finch, Bellotti, Stray, Lipschitz, Cook, Pippard and Huebers55). In NPNL women a serum ferritin concentration of 1 μg/l is equivalent to approximately 7–8 mg mobilisable Fe(Reference Walters, Miller and Worwood56). Thus, a serum ferritin concentration of 50 μg/l indicates adequate Fe stores (350–400 mg) for the day-to-day needs of most NPNL women. However, as >500 mg Fe stores is required to prevent depletion of Fe stores during the average pregnancy(Reference Bothwell57), only serum ferritin concentrations of >70–80 μg/l in early pregnancy are likely to reflect sufficient Fe stores able to prevent depletion without the need for supplementation(Reference Casanueva, Pfeffer, Drijanski, Fernandez-Gaxiola, Gutierrez-Valenzuela and Rothenberg58, Reference Bentley59).
The interpretation of serum ferritin is based not on reference data from healthy individuals, as with the detection of IDA, but rather on the concentrations at which depleted body Fe stores can be predicted with great confidence. In NPNL individuals a serum ferritin of <15–16 μg/l identifies the absence of stainable bone marrow with 75% sensitivity and 98% specificity(Reference Milman, Pedersen and Visfeldt60, Reference Hallberg, Bengtsson, Lapidus, Lindstedt, Lundberg and Hulten61). Only one study has carried out similar assessments of pregnant women(Reference van den Broek, Letsky, White and Shenkin62). In this study the most accurate cut-off point in late pregnancy was determined to be 30 μg/l, which is markedly higher than that found in NPNL women. Sensitivity and specificity at this level are 90·0% and 85·1% respectively, compared with 37·5% and 93·7% for 12 μg/l; data for the commonly-used cut-off at 15 μg/l were not presented.
The higher cut-off point found in this study may have been a result of the high levels of inflammation in the sample. Pregnancy is associated with a physiological increase in inflammatory biomarkers, especially during the 1st and 3rd trimesters(Reference Mor63, Reference Brewster, Orsi, Gopichandran, McShane, Ekbote and Walker64). Serum ferritin becomes elevated during inflammation because of its role as an acute-phase reactant, and therefore may overestimate body Fe stores(Reference Rogers, Bridges, Durmowicz, Glass, Auron and Munro65). High serum ferritin can also result from damage to ferritin-rich tissues that releases it into the maternal circulation, such as can occur in cases of pre-eclampsia(Reference Hubel, Bodnar, Many, Harger, Ness and Roberts66). Inflammation and infection tend to affect the entire serum ferritin distribution, not just those individuals with high concentrations(Reference Beard, Murray-Kolb, Rosales, Solomons and Angelilli67) and therefore, whenever possible, serum ferritin should be measured alongside inflammatory markers such as C-reactive protein, α1-antichymotrypsin, α1-acid glycoprotein or erythrocyte sedimentation rate(Reference Thurnham, Mburu, Mwaniki, Muniu, Alumasa and de Wagt68, Reference Khusun, Yip, Schultink and Dillon69).
The relationship between serum ferritin and pregnancy outcome is confounded by several of the same factors that complicate the assessment of anaemia. Maternal infection, inflammation and hypertensive disorders are all associated with a higher incidence of preterm delivery and fetal growth restriction(Reference Goldenberg, Tamura, DuBard, Johnston, Copper and Neggers70–Reference Bartha, Romero-Carmona and Comino-Delgado73). Thus, whilst it appears from observational studies that serum ferritin concentrations <30 μg/l during the second half of pregnancy are protective(Reference van den Broek, Letsky, White and Shenkin62), this is most probably because the risks associated with these confounders are much greater than those associated with depletion of maternal Fe stores. It is unlikely that there are any beneficial effects of maternal Fe stores depletion per se, since healthy women supplemented with Fe from early pregnancy experience no increase in adverse pregnancy outcomes and are less likely to become Fe deficient(Reference Makrides, Crowther, Gibson, Gibson and Skeaff74).
Assessment of iron-deficient erythropoiesis
Fe-deficient erythropoiesis (IDE) is the synthesis of erythrocytes deficient in Hb as a result of a lack of available Fe. The main difference between IDE and IDA is that IDE reflects current erythrocyte production whereas IDA reflects the state of the erythrocyte population as a whole. As only 1–2% of the erythrocyte mass is replaced each day, it can take several weeks of IDE before its effects become clinically apparent in the form of anaemia. Conversely, once effective Fe therapy begins IDE will cease yet IDA can persist for several weeks until a substantial proportion of the erythrocyte population is replaced by normochromic normocytic cells(Reference Conrad and Crosby75).
