According to a Packaged Facts™ report( 1 ), the number of consumers who are seeking out high-n-3 products has increased dramatically over the past few years. In the US market, the percentage of adults who take fish oil supplements has jumped from 8 % in 2006 to 17 % in 2011; global consumer spending on n-3 products (excluding fish) was about $13 × 109 in 2011 and will reach $34·7 × 109 by 2016. Asia–Pacific is accounting for 43 % of sales.
According to a Transparency Market Research™ report( 2 ), the demand for fish oil was 1035 kilotonnes in 2011 and is expected to reach 1130 kilotonnes in 2018. Aquaculture (salmon and trout) accounted for over 70 % of the consumption of oil in 2011 primarily in Chile and Peru. However, direct human consumption of fish oil has been increasing over the past 5 years, due to marketer's influence and due to an increase in awareness of health benefits of long-chain (LC) n-3 PUFA, such as prevention of type-2 diabetes (Asian, Eskimos), prevention of insulin-resistance( Reference Delarue, LeFoll and Corporeau 3 , Reference Zhang, Picard-Deland and Marette 4 ), decrease in plasma TAG( Reference Mozaffarian and Wu 5 ), anti-inflammatory effect( Reference Calder 6 ), the defect of brain and retina development (only if deficiency in LC n-3 PUFA), primary cardiovascular prevention( Reference Mozaffarian and Wu 5 ), anti-stress and anti-adrenergic effect( Reference Delarue, Matzinger and Binnert 7 , Reference Delarue, Guillodo and Guillerm 8 ) and decrease in liver steatosis( Reference Di Minno, Russolillo and Lupoli 9 ). Because of these health benefits and of some deleterious effects of deficiency, many national and international recommendations have been proposed about the optimal intake of LC n-3 PUFA for the general population. They vary considerably depending on the country and on the type of illness to prevent and/or to treat (Table 1) (see website: www.omega3dressings.com/Dietary_Recommendation.html).
Table 1. Selected suggested long-chain n-3 (EPA+DHA) intakes for adults available from various agencies and bodies (from( 10 ))
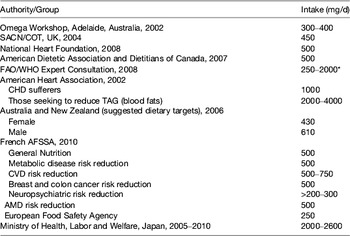
SACN/COT, Scientific Advisory Committee on Nutrition/Committee on Toxicity of Chemicals in Food, Consumer Products and the Environment; AFSSA, Agence française de sécurité sanitaire des aliments; AMD, age-related macular degeneration.
* For secondary prevention of CHD.
A calculation can be easily made: choosing a daily 500 mg requirement of EPA+DHA, a global population reaching 8 × 109 by 2025, assuming fish contains 2–5 mg/100 g oil and an LC n-3 PUFA content of 450 mg/150 g flesh, the current global fish harvest (93 metric tonnes per annum) will not supply this requirement. If the UK recommendation of intake of two meals including 140 g fish per week were followed( 11 ), then annual per capita consumption would have to rise to 23·3 kg. This translates into an additional production of 40 million tonnes for 2008, rising to 82 million tonnes in 2050. Per capita fish consumption per year has increased hugely from the 1960s: 9·9 kg in the 1960s, 11·5 kg in the 1970s, 12·6 kg in the 1980s, 14·4 kg in the 1990s, 17·0 kg in 2007 (China 26·7 kg; 14·6 kg without China) and 17·1 kg in 2008. Fish and fish trimmings caught for fishmeal and fish oil productions are reported in Tables 2 and 3.
Table 2. Species of fish and countries where they are caught for fishmeal and oil production (from( 12 ))
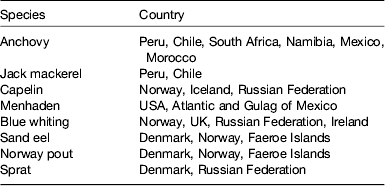
Table 3. Fish trimmings used for fishmeal and oil production (from( 12 )).
