- BMD
bone mineral density
- 25 OHD
25-hydroxyvitamin D
Bones break because the loads placed on them exceed their ability to absorb the energy involved(Reference Parfitt, Avioli and Krane1). This outcome is likely to be the result of a number of factors including, but not limited to: a reduction in bone mass; a change in the distribution of bone; loss of cancellous or cortical microarchitecture; an accumulation of damaged bone; a change in the material properties of the remaining bone(Reference Loveridge, Lanham-New, Mathers and Buttriss2).
Bone is a living tissue. There is a continuous cycle of active bone formation (through the activity of osteocytes and osteoblasts) and bone resorption (involving osteoclasts). Fig. 1 shows the sequence of events in the bone remodelling sequence. Osteoclasts are attracted to a quiescent bone surface and then excavate an erosion cavity. Mononuclear cells smooth off the erosion cavity, which is a subsequent site for the attraction of osteoblasts that synthesise an osteoid matrix. Continuous new bone matrix synthesis is followed by calcification of the newly-formed bone. When complete, lining cells once more overlie the trabecular surface(Reference Eastell and Favus3).

Fig. 1. Steps in the remodelling sequence of cancellous bone. Osteoclasts are attracted to a quiescent bone surface (A) and then excavate an erosion cavity (B, C). Mononuclear cells smooth off the erosion cavity (D), which is a subsequent site for the attraction of osteoblasts that synthesise an osteoid matrix (E). Continuous new bone matrix synthesis (F) is followed by calcification (G) of the newly-formed bone. When complete, lining cells once more overlie the trabecular surface (H). (From the National Osteoporosis Society(14); reproduced with permission.)
Osteoporosis is defined as a metabolic bone disease ‘characterised by low bone mass and microarchitectural deterioration of bone tissue, leading to enhanced bone fragility and a consequent increase in fracture risk’(4, 5). There is a normal mineral:collagen, unlike that of other metabolic bone diseases such as osteomalacia (which is characterised by deficiencies of mineral relative to collagen)(Reference Heaney, Abrams, Dawson-Hughes, Looker, Marcus, Matkovic and Weaver6). An example of osteoporotic and normal bone is shown in Fig. 2.

Fig. 2. Examples of normal and osteoporotic bone under the microscope. (From Eastell(3); reproduced with permission.)
Public health impact of poor bone health
Globally, it is estimated that one in three women and one in twelve men aged >50 years will suffer from osteoporosis in their lifetime, which roughly equates to three million individuals(Reference van Staa, Dennison, Leufkens and Cooper7). There are >230 000 osteoporotic fractures occurring every year in the UK at a cost of >£1·7×109 per year. In Europe the cost of treating osteoporotic fractures is an important contributor to the financial implications of osteoporosis generally (it is estimated that €13·9×109 is the expected annual total cost relating to osteoporosis)(Reference Dennison, Cooper, Lanham-New, O'Neill, Morris, Skeleton and Sutcliffe8). Specifically, hip fractures account for more than one-third of the total cost of osteoporosis and are a reflection of the inpatient (hospital) and outpatient (nursing home) care(Reference Torgerson, Iglesias and Reid9). Furthermore, the WHO has projected a rise in osteoporotic fractures from 1·66×106 hip fractures in 1990 to 6·26×106 in the 2050, which suggests that the future economic impact of osteoporosis will be enormous(10).
Changes in bone mass with ageing
Considerable changes in skeletal mass occur during the life cycle. Adult bone health is predominantly determined by three key factors: the maximum attainment of peak bone mass, which is achieved during growth and early adulthood; maintaining bone mass in adulthood; reducing the rate of bone loss with advancing age, with the menopausal years being a time of considerable concern for women (Fig. 3)(Reference Abrams11). Both peak bone mass attainment in the younger population and the rate of bone loss in post-menopausal women and the elderly are determined by key endogenous and exogenous factors, i.e. a combination of genetic, endocrine, mechanical and nutritional factors(Reference Smith, James and Garrow12), with evidence of extensive interactions within and between these groups.

Fig. 3. Changes in bone mass during the life cycle. Critical times are: (1) attainment of peak bone mass (PBM; 0–28 years of age, with pubertal years being particularly crucial); (2) menopause (; during the menopause and ⩽10 years post menopause it is estimated that 1–2% of bone is lost per year); (3) age-related bone loss (a low bone mineral density threshold increases osteoporosis fracture risk).