Although the reduced O2-carrying capacity caused by IDE may be compensated by an array of adaptive responses, depletion of tissue Fe has widespread, if not clinically-apparent, effects on the maternal metabolism. Reductions in cellular Fe concentrations may adversely affect the function of a wide range of Fe-dependent tissue enzymes such as catalases, peroxidises and cytochromes(Reference Letsky76, Reference Beard77). The early detection and treatment of IDE is therefore important not only to prevent the onset of IDA, but also to ensure optimal Fe-dependent metabolism throughout pregnancy.
Erythrocyte zinc protoporphyrin
The most established biomarker of IDE is zinc protoporphyrin (ZnPP), which measures the extent to which Zn, rather than Fe, has been chelated with protoporphyrin. Increasing amounts of ZnPP are produced in maturing erythroblasts as Fe availability becomes suboptimal in the bone marrow. This process may function as a homeostatic mechanism by inhibiting the excretion of Fe following haemolysis by macrophages(Reference Maines78). Thus, it is a highly-sensitive functional indicator of IDE, especially when presented as ZnPP:haem, as this variable controls for haemodilution during pregnancy(Reference Schifman, Thomasson and Evers79). ZnPP remains constant throughout pregnancy in Fe-replete women, whereas in unsupplemented women it tends to rise markedly in the last trimester(Reference Romslo, Haram, Sagen and Augensen80, Reference Milman, Ibsen and Christensen81).
Although ZnPP measures IDE with reasonable accuracy, it cannot distinguish between true and functional Fe deficiency. The latter occurs when body Fe stores are adequate yet Fe is not available to the bone marrow, such as can happen during infection and inflammation. The underlying cause of IDE may be identified by concurrent assessment of serum ferritin. For instance, if both measures are high then IDE caused by chronic inflammation is more likely, whereas if ZnPP is high (≥60 μmol/mol haem) and serum ferritin is low (<15 μg/l) then true IDE caused by depleted Fe stores is more likely. This combined use of ZnPP and ferritin values has been recommended by several authors as a reliable method for the determination of IDE(Reference Hastka, Lasserre, Schwarzbeck and Hehlmann82, Reference Labbe, Vreman and Stevenson83). However, it should be noted that functional and true IDE are not mutually exclusive processes and may co-exist, especially during late pregnancy when low-level inflammation with depleted Fe stores is a common occurrence. In such circumstances the relative contributions of the underlying causes of IDE may be difficult to quantify.
The main advantages of ZnPP are its cost efficiency and its ability to be used in the field by unspecialised personnel using a portable haematofluorometer. However, its use is limited by its high sensitivity to environmental Pb pollution(Reference Lamola and Yamane84), which inhibits the binding of Fe to protoporphyrin, and its poor sensitivity in identifying improvements once Fe therapy has begun. This limitation might be resolved if the assay could be refined to measure only reticulocyte ZnPP, as has been suggested by some authors, and initial crude efforts to this effect have shown considerable promise(Reference Kleven, Blohowiak and Kling85). Despite its advantages, however, the lack of an automated method for assessment of ZnPP has severely constrained its use in most clinical laboratories.
Reticulocyte indices
Reticulocytes are immature erythrocyte cells that under normal conditions comprise about 1% of the erythrocyte population. They mature in the bone marrow for 1–3 d and are then released in the maternal circulation for 1–2 d before they lose their RNA and become fully mature. Reticulocyte counts have been available in most clinical laboratories for many years as a measure of the rate of erythropoiesis. However, a few modern haematological analysers now have the ability to measure the Hb content of reticulocytes, which effectively measures the extent of IDE over the previous 3–4 d(Reference Brugnara86).
Reticulocyte Hb content (CHr) is similar in some aspects to ZnPP. It is a cellular measure of IDE that on its own does not distinguish between true and functional Fe deficiency. However, unlike ZnPP, analysis is restricted to reticulocytes, so its sensitivity to short-term changes in Hb production is much greater(Reference Thomas and Thomas87). Values <26–28 pg identify IDE in NPNL adults(Reference Fishbane, Galgano, Langley, Canfield and Maesaka88).
CHr works by assessment of the reticulocyte cell volume and Hb content; hence conditions that change MCV independently of Fe deficiency will lower the precision of Chr(Reference Mast, Blinder and Dietzen89). Such conditions include microcytosis caused by thalassaemia and macrocytosis caused by folate or vitamin B12 deficiency(Reference Mast, Blinder, Lu, Flax and Dietzen90). Unfortunately, concurrent Fe and folate deficiency is relatively common during late pregnancy(Reference d'Onofrio, Chirillo, Zini, Caenaro, Tommasi and Micciulli91). Whilst initial validation studies seem to suggest that CHr is an accurate method of assessing IDE in pregnancy, the effects of suboptimal folate or vitamin B12 status may be important sources of error during pregnancy that require further study.