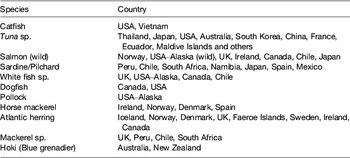
Myers & Worm( Reference Myers and Worm 13 ) have published in Nature a frequently cited paper where they conclude that ‘Industrialised fisheries typically reduced community biomass by 80 % within 15 years of exploitation. We estimate that large predatory fish biomass today is only about 10 % of pre-industrial levels. We conclude that some species compensation was evident, but often reversed within a decade or less, probably because of changes in targeting or bycatch.’
For all these reasons, concerns have been reached about the risk of depletion of oily fish communities (the most used source of LC n-3 PUFA). Thus, over the past few years, alternative sources of LC n-3 PUFA to oily fish have been developed.
Alternative sources of long-chain n-3 PUFA
In order to be acceptable for use in human subjects, alternative sources and final products must be safe, green and healthy. The first alternative to oily fish capture is aquaculture. However, aquaculture uses manly fish oil and fishmeal( Reference Petrie, Shrestha and Zhou 14 ). Strobel et al.( Reference Strobel, Jahreis and Kuhnt 15 ) reported that, in Germany, fat content in fillets from cultured salmon was 12·3 v. 2·07 wt % in the wild counterpart, which resulted in 4-fold greater absolute amount in total LC n-3 PUFA (2·40 (sd 0·78) v. 0·53 (sd 0·21) % of fresh weight). Nichols et al. ( Reference Nichols, Petrie and Singh 16 ) reported that in Australia the average content in LC n-3 PUFA of wild fish was 350 mg/150 g wet weight as compared with a range of 1200–3700 mg/150 g in farmed fish. This requires fishmeal and fish oil.
In order to increase sustainability of sources of LC n-3 PUFA, fish oil and fishmeal use can be limited to the last weeks of growth (finishing diets). However, other sources should be used or developed for aquaculture or as a direct source of LC n-3 PUFA as food ingredient or as pharmaceutical. These alternatives are: (a) terrestrial or GM plant-based lipids; (b) single-cell oil (SCO); (c) krill.
Terrestrial plant based-lipids and transgenic oil seeds
Terrestrial plants, such as canola, sunflower, soya, flax, olive, palm or linseed contain linoleic acid (18:2n-6), oleic acid (18:1n-9) or α-linolenic acid (18:3n-3) have been used to feed farmed fish, but their flesh content in LC n-3 PUFA was lower than that of their wild counterparts. Consequently, they are now used only during the initial phase of growth and fish oils or fishmeal rich in LC n-3 PUFA are used as finishing diet over the final 10 weeks.
Stearidonic acid (SDA, 18:4n-3) is more efficiently converted to EPA than 18:3n-3 because it does not require the first rate-limiting step: ∆6-desaturase. Foods rich in SDA are hemp oil, seeds from Boraginacea family of plants including borage, blackcurrant seed oil (2–4 % SDA) and Echium plantagineum seed oil (echium oil, 12–14 % SDA)( Reference Walker, Jebb and Calder 17 , Reference Surette 18 ). A new plant oil extracted from the seeds of Buglossoides arvensis (Ahiflower™ oil) is a rich source of SDA (20 % SDA). Lemke et al. ( Reference Lemke, Maki and Hughes 19 ) compared in healthy subjects either 1·5 g/d high-oleic sunflower ethyl ester oil capsules plus foods containing 7 g/d high-oleic sunflower oil (control) or 1·5 g/d EPA oil ethyl ester capsules plus foods containing 7 g/d high-oleic sunflower oil (EPA), or 1·5 g/d high-oleic sunflower ethyl ester oil capsules plus foods containing 7 g/d SDA soyabean oil for 12 weeks. The content in EPA of erythrocyte membranes was 0·50 ± 0·03, 2·17 ± 0·21 and 0·85 ± 0·05 % for control, EPA and SDA, respectively. This demonstrates the ability of SDA to be converted into EPA in human subjects but the content in EPA was far below this obtained following EPA oil supplement.
A SDA-rich oil (E. plantagineum L., Boraginaceae; 14 % SDA), given over 6 weeks to Atlantic salmon parr (freshwater phase) led to a similar accumulation of LC n-3 PUFA to fish oil( Reference Miller, Nichols and Carter 20 ). As a comparison, canola oil rich in 18:3n-3 did not lead to accumulation of EPA and DHA. Thus, SDA-rich aquafeeds might replace fish oil in freshwater aquaculture. However, this occurred over the period before smolting, which has been shown to coincide with a period of peak of LC n-3 PUFA production. Conversely, when given to seawater Atlantic salmon, SDA-rich oil did not produce the same level of EPA and DHA as a fish oil diet( Reference Miller, Bridle and Nichols 21 ). Thus, further assessment of SDA-rich oil is needed. Furthermore, it is currently more expensive than fish oil.