Calcium nutrition: important concepts
Ca is the most abundant mineral in the body(13). Approximately 1·2 kg (equivalent to about 300 mmol) is contained within the human body, with 99% of this Ca being located within the bones and teeth. Ca is also located in body fluids and soft tissues. It has two key roles: (1) supporting structural integrity; (2) regulating metabolic function(14). Ca is essential for: cellular structure; intercellular and intracellular metabolic function; signal transmission; muscle contractions, including heart muscle; nerve function; activities of enzymes; normal clotting of blood. There is no functional marker of Ca status, since its role in normal blood clotting takes priority and hence plasma Ca is maintained within very narrow limits (Fig. 4)(15).

Fig. 4. Regulation of calcium levels in blood and tissue. 1,25 (OH)2D3, 1,25-dihydroxycholecalciferol. (From Smith(12); reproduced with permission.)
Ca metabolism
Ca absorption occurs predominantly in the jejunum and also in the ileum and colon. Uptake occurs by active transport and simple passive diffusion. At low Ca intakes active transport predominates, but as intakes increase more is absorbed by non-specific pathways(16). The metabolite of vitamin D (1,25-dihydroxycholecalciferol) stimulates Ca transport across the intestinal cells by inducing the production of a Ca-binding protein. This process occurs within the villus cells through the normal process of receptor binding, DNA interaction and messenger RNA production. Hence, vitamin D is critical for effective Ca absorption(17).
Ca is lost mainly through renal excretion but there are data to also support losses occurring via faeces, sweat, skin and hair, as well as nails. Ca enters the gut via the bile, which is known to be relatively rich in Ca. It also enters the gut in pancreatic secretions and as part of desquamated cells from the mucosal lining. It may also be reabsorbed from the ileum and colon. As a result of the endogenous secretion the net absorption is less than gross dietary absorption by approximately 100 mg. Plasma Ca is tightly maintained at a level of 90–105 mg/l, of which approximately 50% is ionised. The plasma ionised Ca compartment is maintained by a combination of humeral factors that regulate intestinal absorption, renal loss and deposition or mobilisation of Ca from bone(Reference Prentice, Schoenmakers, Laskey, de Bono, Ginty and Goldberg18).
Urinary Ca excretion
Approximately 97% of the filtered Ca load is re-absorbed by the renal tubules and the remaining 3%, which is excreted in urine, represents the obligatory loss. The major determinant of urinary Ca excretion is dietary Ca intake. Urinary Ca excretion is higher when protein and Na intakes are high than when intakes of these two nutrients are low. The effects of a high protein intake on Ca excretion may be partly offset by higher P intake. Urinary Ca excretion decreases in old age with glomerular filtration rate. Also, intestinal absorption declines as the result of a reduction in the efficiency of parathyroid hormone and vitamin D metabolism. Increased urinary Ca in women at the menopause reflects the increased mobilisation of bone Ca because of oestrogen deficiency(Reference Abrams19).
Control of Ca balance
An adult on a normal mixed diet is usually in a state of equilibrium, i.e. the amount lost in the faeces and urine is approximately equal to the amount present in the food. In growing children the body is normally in positive balance. Ca is steadily maintained for the formation of new bone. When the need of the bones is great the net absorption of dietary Ca via the intestinal mucosa can be much greater than normal. The main features of Ca balance include a miscible pool mainly in blood and extracellular fluids, which provides for the slow turnover in bones. The pool is replenished by dietary Ca and losses occur in urine.
It is very important to note that the concentration of plasma Ca is finely regulated and controls the size of the pool. Ca balance is hence actively controlled by a large number of factors. The external balance of Ca (the difference between intake and output) is, in effect, determined by the exchange between the skeleton, the intestine and the kidney. These fluxes are controlled by the action of calciotrophic hormones: parathyroid hormone; 1,25-dihydroxycholecalciferol; calcitonin. It is also influenced by other factors such as sex hormones, growth hormones, corticosteroids and a variety of locally-acting hormones (Fig. 4)(Reference Ward, Roberts, Adams, Lanham-New and Mughal20).
Within bone, Ca is in the form of hydroxyapatite crystals (Ca10 (PO4)6 (OH)2), which also contain P and Mg, and contributes to its strength. P is found in abundance in a variety of foods but Mg is more limited and is an area of bone health nutrition that requires further attention.
Calcium and peak bone mass development
Peak bone mass is one of the key factors determining bone mass and fracture risk later in life. Attaining a high peak bone mass within an individual's genetic potential is a key preventative strategy for fracture reduction. Hence, the focus of research must be more fully directed on modifiable factors affecting the peak attainment of bone. It is known that genetic factors do play a key role in the variation of peak bone mass, with estimates of approximately 70–75%(Reference Ralston, Lanham-New, O'Neill, Morris, Skeleton and Sutcliffe21), which still leaves plenty of room for key exogenous factors such as diet and physical activity to play a key role in bone mass development.