CHr is essentially a short-term indicator, but it can now be measured alongside the percentage of hypochromic erythrocytes, which until recently had only been available by manual blood film(Reference Brugnara86). Together CHr and the percentage of hypochromic erythrocytes appear to offer the most accurate assessment of past and present IDE and IDA during pregnancy(Reference Ervasti, Kotisaari, Heinonen and Punnonen92). However, determination of the underlying cause of IDE may require additional assessment of tissue Fe levels.
Assessment of maternal tissue iron depletion
The maintenance of serum Fe concentrations is dependent on the export of Fe from storage cells such as the duodenal mucosa, macrophages and hepatocytes(Reference De Domenico, McVey and Kaplan93). As these stores become depleted, the proportion of serum transferrin saturated with Fe (transferrin saturation) decreases. In response, the serum concentration of transferrin (the total Fe-binding capacity) is increased to promote uptake and delivery of Fe to dependent tissues such as the bone marrow or placenta. The classical signs of tissue Fe deficiency are therefore low serum Fe (<400 μg/l), high serum total Fe-binding capacity (<2160 μg/l), and low transferrin saturation (<16%)(47, Reference Huebers and Finch94).
By mid–late pregnancy tissue Fe deficiency is common as a result of sustained demand for Fe during the expansion of the erythrocyte mass and development of the conceptus. However, during the last trimester (27–40 weeks) the fetus starts to grows at its maximal rate and accumulates Fe stores in preparation for infancy(Reference Singla, Gupta and Agarwal49, Reference Bothwell57). If during this period maternal serum Fe concentrations are suboptimal, placental Fe uptake may be maintained by up-regulation of placental transferrin receptors(Reference Georgieff, Berry, Wobken and Leibold95, Reference Li, Yan and Bai96) and modulation of Fe regulation in the maternal gut(Reference O'Brien, Zavaleta, Abrams and Caulfield97). However, such mechanisms are not always able to compensate sufficiently to prevent reduced rates of fetal Fe accretion(Reference Singla, Gupta and Agarwal49, Reference Halvorsen98). Accurate identification of maternal tissue Fe deficiency during pregnancy is therefore essential to prevent suboptimal Fe status in the infant.
Serum total Fe-binding capacity is increased by steroid hormones such as oestrogens(Reference McKnight, Lee, Hemmaplardh, Finch and Palmiter99), which during pregnancy are found in high concentrations in maternal plasma. Even Fe-replete women with constant serum Fe tend to show increasing total Fe-binding capacity and falling transferrin saturation with advancing gestation(Reference Romslo, Haram, Sagen and Augensen80, Reference Malkasian, Tauxe and Hagedorn100–Reference Morgan102). As a result it may be preferable during pregnancy to concentrate entirely on the direct measurement of serum Fe(Reference Singla, Gupta and Agarwal49, Reference Nhonoli, Kihama and Ramji103).
Serum Fe measures the transferrin-bound Fe available for maternal and placental uptake. It has a high level of intra-individual and diurnal variability, and may reflect recent dietary intake, especially following use of supplemental Fe(Reference Beaton, Corey and Steele104, Reference Hoppe, Hulthen and Hallberg105). It is highly reactive to infection and inflammation since both Fe uptake from the gut and Fe release from macrophages are down-regulated by the action of hepcidin, a regulator of Fe homeostasis(Reference Knutson, Oukka, Koss, Aydemir and Wessling-Resnick106). These limitations may be overcome to some extent by sufficient sample size, careful study design and repeated measurements. However, its susceptibility to such large day-to-day fluctuations makes it a crude indicator of maternal tissue Fe status and of little diagnostic utility in the individual.
A general summary of Fe biomarkers during pregnancy is shown in Table 1.
Serum soluble transferrin receptor
Soluble transferrin receptors (sTfR) are truncated versions of the transferrin receptors that facilitate cellular uptake of Fe-rich plasma transferrin. Expression of transferrin receptors is inversely related to that of intracellular ferritin, since both are mediated by Fe-responsive elements whose main purpose is to maintain intracellular Fe concentrations(Reference Klausner, Rouault and Harford107). Expression of transferrin receptors therefore increases as tissue Fe levels fall, resulting in higher concentrations of sTfR in the serum(Reference Skikne, Flowers and Cook108). The density of transferrin receptors is highest in cells with the greatest demand for Fe, such as immature erythroid cells, hepatocytes and the placenta(Reference Huebers and Finch109).