A mixed vegetable oil (mix of rapeseed, palm and degummed linseed oils in a 3·7:2:1 ratio) resulted after the finishing diet period, in LC n-3 PUFA flesh content similar to that obtained with a 100 % fish oil fed salmon without any difference in growth, mortality and sensory characteristics( Reference Torstensen, Bell and Rosenlund 22 ).
Transgenic plants have been created to produce LC n-3 PUFA owing to the characterisation of LC PUFA biosynthetic pathways and the availability of many genes encoding these pathways (see details in Venegas-Calerón et al ( Reference Venegas-Calerón, Sayanova and Napier 23 )). Briefly, the (aerobic) ∆6-pathway is widely spread in eukaryotes; the alternative pathway in algae and protists is the ∆8-pathway (18:3n-3 is first elongated to 20:3∆11,14,17 eicosatrienoic acid followed by a ∆8-desaturation). A third (anaerobic) pathway (present in a few lower marine organisms) requires neither desaturases nor elongases, but a processive polyketide synthase-like reaction from malonyl-CoA (Fig. 1).
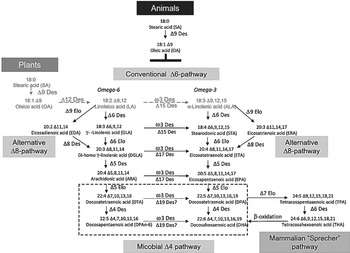
Fig. 1. Aerobic very long chain PUFA biosynthetic pathways. The various routes for synthesis of arachidonic acid, EPA and DHA are shown, as mediated by the consecutive action of desaturases and elongases. The predominant Δ6-pathway is shown, as is the alternative Δ8-pathway. Two routes for DHA synthesis are shown, microbial Δ4-pathway and mammalian ‘Sprecher’ pathway. Des, desaturase; Elo, elongase (from ( Reference Venegas-Calerón, Sayanova and Napier 23 )).
These transgenic plants can synthesise EPA and DHA, but most of them also accumulate intermediates of the n-6 pathway. Recently, Petrie et al.( Reference Petrie, Shrestha and Zhou 14 ) described the transgenic DHA production by Arabidopsis thaliana with a DHA content as high as 15 % (higher than the 12 % usually found in classical fish oils) and a high n-3:n-6 ratio (8:1 to 16:1 depending on the native 18:3n-3 and 18:2n-6 were included or not). However, the EPA content of this transgenic seed was only 1·8 % (18 % in classical fish oils). The same authors( Reference Petrie, Shrestha and Zhou 14 ) calculated that the application of this technology in crop species would result in 1 ha of a Brassica napus (canola) crop containing 12 % DHA in seed oil to produce as much DHA as approximately 10 000 fish. Ruiz-Lopez et al.( Reference Ruiz-Lopez, Haslam and Napier 24 ) have also developed a transgenic approach in the seed oil of the crop Camelina sativa. They obtained some seeds containing EPA levels up to 31 % (mean 24 %) and other seeds accumulating up to 12 % EPA and 14 % DHA (mean 11 % EPA and 8 % DHA).
In Europe, GM organisms are not well accepted by the consumers. Thus, a more acceptable alternative could be to feed farmed fish with these transgenic plants to enhance EPA and DHA generation instead of fishmeal or fish oil.
Single-cell oil
SCO, extracted from micro-organisms grown under heterotrophic conditions, can also be rich in LC n-3 oils. Examples of micro-organisms are microalgae( Reference Hong, Rairakhwada and Seo 25 – Reference Ryckebosch, Bruneel and Muylaert 27 ) including thraustochytrids( Reference Zhou, Lu and Li 28 , Reference Qu, Ji and Ren 29 ) and Escherichia coli ( Reference Amiri-Jami and Griffiths 30 ).