The length of time peak mass is maintained before age-related bone loss begins is approximately 28 years of age. There are some studies that indicate that the duration is relatively brief and others that indicate it may last several years. This disparity appears to depend on the skeletal region examined(Reference Seeman and Hopper22), and is likely to become clearer as more longitudinal studies are published. There are data, however, to show that both Ca absorption and bone Ca deposition rates peak in girls shortly before menarche(Reference Langman23), which raises key questions as to the most effective timing of Ca and physical activity strategies for improving peak bone mass attainment.
Low Ca intakes during growth and late menarcheal age will affect peak bone mass, and consequently are likely to impact on fracture risk later in life. There are some studies that indicate that adolescent girls are less likely than boys to meet the current recommended dietary levels for Ca (although under-reporting may be a key confounding factor in these studies)(Reference Lorentzon, Mellstrom and Ohlsson24).
Clinical trials with Ca supplements in both children and teenagers have shown an overall positive effect of Ca on bone mass accrual. The effect has been shown to be between 1 and 6% per year in the total body and between 1 and 10% per year at different specific skeletal regions when compared with a placebo(Reference Nieves, Lanham-New, O'Neill, Morris, Skeleton and Sutcliffe25). However, the studies have only been of short duration (between 1 and 3 years). Pubertal stage is critical in relation to the effectiveness of the supplement. For example, a study of adolescent girls post menarche (<15·5 years of age) who had baseline low Ca intakes (<800 mg/d) has shown enhanced bone mineral acquisition in those girls given a 1000 mg/d supplement in comparison with girls given a placebo(Reference Rozen, Rennert, Dodiuk-Gad, Rennert, Ish-Shalom, Diab, Raz and Ish-Shalom26). The effect was observed to be especially evident in girls who were >2 years past the onset of menarche.
In a follow-up study to a 3·5-year Ca supplementation trial in ninety-six adolescent girls multivariate analysis has revealed that total-body bone mineral density (BMD) accrual over the 7 years (original and follow-up study) in the active-treatment cohort is attributable to Ca supplementation(Reference Bonjour, Ammann, Chevalley, Ferrari, Rizzoli, New and Bonjour27). A further 8-year follow-up study of Ca supplementation in girls who were prepubertal has suggested that prepubertal Ca intake may also have an effect on the timing of menarche(Reference Chevalley, Rizzoli, Hans, Ferrari and Bonjour28). The areal BMD gain between 7·9 and 16·4 years of age was found to be inversely related to menarcheal age at the axial and appendicular skeletal sites. A significant difference was observed between groups in relation to menarcheal age, with girls in the Ca-supplemented group starting menarche approximately 5 months sooner. On subgroup analysis based on menarcheal age it was found that the mean areal BMD gain from baseline in girls whose menarcheal age was below the median (13 years) remained significantly greater at all sites measured. In the late-menarche subgroup no lasting effect of Ca supplementation on BMD was observed. The authors suggest that Ca supplementation in prepubertal girls may accelerate the onset of pubertal maturation.
These data indicate the importance of Ca to peak bone mass development, although further research is clearly required to identify the exact mechanisms of an effect of increased Ca on pubertal timing(Reference Abrams, Copeland, Gunn, Gundberg, Klein and Ellis29).
Calcium and physical activity interactions: effects on peak bone mass attainment
Over 100 years ago, the German scientist Julius Wolff proposed the theory that is known as ‘Wolff's Law’: ‘bone accommodates the forces applied to it by altering its amount and distribution of mass’(Reference Wolff30). More recently, this concept has been refined to a general theory of bone mass regulation, known as the mechanostat model(Reference Frost31). It is well known that in the absence of weight-bearing exercise bone loss will occur at both axial and appendicular skeletal sites. A 3-year longitudinal investigation has shown consistently higher bone mineral content in young girls regularly undertaking weight-bearing exercise in comparison with healthy controls, despite late age of menarche or amenorrhoea (Fig. 5)(Reference Nurmi-Lawton, Baxter-Jones, Mirwald, Bishop, Taylor, Cooper and New32). Controlled intervention studies provide stronger evidence that effects of activity are causal and can be attained in less-active children who start to exercise (Fig. 6(Reference Fuchs, Bauer and Snow33)). Several such studies have examined the effects of exercise interventions on bone in children, with BMD or bone mineral content gains in the order of 1–5% greater in the exercise-intervention group than in the control groups(Reference Fuchs, Bauer and Snow33, Reference Valdimarsson, Linden, Johnell, Gardsell and Karlsson34). Structural adaptation also seems to occur, including increases in bone area, bone circumference and bone width.