Table 1. Laboratory measures of maternal iron status
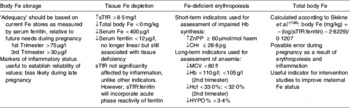
sTfR, soluble transferritin receptor; ZnPP zinc protoporphyrin; CHr, reticulocyte Hb content; MCV, mean corpuscular volume; Hct, packed cell volume; percentage of hypochromic erythrocytes; ↑, increased; ↓, reduced.
Serum sTfR has a high specificity to tissue Fe deficiency and a low sensitivity to acute or chronic inflammation, unlike most other indicators of Fe status(Reference Ferguson, Skikne, Simpson, Baynes and Cook110, Reference Dimitriou, Stiakaki, Markaki, Bolonaki, Giannakopoulou and Kalmanti111). When combined with measurement of serum ferritin, the sTfR:ferritin can be used to estimate total body Fe (mg/kg) with high precision(Reference Cook, Flowers and Skikne112).
However, the accuracy of sTfR and sTfR:ferritin during pregnancy has not yet been firmly established. As a large proportion of sTfR is derived from eythroid precursors in the bone marrow, changes in the rate of erythropoiesis also affect sTfR concentrations. In Fe-replete individuals sTfR is an accurate indicator of erythropoiesis(Reference Robach, Fulla, Westerterp and Richalet113), whereas in those individuals with a steady rate of erythropoiesis sTfR is an accurate indicator of tissue Fe depletion(Reference Metzgeroth, Adelberger, Dorn-Beineke, Kuhn, Schatz, Maywald, Bertsch, Wisser, Hehlmann and Hastka114).
Unfortunately, pregnancy is associated with marked changes in both erythropoiesis and body Fe stores that introduce considerable uncertainty as to the primary cause of changes in sTfR concentrations. There are few data on the normal patterns of erythropoiesis during pregnancy, mainly because of the prohibitive nature of radio Fe studies, and therefore it is not possible to adjust for the influence of erythropoiesis at each stage. During pregnancy raised sTfR may in fact be more strongly predicted by the rate of erythropoiesis than by Fe status(Reference Choi, Im and Pai115).
Some studies have reported sTfR to be a sensitive and specific measure of tissue Fe deficiency in pregnancy(Reference Akesson, Bjellerup, Berglund, Bremme and Vahter116, Reference Carriaga, Skikne, Finley, Cutler and Cook117), but these have not had the benefit of comparison with gold standard methods, as has been the case for NPNL subjects. sTfR and the sTfR:ferritin appear to have great potential as indicators of tissue Fe depletion, but their validity during pregnancy remains uncertain until the effects of erythropoiesis can be ascertained. In the meantime, sTfR:ferritin is probably of most use as a method of evaluating the effectiveness of interventions to improve Fe status during pregnancy, since the error as a result of erythropoiesis and inflammation ought to be similar in both control and intervention groups(Reference Cook, Flowers and Skikne112).
Direct assessment of folate status
Most pregnant women in the UK will only be screened for folate deficiency by assessment of MCV. However, such assessment is highly insensitive, especially in the presence of concurrent Fe deficiency, and is unable to specify the underlying cause. The most common method of directly assessing folate status is serum or plasma folate. This short-term indicator is highly sensitive to recent folate intake and indicates the amount of folate being taken up by tissues, catabolised and excreted at the point in time when the serum sample was drawn(Reference Herbert118). It is markedly and quickly decreased during negative folate balance, when tissue demands exceed that provided by dietary supply(Reference Sauberlich, Kretsch, Skala, Johnson and Taylor119).
The advantage of serum folate in studies of pregnant women is that it represents the concentration available for placental uptake at the time of blood sampling, whereas erythrocyte folate (RCF) represents average availability over several months. As folate demands change several fold between trimesters, analysis of serum folate allows time-specific determination of folate availability. In NPNL individuals serum folate and dietary folate intake are generally well correlated. However, during pregnancy this correlation may be weakened(Reference Takimoto, Mito, Umegaki, Ishiwaki, Kusama, Abe, Yamawaki, Fukuoka, Ohta and Yoshiike120).
RCF concentrations indicate the availability of folate at the time when the cell was forming in the bone marrow(Reference Bailey121). Once DNA synthesis ceases in maturing erythrocytes, the remaining folate concentrations are relatively static until erythrophagocytosis, when the folate is recycled. The mean RCF concentration therefore reflects a moving average of serum folate status over the 120 d lifespan of the erythrocyte population. As this period also approximately corresponds to the time in which hepatic folate stores become depleted on a folate-free diet(Reference Herbert, MF, ELR and JF122), RCF concentrations are assumed to correlate with those in tissue(Reference Herbert123); this correlation has been confirmed by examination of liver and RCF concentrations in subjects with chronic alcoholism(Reference Wu, Chanarin, Slavin and Levi124). However, the validity of this assumption during late pregnancy, when both folate and erythrocyte turnover are markedly different from that during the NPNL state(Reference Lurie125), remains untested.