Microalgae
The ability of microalgae to be an alternative source of LC n-3PUFA has been recently reviewed( Reference Araujo, Zhu and Breivik 31 ). In the marine food chain, microalgae (phytoplankton) are rich in EPA and DHA. They are consumed by zooplankton itself consumed by fish, so that the content in EPA and DHA is particularly high in marine oily fish. Microalgae offer a non-polluted resource for LC n-3 PUFA production, but for human consumption, microalgae and their products must be non-pathogenic and non-toxic, genetically stable, and present high growth and product formation rates( Reference Ward and Singh 32 ). Ideally, the selected strains should be able to accumulate high amounts of TAG rich in EPA and/or DHA (up to 50 % biomass dry weight, including 30–70 % DHA).
LC n-3 PUFA biosynthesis from α-linolenic acid by the elongase–desaturase pathway is common in microalgae. In some species, biosynthesis of EPA involves elongation of α-linolenic acid, followed by a Δ8 and Δ5 desaturation; DHA is obtained through EPA elongation into docosapentaenoic acid and subsequent desaturation by Δ4 desaturase, or through the anaerobic polyketide synthase pathway, as it has been suggested for thraustochytrids( Reference Venegas-Calerón, Sayanova and Napier 23 ).
Photoautotrophic microalgae
Photoautotrophic LC n-3 PUFA producing microalgae are mainly marine species. The EPA and DHA content and the EPA:DHA ratio vary from species to species (Tables 4–6). Large variation in EPA and DHA content within one species is due to the culture conditions, the growth phase of the microalgae at the time of harvest and the extraction technique used to determine the lipid content and fatty acid profile. Photoautotrophic technologies for marine microalgae cultures in open pond systems cultivation can utilise non-arable lands and water resources considered unsuitable for agriculture. These processes also have a more favourable net energy ratio, relative to heterotrophic systems, but specific growth rates in these systems are relatively low, harvesting may be costly, and the risks of salinity fluctuation and contamination can lead to variable quality and quantity of the final product. Photobioreactors allow better growth efficacy and productivity, better control over environmental parameters, significant reduction of contamination risks, and allow higher biomass concentrations, but high volume to surface ratio is currently limited due to light penetration restrictions. Lastly, they are very expensive. Thus, the use of these systems is currently justified only for production of high-value products.
Table 4. Examples of marine microalgae species characterised by EPA production (adapted from( Reference Martins, Custódio and Barreira 26 ))
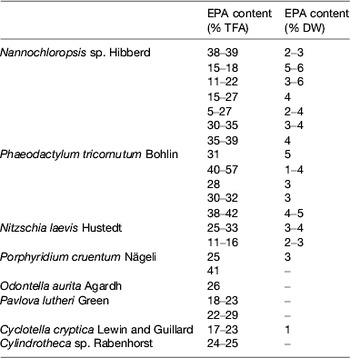
TFA, total fatty acids; DW, dry weight.
Table 5. Examples of marine microalgae species characterised by DHA production (adapted from( Reference Martins, Custódio and Barreira 26 ))
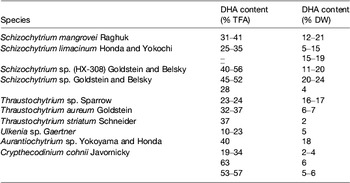
TFA, total fatty acids; DW, dry weight.
Table 6. DHA and EPA content in various fish species (from ( Reference Lee, O'Keefe and Lavie 33 ))
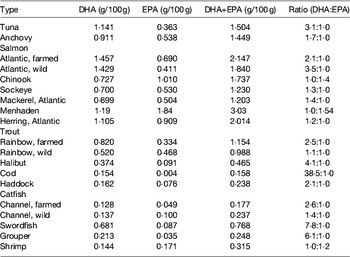
Photosynthetic microalgae tend to produce higher levels of EPA than heterotrophs. Nannochloropsis Hibberd, Phaeodactylum Bohlin, Nitzschia Hassall and Porphyridium Nägeli can present a high percentage of EPA in total fatty acids, but its total amount in the biomass is low (Table 4). Photoautotrophic species contain lipids involved in the photosynthetic metabolism and, unlike various DHA-rich microalgae, the fatty acid profiles of EPA producers usually show other LC-PUFA, such as DHA and/or arachidonic acid (Table 5).