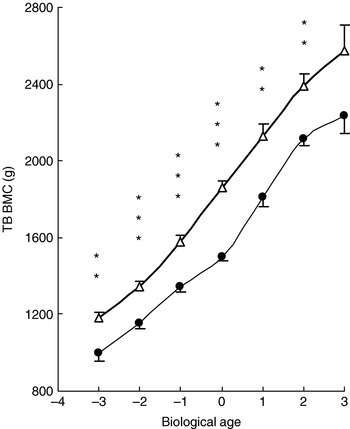
Fig. 5. Positive effect of impact loading exercise on peak bone mass attainment in young female gymnasts (△) in comparison with healthy controls (CON; ●) based on total-bone (TB) bone mineral content (BMC) by maturity (biological age 0 being the age of puberty and peak height velocity). Values are means with their standard errors represented by vertical bars. When adjusted for height and weight mean values were significantly different from those for CON: **P<0·01, *** P<0·001. (From Nurmi–Lawton et al.(Reference Nurmi-Lawton, Baxter-Jones, Mirwald, Bishop, Taylor, Cooper and New32); reproduced with permission.)

Fig. 6. An example of a jumping programme that improves hip and lumbar spine bone mass in prepubescent children. (From Fuchs et al.(Reference Fuchs, Bauer and Snow33); reproduced with permission.)
There are increasing data to suggest that both Ca and physical activity work synergistically on the skeleton(Reference Specker35). A randomised controlled trial in young children (3–5 years) has shown that exercise increases leg bone mineral content in children receiving a Ca supplement (1000 mg/d) but not in those receiving a placebo(Reference Specker and Binkley36). Cortical thickness and area of the tibia have also been found to increase in response to activity in children taking Ca, but to decline in those receiving a placebo. Similar results have also been reported for older children (7–11 years); greater leg bone mineral accrual was found with a combination of a Ca supplement (800 mg/d) and exercise compared with either treatment alone(Reference Bass, Naughton, Saxon, Iuliano-Burns, Daly, Briganti, Hume and Nowson37). Hence, adequate Ca intake may be essential for optimal skeletal response to exercise and exercise might enhance effects of Ca at weight-bearing sites(Reference Dawson-Hughes, Holick and Dawson-Hughes38).
Calcium and post-menopausal bone loss
There are now good data to show that Ca supplements are effective in reducing bone loss in women late post menopause (>5 years post menopause), particularly in those with low habitual Ca intake (<400 mg/d)(Reference Heaney39). A key study has shown that women late post menopause have a significant reduction in lumbar spine and femoral neck bone loss following supplementation with 500 mg Ca/d, with no such effect in women early post menopause (<5 years post menopause)(Reference Dawson-Hughes, Dallal, Krall, Sadowski, Sahyoun and Tannenbaum40) (Table 1). A review of over twenty studies has shown that Ca supplementation can decrease bone loss by approximately 1% per year(Reference Goulding, Grant, Lanham-New, O'Neill, Morris, Skeleton and Sutcliffe41) and a meta-analysis that included fifteen trials indicates that Ca supplementation at levels between 500 and 2000 mg/d reduces post-menopausal bone loss(Reference Shea, Wells and Cranney42); Ca changes were of the order of 1·66% at the lumbar spine and 1·64% at the hip. There are some data to suggest that the effect of Ca supplementation may be greater at skeletal sites with more cortical bone(Reference Ho, Chen, Woo and Lam43, Reference Suzuki, Davison and Chilibeck44). There are also data to suggest that Ca supplementation improves the efficacy of antiresorptive therapy on bone mass(Reference Nieves, Komar, Cosman and Lindsay45).
Table 1. Calcium supplementation and post-menopausal bone loss in healthy women who received placebo or either calcium citrate malate (CCM) or calcium carbonate (CC; 500 mg calcium/d) for 2 years (from Dawson-Hughes et al.(Reference Dawson-Hughes, Dallal, Krall, Sadowski, Sahyoun and Tannenbaum40); reproduced with permission)

BMD, bone mineral density.