RCF is standardised by packed cell volume and is therefore not confounded by variation in plasma volume expansion. Values <140–150 μg/l for RCF or <3 μg/l for serum folate are generally considered to be indicative of folate deficiency in the NPNL state(Reference Snow126).
Both serum folate and RCF are most often measured by methods that use high-affinity folate-binding proteins such as RIA, ion-capture assays and chemiluminescence. Microbiological assays using various strains of Lactobacillus casei have traditionally been used as the reference standard and some are now fully automated and cost efficient(Reference Molloy and Scott127). However, they can sometimes be confounded by antibiotics, the use of which may be relatively common during pregnancy, and are therefore not often used in clinical contexts.
Although folate-binding protein assays have been widely used, persistent doubts have been raised about their reliability(Reference Levine128–Reference Wilson, Williams, Herrmann, Wiesner and Brookhart130). A round-robin international survey of reputable laboratories found inter-assay CV of 32–41% with RCF and 17–48% with serum folate(Reference Gunter, Bowman, Caudill, Twite, Adams and Sampson131). The variability of high or low values was found to be much greater than that for mid-range concentrations. Much of the difference been attributed to the types of reference standards in commercial assays, which were found to vary considerably by manufacturer. Recent efforts to standardise these reference materials in whole blood(Reference Thorpe, Sands, Heath, Hamilton, Blackmore and Barrowcliffe132) and serum(Reference Thorpe, Heath, Blackmore, Lee, Hamilton, O'Broin, Nelson and Pfeiffer133) may substantially reduce inter-assay variability in the future, thus making comparison of absolute values between studies more reliable.
Although generally folate-binding-protein assays tend to underestimate folate status as a result of incomplete recovery of 5-methyltetrahydrofolate(Reference Fazili, Pfeiffer and Zhang134), they may actually overestimate folate status during pregnancy. A comparison of four different methods for assessing RCF in pregnant and non-pregnant women(Reference Clifford, Noceti, Block-Joy, Block and Block135) has reported major inter-assay differences, with RIA giving approximately 40% greater values than L. casei (Reference Clifford, Noceti, Block-Joy, Block and Block135). Whilst the folate status of pregnant and non-pregnant women were not found to be significantly different when measured using L. casei and GC–MS, the values for pregnant women according to the folate-binding-protein assays were found to be higher than those of the non-pregnant women.
A recently-developed method using isotope-dilution liquid chromatography–tandem MS now enables high-throughput analysis of folate status with high precision and the ability to differentiate between folate species(Reference Pfeiffer, Fazili, McCoy, Zhang and Gunter136). This method represents a considerable advance in the ability to examine the complex inter-relationships between folate species and may help to resolve the emerging debate about the risks associated with unmetabolised folic acid in plasma(Reference Cole, Baron and Sandler137, Reference Kim138). Recent comparative studies have shown excellent agreement between liquid chromatography–MS–MS and L. casei (Reference Fazili, Pfeiffer and Zhang134).
The lack of comparability between laboratories has led to the classification of inadequate folate status being generally based on comparison with reference data from local populations and not on symptoms of functional folate deficiency such as neutrophil hypersegmentation or macrocytosis. Such an approach may be preferable since during pregnancy these functional indices are less reliable than during the NPNL state. Neutrophils tend to hyposegment with advancing gestation, which can mask folate deficiency(Reference Gadowsky, Gale, Wolfe, Jory, Gibson and O'Connor139), and MCV may sometimes be more influenced by the microcytic effects of poor maternal Fe status during late pregnancy, as seen in pregnant adolescents from the About Teenage Eating Study (Fig. 1). However, the use of reference population data to determine folate status is complicated by the increasing amount of food now fortified with folic acid in modern diets, especially fortified flour, since normal ranges in some populations are now higher than those of previous generations(Reference Pfeiffer, Johnson, Jain, Yetley, Picciano, Rader, Fisher, Mulinare and Osterloh140). As a result, the use of normality-based approaches is not only incompatible between countries with different fortification strategies, but is unlikely to be a sensitive method of detecting functional folate deficiency in pregnancy.
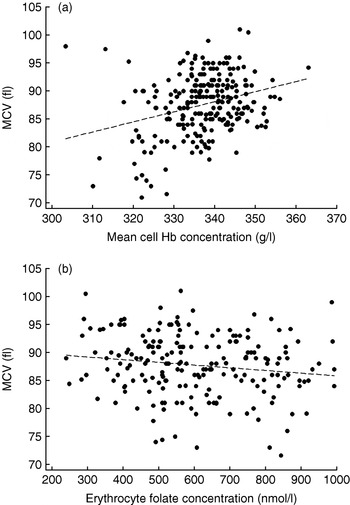
Fig. 1. Conflicting influences on mean corpuscular volume (MCV) in 239 pregnant adolescents from the About Teenage Eating Study measured during the third trimester of pregnancy. Mean cell Hb concentration was calculated as the Hb concentration divided by the packed cell volume; erythrocyte folate concentrations were similarly standardised. (a) r p 0·30, P<0·001; (b) r p −0·13, P=0·04. (Data from S. Wheeler, unpublished results.)