Phototrophic species, such as the eustigmatophyte Nannochloropsis, have long been used by the aquaculture industry with the aim to supply larval fish with n-3 LC-PUFA through the enrichment of live feeds (e.g. rotifers; for review see Martins et al. ( Reference Martins, Custódio and Barreira 26 )). Gog et al.( Reference Gog, Senila and Roman 34 ) described a Nannochloropsis oculata with a fatty acid profile containing 49 % EPA, while most commonly Nannochloropsis strains show lower levels, in the range of 11–39 %. Although Nannochloropsis accumulates TAG in response to environmental stressors, EPA deposition may be low within this lipid class, which is instead associated with shorter fatty acids. Deposition of EPA in Nannochloropsis seems to be favoured by low salinity, whereas total lipids appear to increase in higher salinity waters. The cultivation of Nannochloropsis sp. in ultra-dense cultures, with valuable EPA production, using frequent replacement of nutrient medium, has been described. In fact, the genus Nannochloropsis is currently the source of marketed oils due to its potential to produce high EPA lipids with very low DHA and arachidonic acid content, which is advantageous for the manufacture of dietary supplements (Table 7).
Table 7. Levels of EPA and/or DHA in commercially available oils derived from marine microalgae cultures (adapted from( Reference Martins, Custódio and Barreira 26 ))
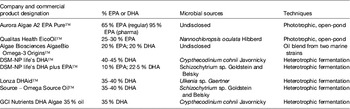
Heterotrophic microalgae
Heterotrophic microalgae produce EPA and/or DHA mainly as TAG and phospholipids. Heterotrophic DHA, predominantly obtained with Schizochytrium species, is already commercially available for human consumption, animal feed and aquaculture. Heterotrophic cultivation without light, using inexpensive mineral medium supplemented with a carbon source is feasible provided fermentation and culture conditions are optimised to obtain the highest content in EPA and/or DHA of each strain. Overall, application of photosynthetic species for the commercial production of oils requires advances in physiological and genetic engineering studies to enhance growth performances and lipid deposition, optimisation of fatty acid composition, biotechnological improvements regarding light capture and contamination, and lowering of costs involved in biomass production and harvesting( Reference Lee, O'Keefe and Lavie 33 ). Several marine heterotrophic microalgae are considered as good DHA sources (Table 5). These species represent the most preeminent alternative industrial sources of oils rich in DHA (Table 7), with approved use in human foods, especially for application in infant formulas, since they are considered to be non-pathogenic and non-toxigenic. Ward and Singh( Reference Ward and Singh 32 ) reported that some Schizochytrium strains might produce levels as high as 94 % DHA of total n-3 fatty acids. Schizochytrium sp. possess a number of traits that are advantageous for the industry, including high lipid content, elevated DHA production and presentation in the TAG form, as well as good growth in culture under elevated cell concentrations. In Schizochytrium strains, DHA may reach 30–35 % of total fatty acids mainly in TAG fraction (see Martins et al. ( Reference Martins, Custódio and Barreira 26 ) for details).
Thraustochytrids have also been used in the grow-out phase of Atlantic salmon, either as whole-cell biomass or the extracted SCO. Complete replacement of fish oil by thraustochytrid oil in the diets resulted in a comparable growth and health associated with a higher concentration of DHA in the flesh. The large quantity of oil required throughout the life history of salmonids and marine fish makes such the use of SCO cost-prohibitive as compared to LC n-3 PUFA obtained from plants. A potentially cost-effective approach is to use SCO in diets during the final 6–12 weeks of fish growth. A reduction of production costs of SCO is awaited because of the increasing interest in the industrial biofuels production from microalgae( Reference Lum, Kim and Lei 35 ).
Transgenic microalgae
Metabolic engineering of microalgae is, as in terrestrial plants, a way to enhance LC n-3 PUFA( Reference Hamilton, Haslam and Napier 36 , Reference Mühlroth, Li and Røkke 37 ). Phaeodactylum tricornutum is a unicellular diatom, which accumulates EPA (up to ∼35 %), but only trace levels of DHA. Hamilton et al. ( Reference Hamilton, Haslam and Napier 36 ) have overexpressed, in P. tricornutum, the Δ6-desaturase and Δ5-elongase, which significantly increased the DHA levels.