Vitamin D nutrition: key concepts
Types of vitamin D
Vitamin D is the generic term for two molecules, i.e. ergocalciferol (vitamin D2) and cholecalciferol (vitamin D3). Ergocalciferol is derived by UV irradiation of ergosterol, which is found in fungi and plants(Reference Holick46). Cholecalciferol is formed from the effect of UV irradiation on the skin and the efficiency of cholecalciferol synthesis is dependent on the number of UVB photons that penetrate into the epidermis. As shown in Fig. 7, the action of sunlight on the skin converts 7-dehydrocholesterol to previtamin D, which is metabolised to vitamin D by a temperature-dependent isomerisation. Vitamin D is then transported via the general circulation to the liver, where the enzyme 25-hydroxylase converts it to 25-hydroxycholecalciferol (25 OHD). The kidney is the site for further conversion to 1,25-dihydroxycholecalciferol. 25 OHD is the best indicator of clinical status and is the key circulating vitamin D metabolite(47). 1,25-Dihydroxycholecalciferol is the active form of the vitamin, which is involved in Ca homeostasis, helping to maintain normal blood levels of Ca and P and promoting Ca absorption and bone mineralisation(Reference Calvo, Whiting and Barton48).

Fig. 7. Metabolism of vitamin D. 25(OH)D3, 25-hydroxycholecalciferol; 1,25(OH)2D3, 1,25-dihydroxycholcalciferol; 24,25(OH)2D3, 24,25-dihydroxycholcalciferol; 1,24,25(OH)3D3, 1,24,25-trihydroxycholcalciferol. (From Holick(54); reproduced with permission.)
Sources of vitamin D
It is believed that the main source of vitamin D is the UV in sunlight. However, whether UV exposure is sufficient to keep vitamin D levels optimal has recently been the subject of considerable debate(Reference Veith, Bischoff-Ferrari and Boucher49), particularly given the evidence of extensive hypovitaminosis D in the UK. Findings of the National Diet and Nutrition Surveys (4–18 years(Reference Finch, Doyle, Lowe, Bates, Prentice, Smithers and Clarke50), 19–64 years(Reference Gregory, Lowe, Bates, Prentice, Jackson, Smithers, Wenlock and Farron51) and ≥65 years(Reference Henderson, Gregory and Swan52)) indicate that vitamin D ‘deficiency’ (defined as a plasma 25 OHD level <25 nmol/l or 10 ng/ml) is a problem (e.g. 24% of men and 28% of women in the age-group 19–24 years have levels <25 nmol/l(Reference Gregory, Lowe, Bates, Prentice, Jackson, Smithers, Wenlock and Farron51)). More recently, data from the 1958 British birth cohort (n 7437) has shown extensive hypovitaminosis D in subjects at 45 years of age; 25 OHD levels <25, <40 and <75 nmol/l were found in 15·5, 46·6 and 87·1% of the population respectively, with lower levels in the north v. south(Reference Hypponen and Power53) (Fig. 8).

Fig. 8. Evidence of extensive hypovitaminosis D in the UK from the 1958 British Cohort (n 7437). 25-hydroxyvitamin D levels (nmol/l) of <25 (10 ng/ml), <40 (16 ng/ml) and <75 (30 ng/ml) were found in 15·5, 46·6 and 87·1% of the population respectively. Distribution in the population: (□), 5–9·9%; (), 10–19·9%; (
), 20–29·9%; (
), 30–39·9%; (
), 40–49·9%; (
), 50–59·9%; (■), 60–69·9%. (From Hyponnen & Powers(Reference Hypponen and Power53); reproduced with permission.)
Much of the UV in sunlight is absorbed by clouds, O3 and other forms of atmospheric pollution. With a reduced zenith angle of the sun and increased path length of sunlight through the atmosphere, the effective level of UV energy decreases north–south with distance from the seasonally-varying latitude at which the sun is directly overhead(Reference Holick54). Hence, in areas of northern latitude (such as the UK) there is no UV radiation of the appropriate wavelength (280 mm–310 mm) from the end of October to the end of March. For the remaining months of the year the main percentage of the effective UV radiation occurs between 11.00 hours and 15.00 hours(55).
A number of factors affect vitamin D production from UV exposure: (1) sunscreens absorb UVB radiation when applied to the skin; hence, there is marked reduction in the penetration of UVB photons into the epidermis and as a result production of pre-cholecalciferol is markedly reduced; (2) glass absorbs all UVB photons and hence exposure of the skin from sunlight that has passed through glass will not promote cholecalciferol synthesis in the skin; (3) clothing also absorbs UVB radiation and so no cholecalciferol is made in the skin covered by clothing; hence, women who cover up for cultural reasons are vitamin D deficient and this factor represents an area of research that requires urgent attention.
As shown in Table 2, there are few dietary sources of vitamin D. The major providers are fat spreads (which are fortified with vitamin D), fish, eggs, pastry products, fortified breakfast cereals and meat(Reference Lanham-New, Thompson, More, Brooke-Wavell, Hunking and Medici56). Recent changes in EU regulations have resulted in a number of cereal manufacturers removing the vitamin D fortification(Reference Cashman, Lanham-New, O'Neill, Morris, Skeleton and Sutcliffe57). Key Food Standards Agency-funded research on the interaction between diet and sunlight exposure on vitamin D status is currently underway and results will provide core information on vitamin D requirements(Reference Ashwell, Stone and Mathers58).