Functional biomarkers of folate status
Serum total homocysteine
Functional indicators can provide useful information that direct measurement of vitamin concentrations cannot, and are often more sensitive. A widely-used functional biomarker of mild or occult folate depletion is serum total homocysteine (tHcy). tHcy is primarily a functional marker of folate status in Western populations, but is also affected by the status of other nutrients such as vitamin B12, vitamin B6, methionine, betaine, choline and riboflavin, all of which are involved in homocysteine production or excretion(Reference McNulty, Dowey, Strain, Dunne, Ward, Molloy, McAnena, Hughes, Hannon-Fletcher and Scott141–Reference Refsum, Nurk, Smith, Ueland, Gjesdal, Bjelland, Tverdal, Tell, Nygård and Vollset143).
A major advantage of tHcy over serum folate is that it does not require fasting blood samples, the collection of which may be ethically prohibited in studies of pregnant women. Postprandial serum tHcy concentrations are generally slightly lower than fasted values but these differences are small compared with the total amount of day-to-day variation(Reference Thirup and Ekelund144, Reference Jacques, Rosenberg, Rogers, Selhub, Bowman, Gunter, Wright and Johnson145). Serum folate, on the other hand, can reflect recent intake, especially when consumed in relatively high doses such as those found in antenatal folic acid supplements(Reference Pentieva, McNulty and Reichert146).
During pregnancy the correlation between folate status and tHcy becomes weaker with advancing gestation. Serum tHcy falls markedly during the first 20 weeks of gestation to approximately half that of NPNL levels and then increases slowly back to pre-conceptual levels by parturition(Reference Cikot, Steegers-Theunissen, Thomas, de Boo, Merkus and Steegers147). This uncoupling with folate concentrations, which in unsupplemented subjects continue to fall throughout pregnancy, remains poorly understood. The decline in serum tHcy in the first half of pregnancy has been suggested to be the result of plasma volume expansion, increased renal clearance, decreased serum albumin and higher concentrations of oestrogens, most of which have been eliminated as causal factors(Reference Bartha, Romero-Carmona and Comino-Delgado73). Oestrogens have been suggested as the most probable mediators, but their homocysteine-lowering effects have recently been questioned(Reference Murphy, Scott, McPartlin and Fernandez-Ballart148, Reference Roopnarinesingh, Jackson, Osman, Harrison and Mayne149).
Despite its idiosyncratic behaviour during pregnancy, serum tHcy remains a sensitive functional biomarker of folate status in pregnant women. Precision may be further increased by adjustment for confounding factors such as renal function, smoking, vitamin B12 status and coffee intake(Reference Refsum, Smith and Ueland150, Reference Grubben, Boers, Blom, Broekhuizen, de Jong, van Rijt, de Ruijter, Swinkels, Nagengast and Katan151). A plasma tHcy concentration >15 μmol/l is generally used to define mild hyperhomocysteinaemia in NPNL women, but because of the physiological decrease in plasma tHcy concentrations during pregnancy, an upper reference limit of 10 μmol/l has been recommended by an expert panel(Reference Refsum, Smith and Ueland150). Whilst there have been problems with tHcy assessment in the past, most modern assays now have good levels of precision and comparability(Reference Refsum, Smith and Ueland150).
p-Amino- and acetamido-benzoylglutamate
The rate of folate turnover can be quantified by measurement of the folate catabolites p-amino-benzoylglutamate and acetamido-benzoylglutamate present in serum or urine(Reference McPartlin, Courtney, McNulty, Weir and Scott152). Until recently, the analysis procedure has required extensive sample preparation and clean up(Reference McNulty, McPartlin, Weir and Scott153), making it unsuitable for clinical or epidemiological use. However, recent innovations using liquid chromatography–MS–MS have simplified this process greatly, such that high-volume analysis of urinary and serum p-amino-benzoylglutamate and acetamido-benzoylglutamate may be both possible and cost effective in the future(Reference Sokoro, Etter, Lepage, Weist, Eichhorst and Lehotay154, Reference Hannisdal, Svardal and Ueland155). Analysis of p-amino-benzoylglutamate may also have potential as a biomarker of folate status in degraded blood samples(Reference Hannisdal, Svardal and Ueland155).