Krill
Antarctic krill (Euphausia superba) are the small, bug-eyed shrimp-like crustaceans that are the central diet for whales, penguins, seals and seabirds. Krill contain LC n-3 PUFA mainly as phospholipids( Reference Winther, Hoem and Berge 38 ) but also as TAG( Reference Araujo, Zhu and Breivik 31 ). E. superba contains the highest concentration of EPA and DHA compared to other krill species( Reference Phleger, Nelson and Mooney 39 ). The potential health benefits( Reference Ulven, Kirkhus and Lamglait 40 ) from these attributes are being marketed in various forms of krill oil products. Krill is considered as the single largest ‘underutilised’ commercial marine resource remaining. However, as factory ships are deployed and climate is changing, krill and Antarctic food web that depend on it could be threatened. The Southern Ocean krill biomass is estimated at up to 700 million metric tonnes( Reference Miller, Nichols and Carter 41 ). The annual krill harvest is still well within the very strict limits set by the Convention on the Conservation of Antarctic Marine Living Resources (twenty-four member countries and the EU), which regulates fishing in the Southern Ocean (www.ccamlr.org). The total annual catch level of the current Convention on the Conservation of Antarctic Marine Living Resources is 5 610 000 tonnes/year on sector 48 and 3 085 000 tonnes on sector 58. There is also a precautionary limit of 620 000 tonnes limit in area 48 (the so-called ‘trigger level’) and 452 000 for area 58·4·2. Once this limit is achieved, Convention on the Conservation of Antarctic Marine Living Resources might close the krill fishery until a procedure for the division of the overall catch limit into smaller management units.
As written by Naylor et al. ( Reference Naylor, Hardy and Bureau 42 ) ‘Krill is at the base of the Southern Ocean food web and is also particularly sensitive to environmental variables, including climate change. In some regions, considerable overlap exists between the krill fishery and the foraging ranges of land-based predators, such as penguins, which cannot move readily to new feeding areas. Local krill significantly reduce the food resources of other predators, such as seals and whales. Unfortunately, existing data on krill abundance and population variables are not sufficient to establish precautionary management of the krill fishery and its effect on the Antarctic ecosystem. Considerable care will thus be needed in setting local catch limits for krill harvest to protect key predators and other animals in the Southern Ocean ecosystem, and the Commission for the Conservation of Antarctic Marine Living Resources is trying to develop data-driven procedures to achieve this.’
Conclusion and perspectives
The ratio of wild to farmed fish has hugely decreased over the past decade to fall below one. This could be good news for sustainability of natural resources. However, aquaculture is now the main user of fishmeal and fish oil. Progress in aquaculture has led to the conclusion that the use of fishmeal rich in LC n-3 PUFA could be used only as a finishing diet over the final 10 weeks of fish growth without altering growth, taste or content in LC n-3 PUFA as compared to wild fish counterparts. Efforts have been made to develop alternative sources of LC n-3 PUFA. Crops rich in SDA have a greater interest on their own in human subjects or given to animals. However, cost is very high. Genetic engineering has increased the ability of terrestrial plants to produce more SDA or EPA and/or DHA, but they are poorly generally accepted by the consumers. A more acceptable way (with some psychological limits anyway) could be their use as feed in animals secondarily consumed by human subjects. SCO rich in LC n-3 PUFA show great promise for production of oils and for reducing dependence on wild fisheries. Their high cost of production makes them infeasible for feeding of farmed fish. Their ability to produce biofuels should help to decrease the costs in the future. Metabolic engineering of SCO (microalgae, E. coli) is also a way to generate LC n-3 PUFA. Do not forget that human insulin was for many years produced from E. coli strains. The better delineation of the real daily needs of LC n-3 PUFA, as well as their real health benefits and the specific effect of EPA v. DHA will bring a real boost in the production of high-quality high-grade LC n-3 supplements or of enriched foods of good nutritional value. The choice of the best source of LC n-3 PUFA should be determined by price, availability, formulation, taste and sustainability.
Acknowledgements
J. Delarue thanks la Région Bretagne for its support of his research.
Conflicts of interest
None.
Financial support
This research received no specific grant from any funding agency in the public, commercial or not-for-profit sectors.
Authorship
J. D. wrote the present paper; N. G. performed the literature search, gathered the articles, reviewed the manuscript and made the necessary corrections.