Table 2. Vitamin D content of foodsFootnote * (from Lanham-New et al.(Reference Lanham-New, Thompson, More, Brooke-Wavell, Hunking and Medici56); reproduced with permission)

* Portions are for adolescent boys and girls and adults and portions sizes (with the exception of items indicated) are from Food Standards Agency(94).
† Portion size and values taken from packaging information.
‡ Values (with the exception of items indicated) are from McCance & Widdowson(95).
Importance of vitamin D to bone
Vitamin D stimulates bone matrix formation and bone maturation. It also enhances osteoclastic activity and there are some data to suggest that it may influence differentiation of bone cell precursors(Reference Underwood and DeLuca59). Together with parathyroid hormone, it regulates Ca and P metabolism and promotes Ca absorption from the gut and kidney tubules(Reference Boland60). It has been shown that fractional Ca absorption increases with serum 25 OHD concentrations within the reference range, up to a level of 80 nmol/l, reaching a plateau above that level(Reference Heaney, Dowell and Hale61).
Vitamin D and peak bone mass development
There is growing evidence that mild vitamin D insufficiency can have a detrimental effect on bone mineral mass in adolescent females(Reference Outila, Kakkainen and Lamberg-Allardt62) and children(Reference Lehtonen-Veromaa, Mottonen, Nuotio, Irjala, Leino and Viikari63, Reference Cheng, Tylavsky and Kroger64). A 3-year prospective cohort study in 171 healthy Finnish girls aged 9–15 years comparing subjects with severe hypovitaminosis D (25 OHD <20 nmol/l) with those with normal vitamin D status (25 OHD ≥37·5 nmol/l) has found a difference of 4% in BMD accrual(Reference Cheng, Tylavsky and Kroger64). The adjusted change in lumbar spine BMD was shown to be 27% higher for subjects in the highest tertile of vitamin D intake compared with those in the lowest tertile. Interestingly, the findings were reported to be significant only for girls at the post-pubertal stage, with no differences for girls of less maturity (<12 years); a finding that has been reported previously(Reference El-Hajj Fuleihan, Veith, Burckhard, Heaney and Dawson-Hughes65). Low vitamin D status is a risk factor for attaining a reduced peak bone mass(Reference El-Hajj Fuleihan, Nabulsi, Choucair, Salamoun, Hajj Shahine, Kizirian and Tannous66).
In a key vitamin D-supplementation study on bone mineral accretion in 212 adolescent girls who were Ca replete (mean age 11·4 years) bone mineral augmentation at the femur was found to be 14·3 and 17·2% higher in the groups receiving the vitamin D supplementation (at either 5 μg/d or 10 μg/d) in comparison with the placebo group(Reference Viljakainen, Natri, Karkkainen, Huttunen, Palssa, Jakobsen, Cashman, Molgaard and Lamberg-Allardt67). The findings followed a dose–response relationship for those subjects who were Ca replete. A difference was also seen for lumbar spine bone density between the group supplemented at 10 μg/d level and the placebo group. Furthermore, vitamin D supplementation was found to significantly reduce bone resorption (as assessed by urinary deoxypyridinoline excretion). These findings were only observed to be significant when the data were analysed by the compliance-based method (which includes only participants who complied with instructions) as opposed to the intention-to-treat method (which includes all participants who entered the trial). Further research is required on the combined effect of Ca and vitamin D on maximising peak bone mass attainment, particularly in children accustomed to a low Ca intake.
Calcium and vitamin D in fracture prevention
Ca and vitamin D supplementation studies have been shown to reduce fracture rates in institutionalised elderly individuals(Reference Chapuy, Arlot, Duboeuf, Brun, Crouzet, Arnaud, Delmas and Meunier68). Women living in nursing homes in France were given a 1200 mg Ca supplement and 20 μg vitamin D daily for a period of 18 months. Both hip fracture (P=0·040) and non-vertebral fractures (P=0·015) were shown to be significantly reduced in those women on the active treatment in comparison with the placebo (Fig. 9).

Fig. 9. Cumulative probability of hip fracture (a) and other non-vertebral fractures (b) in a placebo group (□) compared with a group treated with a calcium (1·2 g/d; ●) and vitamin D (20 μg/d) supplement. Hip fracture (P=0·040) and non-vertebral fractures (P=0·015) were significantly reduced for the supplemented group compared with the placebo. (From Chapuy et al.(Reference Chapuy, Arlot, Duboeuf, Brun, Crouzet, Arnaud, Delmas and Meunier68); reproduced with permission.)