Measurement of folate turnover is not a measurement of folate status per se. It does, however, provide detailed information on an individual's folate requirement, since the amount excreted can be assumed to be the amount required to replace it in order to prevent depletion. The analysis of p-amino-benzoylglutamate and acetamido-benzoylglutamate, together with conventional assessment of folate status, may enable more detailed examination of the relationships between folate demands over the course of pregnancy and maternal folate status, which hitherto has been restricted to samples of less than ten individuals(Reference McPartlin, Courtney, McNulty, Weir and Scott152, Reference Gregory, Caudill, Opalko and Bailey156).
Poor folate status and pregnancy outcomes
The placenta is rich in high-affinity folate receptors that are able to maintain folate delivery to the fetus even at relatively low maternal plasma concentrations(Reference Wallace, Bonham and Strain142). However, cord and placental folate concentrations are still dependent on those in maternal plasma(Reference Baker, Frank, Deangelis, Feingold and Kaminetzky157). Poor folate status has been strongly, if somewhat inconsistently, associated with several adverse pregnancy outcomes, such as preterm delivery, pre-eclampsia, stillbirth, spontaneous abortions, placental abruption, fetal growth restriction and congenital birth defects(Reference Vollset, Refsum, Irgens, Emblem, Tverdal, Gjessing, Monsen and Ueland158–Reference Kosmas, Tatsioni and Ioannidis162). Raised tHcy is also associated with these risks, although it is not yet clear what proportions are the result of poor folate status per se or to the folate-independent actions of homocysteine(Reference Gregory, Caudill, Opalko and Bailey156, Reference Baker, Frank, Deangelis, Feingold and Kaminetzky157). The preventive effect of folic acid supplementation in substantially reducing the risk of neural-tube defects has been proven in several randomised controlled trials(163, Reference Czeizel and Dudas164). However, whilst the reduction in the incidence of neural-tube defects has been a tremendous accomplishment of nutrition research, it has also resulted in the situation in which it is now unethical to conduct placebo-controlled intervention trials to determine the effects of folic acid supplementation during pregnancy, since the peri-conceptual use of 400 μg folic acid is now part of routine antenatal care. Hence, the ability to test the associations between poor folate status and other pregnancy outcomes under controlled conditions is severely limited.
Assessment of vitamin B12 status
Serum cobalamin
The accurate detection of vitamin B12 deficiency during pregnancy is extremely difficult. In normal NPNL subjects and in patients with pernicious anaemia serum cobalamin generally correlates well with tissue concentrations, although kinetic studies have shown that concentrations in serum tend to be preserved at the expense of those in tissue(Reference Adams, Tankel and MacEwan165, Reference Boddy and Adams166). As a result, low serum cobalamin is only likely to become apparent with moderate or severe tissue deficiency. Serum cobalamin of <150 ng/l is generally associated with clinically-apparent deficiency in the NPNL state, yet the usefulness of this, or indeed any, cut-off during pregnancy is questionable(Reference Metz, McGrath, Bennett, Hyland and Bottiglieri167). Concentrations below this level have been found in pregnant women with dietary intakes well in excess of the US RDA of 2·6 μg/d and no corroborative clinical or biochemical evidence of deficiency(Reference Pardo, Peled, Bar, Hod, Sela, Rafael and Orvieto168, Reference Koebnick, Heins, Dagnelie, Wickramasinghe, Ratnayaka, Hothorn, Pfahlberc, Hoffmann, Lindemans and Leitzmann169).
Functional biomarkers: serum total homocysteine and serum methylmalonic acid
As serum cobalamin is less sensitive during pregnancy, mild or occult vitamin B12 deficiency may be more easily detected with functional biomarkers such as serum methylmalonic acid and serum tHcy. Vitamin B12 has only two functions in animal cells and impairment of either causes the accumulation of unmetabolised precursors. Methylmalonic acid becomes elevated as a result of the impairment of the cobalamin-dependent enzyme methylmalonyl-CoA mutase, which converts methylmalonyl-CoA to succinyl-CoA, an essential precursor of haem and an intermediate in the citric acid cycle. Serum tHcy accumulates as a result of the impairment of cobalamin-dependent methionine synthase(Reference Allen, Stabler, Savage and Lindenbaum170).