There are also data to suggest an effect of Ca and vitamin D on fracture prevention in free-living elderly populations. Elderly American men and women (mean age 71 years) given 500 mg Ca and 20 μg cholecalciferol daily were found to have a reduced total number of non-vertebral fractures(Reference Dawson-Hughes, Harris, Krall and Dallal69). In a factorial cluster-randomised pragmatic intervention study of 9605 community-dwelling residents living in a northern European region (Denmark) a daily supplement of CaCO3 (1000 mg) and vitamin D (10 μg) over a period of 3 years was reported to result in a 16% reduction in fracture incidence rate (relative risk 0·84 (95% CI 0·72, 0·98) in treated subjects compared with those subjects who were offered no supplement but participated in an environmental and health programme(Reference Larsen, Mosekilde and Foldspang70).
Vitamin D supplementation alone is not effective in preventing fractures in the elderly if the dosage is limited. For example, a supplementation trial undertaken in Norway using cod liver oil that provided 10 μg vitamin D/d was not found to prevent osteoporotic fractures in 1144 nursing home residents(Reference Meyer, Smedshaug, Kvaavik, Falch, Tverdal and Pedersen71). It is important to note that a recent meta-analysis indicates that 17·5–20 μg/d reduces the risk of hip and any non-vertebral fractures in ambulatory or institutionalised elderly individuals, but 10 μg/d is not effective(Reference Bischoff-Ferrari, Willett, Wong, Giovannucci, Dietrich and Dawson-Hughes72).
A recently published study examining the effect of three injections of 7500 μg cholecalciferol per year has found no reduction in fracture risk in 9000 healthy ambulatory elderly men and women and, if anything, has shown that the vitamin D supplementation increased the risk of fracture (P<0·06)(Reference Smith, Anderson, Raphael, Maslin, Crozier and Cooper73). This finding contrasts with the results of a study of elderly British men and women aged 65–85 years in which it was shown that supplementation with 2500 μg cholecalciferol orally every fourth month for 5 years reduces the number of fractures of the hip, wrist, forearm and spine by 22% compared with placebo(Reference Trivedi, Doll and Khaw74).
Two key UK studies have not demonstrated a positive effect of Ca and vitamin D supplementation on fracture prevention in the free-living elderly population(Reference Porthouse, Cockrayne and King75, Reference Grant, Avenell and Campbell76). In a pragmatic open randomised trial conducted with 3314 women aged ≥70 years who had risk factors for hip fracture Ca (1000 mg/d) and cholecalciferol (20 μg/d) supplementation was not found to reduce the risk of fracture(Reference Porthouse, Cockrayne and King75). No evidence of an effect on falls at either 6 months (OR 0·99 (95% CI 0·81, 1·20) or 1 year (OR 0·98 (95% CI 0·79, 1·20)) were seen. Adherence rate (60%), however, was low. In the MRC-Record trial involving 5292 men and women aged ≥70 years who were mobile before developing a low-trauma fracture, 1000 mg Ca and 20 μg vitamin D, either alone or in combination, was not found to significantly reduce the incidence of new low-trauma fractures after 2 years(Reference Grant, Avenell and Campbell76). It is important to note, however, that compliance was again low in this study (possibly as low as 45% when non-responders to the questionnaire were included) and only a small number of subjects were measured for vitamin D status(Reference Sambrook77).
The 2005 Cochrane review has reported a reduced risk for hip fracture (relative risk 0·81 (95% CI 0·68, 0·96) for seven trials) and non-vertebral fractures (relative risk 0·87 (95% CI 0·78, 0·97) for seven trials) but no significant effect on vertebral fractures(Reference Avenell, Gillespie, Gillespie and O'Connell78). The authors conclude that the effect may be restricted to those living in institutional care. The most recent meta-analysis of the effect of Ca and vitamin D supplements on fracture prevention suggests an overall positive effect(Reference Tang, Eslick, Nowson, Smith and Bensoussan79).
Vitamin K nutrition and bone
Vitamin K (‘koagulation vitamin’) was first described as a dietary-derived coagulation factor(Reference Dam80); it was noted that a bleeding disorder in chickens was corrected by feeding a variety of vitamin K-rich diets. The fat-soluble vitamin was finally isolated in 1939(Reference Bolton-Smith, James and Garrow81). Vitamin K refers to a family of compounds with a common chemical structure, 2-methyl-1,4 napthoquinone, as shown in Fig. 10. Phylloquinone (vitamin K1) is present in foods of plant origin. Bacterial forms of vitamin K, referred to as the menaquinones (vitamin K2) differ in structure from phylloquinone(Reference Shearer, Bach and Kohlmeier82).

Fig. 10. Different forms of vitamin K. Vitamin K exists in two forms, phylloquinone and menaquinones.
Vitamin K has an important function for the skeleton as it acts as a cofactor in the post-translational carboxylation of several bone proteins, with osteocalcin being the most abundant(Reference Booth, New and Bonjour83). Deficiency of vitamin K results in the synthesis of under-carboxylated osteocalcin(Reference Szulc, Chapuy, Meunier and Delmas84). There are data to show that low serum concentrations of either phylloquinone or under-carboxylated osteocalcin are associated with low BMD and increased risk for osteoporotic fracture(Reference Binkley, Krueger, Engelke, Foley and Suttie85).
A number of recently published studies suggest a key role for vitamin K nutrition for optimisation of bone health(Reference Weber86). Phylloquinone supplementation (at a dose of 200 μg/d, which would be obtainable in the diet from a 50 g portion of green leafy vegetables) in combination with Ca (1000 mg) and vitamin D (10 μg) was investigated over a 2-year period in a randomised placebo-controlled study (Reference Bolton-Smith, McMurdo, Paterson, Mole, Harvey, Fenton, Prynne, Mishra and Shearer87). Subjects who took the combined phylloquinone and vitamin D plus Ca supplement were found to show a significant increase in ultra-distal BMD and bone mineral content. The effect of phylloquinone (at a higher dose of 1 mg/d) on BMD has been studied in 155 healthy post-menopausal women aged 50–60 years of age over 36 months(Reference Braam, Knapen, Geusens, Brouns, Hamulyák, Gerichhausen and Vermeer88). The study examined the potential complementary effect on post-menopausal bone loss of phylloquinone, vitamin D (8 μg/d) and a mineral supplement (including 150 mg Mg/d, 500 mg Ca/d and 10 mg Zn/d). The results indicate a positive effect of the phylloquinone supplement on bone loss at the femoral neck, leading to a 35% reduction in bone loss when compared with placebo after a period of 36 months.
Menaquinone supplementation has also been shown to effectively improve markers of bone health, although it is important to note that the doses of menaquinones used could not be achieved via the diet(Reference Knapen, Schurgers and Vermeer89). A recent meta-analysis examining the effect of menaquionone supplementation on vertebral and hip fracture shows a convincing effect of pharmacological doses of menaquinones on fracture rate (Fig. 11(a and b))(Reference Cockrayne, Adamson, Lanham-New, Shearer, Gilbody and Torgerson90, Reference Shearer, Cockrayne, Adamson, Lanham-New, Gilbody and Torgerson91). The role of vitamin K on prevention (and possibly treatment) of osteoporosis is an exciting area that requires further research.

Fig. 11. Meta-analysis of the effect of menaquinones on (a) vertebral fracture prevention and (b) hip fracture prevention. (From Cockrayne et al.(Reference Cockrayne, Adamson, Lanham-New, Shearer, Gilbody and Torgerson90); reproduced with permission.)
Concluding remarks
It is widely recognised that there are genetic, environmental, lifestyle and dietary determinants of risk of osteoporotic fracture, as well as interactions between them. There is good evidence to show that the effects of nutrition on the skeleton are powerful and wide-ranging. Given that by the year 2030 one in four of the adult population will be elderly, it is vital that special attention is given to nutritional strategies for the optimisation of bone health throughout the life cycle, including the role of diet in reducing falls(Reference Jarvinen, Sievanen, Khan, Heinonen and Kannus92). Ca and vitamin D are clearly key nutrients for optimal bone health. At all costs, suboptimal intakes or status must be prevented and dietary vitamin D fortification should be considered for vulnerable groups such as older adults, post-menopausal women, adolescent females and amenorrhoeic women. Ca and vitamin D have been shown to be effective strategies for fracture prevention in the elderly, particularly for those populations in which vitamin D insufficiency is rife. Ca supplementation alone does not appear to be effective in reducing fracture(Reference Bischoff-Ferrari, Dawson-Hughes and Baron93). In recent years, evidence has emerged for a role for vitamin K in bone health. Further data are urgently required to enable a fuller understanding of the complex interaction between dietary factors and bone health.
Acknowledgement
The author has the following conflicts of interest to declare: S.L-N. is co-director of D3TEX Ltd, a company which has a patent pending on materials which allow sunlight through. S.L-N. has also acted as a consultant to the following companies with respect to Vitamin D and Vitamin K: Dairy Crest Yoplait, CH Hansen, Springfields Ltd. The views expressed are the author's alone.