Although methylmalonic acid and tHcy are more sensitive than serum total cobalamins, both have major limitations during pregnancy. Serum tHcy can be elevated by folate deficiency, which is more common than vitamin B12 deficiency in Western countries, and becomes uncoupled from vitamin B12 status with advancing gestation. Methylmalonic acid is also affected by renal clearance as well as concurrent use of antibiotics(Reference Lindenbaum, Savage, Stabler and Allen171), and can sometimes be sporadically elevated during pregnancy for reasons as yet unknown(Reference Metz, McGrath, Bennett, Hyland and Bottiglieri167, Reference Pardo, Peled, Bar, Hod, Sela, Rafael and Orvieto168). Measurement of methylmalonic acid is also expensive and requires the use of techniques that may not be widely available in clinical laboratories. The use of either biomarker alone is therefore insufficient to determine vitamin B12 status during pregnancy, although they can provide useful supplemental data to other more direct measures.
Holo-transcobalamin
Cobalamins in human serum are transported by the two main binding proteins, haptocorrin and transcobalamin (TC). These proteins are either complexed with cobalamins (holo) or cobalamin-free (apo). Haptocorrin carries most of the cobalamins in serum, which are cleared slowly over a period of several days. However, no major function has yet been attributed to it other than the binding of metabolically-inert forms of vitamin B12. TC, on the other hand, is highly active and serves as the main transporter of cobalamins from the intestine to tissues including the placenta, which is rich in TC receptors(Reference Obeid, Morkbak, Munz, Nexo and Herrmann172). Newly-absorbed cobalamins bound to holo-TC are generally cleared from the maternal circulation within minutes, although a small amount remains in circulation.
Recent longitudinal analysis of TC and haptocorrin fractions during pregnancy has determined the reason for the decline in serum cobalamin with advancing gestation(Reference Morkbak, Hvas, Milman and Nexo7). Large reductions were found in the haptocorrin fraction saturated with true cobalamins, whereas holo-TC remained stable over the whole of gestation. This finding explains why the physiological reduction in serum cobalamin during pregnancy is not associated with functional vitamin B12 deficiency, as haptocorrin is relatively inert.
Since TC is the main fraction available for placental uptake of vitamin B12, maternal serum holo-TC concentrations are highly correlated with cord blood cobalamins and therefore reflect the maternal supply of vitamin B12 to the fetus(Reference Obeid, Morkbak, Munz, Nexo and Herrmann172). Whilst the high sensitivity and specificity of maternal holo-TC makes it the most suitable biomarker for the assessment of maternal vitamin B12 status, the assay required for its assessment is still highly-specialised and not yet widely available. Until such time as it is suitable for high-volume low-cost throughput of samples, routine screening for maternal vitamin B12 deficiency will continue to be carried out using crude indicators with low sensitivity and specificity such as MCV and serum cobalamin, leaving many pregnant women at risk of subclinical vitamin B12 deficiency.
Implications of poor vitamin B12 for fetal growth
With the notable exception of vegans, low vitamin B12 intakes are relatively rare in Western countries and consequently poor vitamin B12 status is normally a result of non-dietary factors. However, poor vitamin B12 status is still a major public health problem in developing countries with low consumption of animal foods, such as India and Nepal(Reference Refsum, Yajnik and Gadkari173, Reference Bondevik, Schneede, Refsum, Lie, Ulstein and Kvale174). Studies in these countries have found similar relationships between vitamin B12 status and fetal growth restriction to those found with folate(Reference Yajnik, Deshpande, Panchanadikar, Naik, Deshpande, Coyaji, Fall and Refsum175, Reference Muthayya, Dwarkanath, Mhaskar, Mhaskar, Thomas, Duggan, Fawzi, Bhat, Vaz and Kurpad176), although its relationships with other pregnancy outcomes remain unknown.
Summary
Interpretation of micronutrient biomarkers and indices during pregnancy is complex and should take into account the maternal, placental and fetal adaptations to pregnancy, most of which are highly variable between individuals and dependent on the gestational age at which measurement occurs. As a result of the complexity and uncertainty surrounding these factors, sensitivity and specificity of virtually all biomarkers will be reduced, especially during late pregnancy, and many of the normal ranges commonly used for NPNL individuals may be inappropriate. Current antenatal screening of micronutrient status in the UK is crude, as it is concerned primarily with the treatment rather than prevention of nutritional anaemia.
Future research should establish methods that can identify recent-onset functional deficiency without substantial bias from maternal inflammation, infection, endocrine influences, plasma volume and renal function. Several relatively new indicators of Fe status show much promise in this context, especially when used in combination. However, they require further validation in pregnant subjects. Assessment of folate status remains problematic because of issues surrounding the reliability and comparability of folate-binding-protein assays. However, new methods using liquid chromatography–MS–MS are able to measure specific folate species, including products of folate catabolism, with high precision and in large sample sizes. Similarly, measurement of serum holo-TC may provide much needed data on the relationships between vitamin B12 status and pregnancy outcome.
Acknowledgements
S. W. is funded by Tommy's the Baby Charity, UK registered charity no. 1060508. The author declares no conflict of interest.